DOI:
10.1039/C3RA46642F
(Paper)
RSC Adv., 2014,
4, 8439-8445
Antifungal properties of nanosized ZnS particles synthesised by sonochemical precipitation
Received
13th November 2013
, Accepted 2nd December 2013
First published on 2nd December 2013
Abstract
Zinc sulphide (ZnS) nanoparticles, synthesised by a sonochemical route employing zinc chloride and sodium sulphide, displayed significant antifungal properties against the pathogenic yeast Candida albicans (MTCC 227) at a minimum fungicidal concentration of 300 μg ml−1. The antifungal properties of zinc sulphide particles is attributed to the generation of reactive oxygen species due to the interaction of the nanoparticles with water. Additionally, the presence of Zn in the zone of inhibition and the absence of antifungal effects for large micron sized particles of ZnS suggest a size induced effect leading to perturbation of fungal cell membranes, resulting in growth inhibition.
1. Introduction
Control of microbial growth has become increasingly difficult owing to the resistance offered by microbes against conventional antimicrobial agents. The use of nanoparticles such as TiO2, ZnO, Ag etc.1–10 for antimicrobial activity has been successfully demonstrated in recent times. Nanoparticles of ZnO and silver10–15 were developed as novel antibacterial agents and have been prepared by simple and cost effective techniques, such as chemical precipitation, with good control over particle sizes, surface area and stoichiometry.
A host of mechanisms have been postulated to explain the antibacterial effect of nanosized particles. The generation of reactive oxygen species,8,10,16–18 primarily through photo-induced mechanisms, is a prominent cause for the antimicrobial activity in the nanosized particles of titania and ZnO. The effect of particle size on the viability of such microbes has also been addressed and generally the antimicrobial properties increase with decreasing particle size.19–21 An efflux mechanism leading to the release of ions is also observed in certain cases.11,22 Internalisation of nanoparticles below a threshold size and the presence of certain organic species like diethylene glycol in the medium have also been reported to be effective for antimicrobial action.23 While the majority of work has been carried out on metal oxide nanoparticles, recent works report the use of zinc sulphide nanoparticles for antibacterial action.24,25
Bulk ZnS particles possess a band gap of 3.6 eV, while nanosized particles derived through chemical precipitation techniques possess band gap values greater than 3.6 eV due to a quantum confinement effect. However, doping with metallic ions like Ag, Ni, Mn etc. shifts the band gap of ZnS particles enabling them to be visible light active.26–28 This is primarily ascribed to the presence of mid-energy levels induced by the defects in the crystal. Thus the addition of Ag on microwave synthesised ZnS particles, leads to an increase in the photocatalytic activity under indoor light.26 High surface area nanoporous ZnS nanoparticles in the form of uniform spheres displayed effective photocatalysis in the degradation of eosin B under visible light.29 Ni doped nanostructured ZnS photocatalysts, prepared by ultrasonic spray pyrolysis, were successfully employed for hydrogen production under visible light irradiation.27 Studies on the photocatalysis of ZnS particles have prompted researchers to explore the antimicrobial activity of such particles through photoactive mechanisms. The relative ease with which ZnS particles are prepared makes them a commercially viable candidate material for antimicrobial applications.24,25
Pillai and co-workers recently demonstrated the antibacterial activity of nanocrystalline ZnS, through the degradation of Staphylococcus aureus and Escherichia coli both in the light and dark conditions.25 In an earlier report, ZnS nanospheres prepared by a facile one pot synthesis, involving the surfactant dodecyldimethyl ammonium bromide, exhibited high antibacterial activity and negligible mammalian cell toxicity.24 However, to the best of our knowledge, the antifungal properties of nano ZnS have not been explored yet. In this current investigation, we report the use of ZnS nanoparticles, synthesised by an ultrasonic assisted precipitation technique, for antifungal activity against the fungus Candida albicans (C. albicans).
2. Experimental
2.1. Preparation of ZnS nanoparticles
ZnS nanoparticles were synthesised by the chemical reaction of zinc chloride with sodium sulfide in solution. In a typical synthesis, 125 ml of 0.5 M aqueous solution of zinc chloride was subjected to ultrasonic irradiation using a probe sonicator (Vibronics, Mumbai, India). During sonication, 125 ml of 0.5 M sodium sulphide solution was added dropwise and the sonication was continued for 45 minutes until a whitish yellow thick precipitate of ZnS was obtained. The product was washed with an excess amount of water to remove any unreacted species and dried further in an oven at 70 °C.
For comparison experiments, commercially available ZnS particles (Aldrich, Bangalore, India) were used.
2.2. Characterisation
The ZnS obtained from sonochemical precipitation was characterised by X-ray diffraction using XRD (PW1710 Philips, The Netherlands) in the 2θ range of 10–80° using a Cu Kα radiation source. The Scherrer equation (eqn (1)) was employed for the determination of the crystallite sizes of the ZnS samples. |
 | (1) |
where ϕ is the crystallite size, λ is the X-ray wavelength, K is the shape factor, and β is the full line width at the half-maximum height of the major intensity peak. The luminescence spectrum was measured using a spectrofluorometer (Cary Eclipse, Varian, Netherlands). The powder morphology of the synthesized particles was observed under a transmission electron microscope (Tecnai G2, FEI, The Netherlands, operated at 300 kV). Particle size measurements were performed by photo-correlation spectroscopy of dilute suspensions of ZnS particles. BET surface area studies were carried out using a BET surface area analyser (Gemini 2375, Micromeritics, USA) after degassing the samples at 200 °C for 2 hours. Observation of fungi before and after exposure to ZnS was performed using a scanning electron microscope (Carl Zeiss, Germany).
2.3. Antifungal studies
2.3.1. Disc diffusion method. An agar disc diffusion method for antifungal tests was carried out using potato dextrose agar.30 The inoculum was prepared using 24 h plate cultures of test fungi C. albicans (MTCC 227). The colonies were suspended in 0.85% saline and the turbidity was compared with the 0.5 McFarland standards, to produce a 1 × 106 CFU ml−1 suspension. The suspension was loaded on a sterile cotton swab that was rotated several times and pressed firmly against the inside wall of the tube to remove excess inoculum from the swab. The dried surface of a potato dextrose agar plate was inoculated by streaking the swab over the entire sterile agar surface. The discs of ZnS prepared from both the synthesized nanoparticles (n-ZnS) and commercially available micron sized (m-ZnS) particles (0.3 g, 11 mm diameter) were placed on the top of the inoculated plates and incubated at 30 °C for 48 h. The antifungal activity was evaluated by measuring the zone of growth inhibition surrounding the discs. The larger the diameter of the zone of inhibition, the greater the antimicrobial activity.
2.3.2. The effect of ZnS concentration on C. albicans. Cultures of C. albicans grown on potato dextrose agar broth for 24 h were used for the quantitative antifungal studies by an optical density (OD) method at 600 nm. The viability and growth curve of C. albicans were measured by adding different amounts of ZnS nanoparticles to the culture (3 × 105 CFU ml−1) and subsequently measuring the OD at different time intervals. Control experiments were performed on cultures without n-ZnS.
2.3.3. Determination of the minimum fungicidal concentration of ZnS on C. albicans growth. The minimum fungicidal concentration (MFC) of ZnS nanoparticles on C. albicans was resolved by adding different concentrations of ZnS nanoparticles into the C. albicans culture and measuring the OD after 18 h.
2.3.4. The role of reactive oxygen species in antifungal activity. The role of reactive oxygen species (ROS) in antifungal activity is evaluated with the aid of histidine, a known ROS scavenger material. The C. albicans culture, containing a specific amount of ZnS nanoparticles (300 μg ml−1), was treated with different concentrations of histidine and the change in OD was monitored at different time intervals. Control experiments were performed as mentioned above.
3. Results
3.1. X-ray diffraction
Fig. 1 presents the X-ray diffraction patterns of the sonochemically synthesised ZnS in comparison with that of the commercial ZnS. The peak positions for both the ZnS particles at 2θ values of 28.6, 47.5 and 56.3 corresponded to the (111), (220) and (311) planes respectively and confirmed the crystallisation of ZnS in a cubic blende structure.
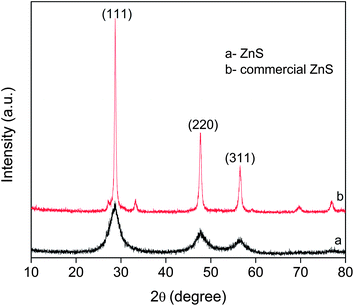 |
| Fig. 1 XRD patterns of ZnS particles synthesised by the sonochemical route (a) and commercially obtained (b). | |
A crystallite size of 3.4 ± 1 nm was estimated from the diffraction data using the Scherrer equation and calculated from the full width half maxima (FWHM) of the (111) peak. The m-ZnS displayed well defined peaks characteristic of highly crystallised particles and additional peaks at 2θ values of 70 and 78 corresponding to sphalerite phases are also seen.
3.2. Particle size distribution
The particle size distributions of n-ZnS and m-ZnS were estimated using photo-correlation spectroscopy and the number average plots are presented in Fig. 2. The ZnS nanoparticles, in the absence of surface modifiers, are in agglomerated form and the average agglomerate size is above 100 nm. The commercial ZnS particles were distinctly larger with particle sizes larger than 2 microns.
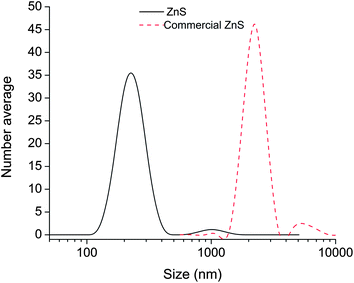 |
| Fig. 2 Particle size distribution plots for ZnS particles synthesised by sonochemical route (a) and commercially obtained (b). | |
3.3. Transmission electron microscopy
Fig. 3 provides the TEM images of the ZnS nanoparticles derived from the sonochemical precipitation. The particles appear agglomerated which is a characteristic feature of surfactant free precipitation reactions in aqueous media. Primary particles, 4–5 nm in size, were found to be spherical in morphology and exhibited a narrow distribution within the aggregates.
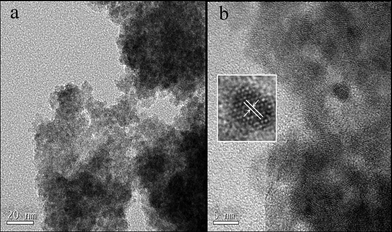 |
| Fig. 3 A TEM image of the ZnO particles obtained by sonochemical precipitation (a) and a HRTEM image showing individual particles (b). | |
This particle size value is in line with the crystallite size value of 3.4 ± 1 nm measured by XRD. The HRTEM image shown in Fig 3b indicates the appearance of lattice fringes confirming the crystallinity. The distance between two fringes is calculated (inset) to be 0.3 nm and is in good agreement for ZnS nanoparticles.
3.4. Disc diffusion test for antifungal activity
The n-ZnS and m-ZnS were then subjected to antifungal activity (against C. albicans) using the disc diffusion method. Fig. 4a and b show the photographs of agar plates containing ZnS discs (0.3 g, 11 mm diameter) inoculated with C. albicans fungi. The zone of inhibition, approximately 29.8 mm in diameter, surrounding the n-ZnS disc obtained from the sonochemical precipitation, clearly demonstrated the antifungal properties of the zinc sulphide nanoparticles. In contrast, the m-ZnS disc obtained from the commercial ZnS particles indicated negligible antifungal action, as indicated by the absence of a zone of inhibition.
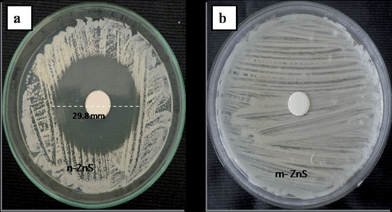 |
| Fig. 4 Photographs of agar plates containing (a) n-ZnS and (b) m-ZnS after 24 hours, displaying the results of the zone of inhibition test. | |
3.5. Cell morphology by SEM imaging
The SEM images of C. albicans with n-ZnS after 0 and 24 h are presented in Fig. 5a–c. In Fig. 5a, the oval shaped unicellular fungi are seen to be spread over the broth at the start of the test. Elongated cells are also occasionally observed. After 24 h there is a considerable decrease in the number of fungal cells observed. The magnified micrograph presented in Fig. 5c captures the decay of the fungal cells.
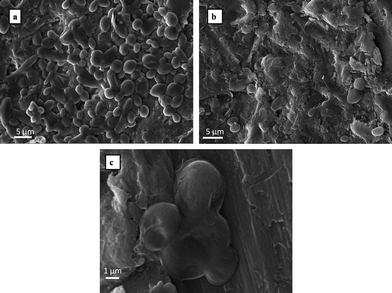 |
| Fig. 5 SEM micrographs of C. albicans in presence of n-ZnS at (a) the start of the test and (b) after 24 h. (c) A higher magnification image of (b). | |
3.6. Growth curves of C. albicans in the presence of n-ZnS
The ability of the ZnS nanoparticles to inhibit the growth of C. albicans was explored using different concentrations of n-ZnS in comparison with a control sample without ZnS. Fig. 6 represents the optical density at 600 nm against time for n-ZnS concentrations of 50–300 μg ml−1. It is clearly evident from the growth curves that the presence of n-ZnS retarded the growth of C. albicans. The growth inhibition was strongly influenced by the n-ZnS concentration and the steep rise in the growth curve observed for the control sample is significantly reduced in the presence of the n-ZnS. It is also observed that the logarithmic phase of the growth curve is delayed and the growth rate decreased with increasing concentrations of n-ZnS, as has been reported in the case of ZnO nanoparticles (ZnO NPs).8
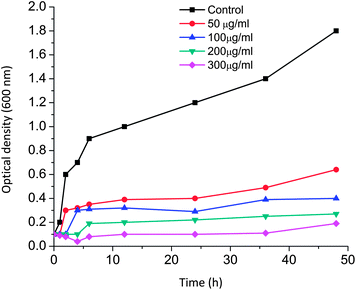 |
| Fig. 6 Growth curves of C. albicans with varying concentrations of n-ZnS compared with that of a control sample. | |
3.7. Minimum fungicidal concentration (MFC)
The lowest concentration of n-ZnS required to affect the viability of C. albicans was elucidated by a measurement of Minimal Fungicidal Concentration (MFC). Different concentrations of ZnS nanoparticles (5–500 μg ml−1) were added to C. albicans cultures and the survival fractions were analysed (Fig. 7). It has been found that the cell counts of C. albicans decreased as the concentration of ZnS increased. A minimum n-ZnS concentration of 300 μg ml−1 was essential to realise >90% fungicidal activity.
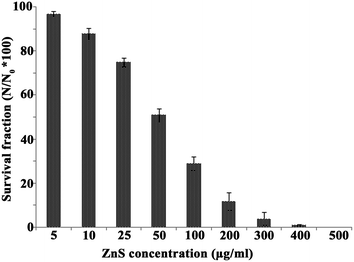 |
| Fig. 7 Estimation of survival fractions of C. albicans with varying concentrations of n-ZnS. | |
3.8. The role of histidine on the growth curves of C. albicans
The results shown in Fig. 4, 6 and 7 clearly indicate that the presence of ZnS nanoparticles (n-ZnS) or pellets made out of n-ZnS inhibits the growth of C. albicans. The most likely mechanism contributing to the antifungal activity of the nanoparticles is the generation of reactive oxygen species. One way to validate the ROS mechanism is by using hydroxyl radical scavengers like histidine.8,31 In the present study, the effect of histidine on the fungicidal activity of the n-ZnS was studied by analysing the optical density with time. Growth curves of cultures containing n-ZnS (300 μg ml−1) and varying amounts of histidine were estimated and the data presented in Fig. 8 clearly demonstrate a concentration dependent influence of histidine on the fungicidal activity of the n-ZnS. It was found that fungicidal activity was lower at higher histidine concentrations. The sample containing n-ZnS and 4 mM histidine showed a growth profile that more or less matched that of the control sample (without n-ZnS), indicating that the generated ROS is effectively scavenged by histidine. The results thus obtained revealed that the antifungal activity of the n-ZnS is primarily related to the ROS mechanism.
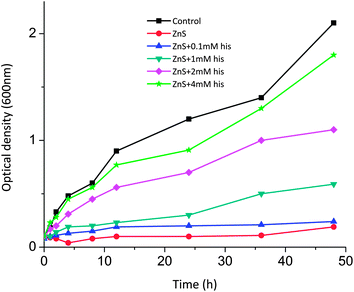 |
| Fig. 8 Growth curves of C. albicans in n-ZnS cultures containing varied concentrations of histidine. | |
3.9. Photoluminescence spectra
The luminescence spectrum of ZnS nanoparticles upon excitation at 270 nm is presented in Fig. 9. The broad peak observed in the wavelength region of 340–350 nm is generally ascribed to interstitial sulphur.32,33 The presence of interstitial zinc is denoted by the small peak observed in the wavelength region of 392–395 nm.32,33 The most prominent peak at 432 nm is characteristic of S vacancies in ZnS powders. The minor peak at 490 nm is associated with Zn vacancies.32,33 The observed peaks and their related defects suggest the existence of donor levels within the band gap.
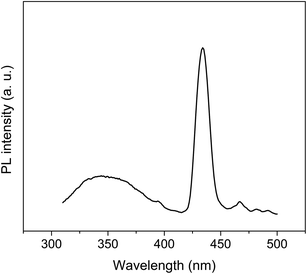 |
| Fig. 9 Photoluminescence spectrum of the n-ZnS. | |
3.10. Zn elemental mapping
In order to explore the possibility of mechanisms other than ROS, we have carried out elemental mapping studies on the zone of inhibition and Fig. 10 plots the Zn/Cl ratio against the distance from the outer edge of the disc in the zone of inhibition.
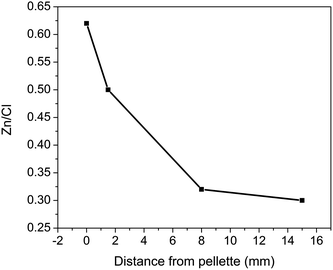 |
| Fig. 10 Variation of zinc concentration along the diameter of the zone of inhibition (Cl from brine solution was uniformly present in the reaction medium and was taken as the standard against which the decay in Zn concentration is represented). | |
As shown, there is a gradual decrease in the Zn concentration along the radius of the zone of inhibition. The presence of Zn along the zone of inhibition is presumably an indication of the Zn2+ efflux mechanism also contributing towards the antifungal activity.
4. Discussion
The antimicrobial properties of nanoparticles is ascribed to a combination of factors that include generation of ROS and efflux mechanisms leading to the release of constituent ions.1,8,11,12,22 The disruption of the cell membrane activity by the direct contact between the nanoparticles and cell membrane is also reported to be responsible for the antifungal activity of ZnO nanoparticles.17,34 The binding of nanoparticles to the microbe cell membrane, by direct or electrostatic forces, affects the permeability of the membranes and induces oxidative stress, preventing cell growth.17,35,36 It was previously shown that the smaller the particle size, the greater the antimicrobial activity will be.19,20,37–39 In the present study, the n-ZnS obtained by the sonochemical precipitation displayed remarkable antifungal action at a minimum fungicidal concentration of 300 μg ml−1. The inhibition of antifungal activity on addition of 4 mM histidine suggested the generation of ROS to be the most likely mechanism. n-ZnS with its intrinsic defects (clear from photoluminescence spectra) produced electron–hole pairs. The holes reacted with water producing H+ and OH− and the electrons converted dissolved oxygen molecules to superoxide radical anions. The superoxide radical anions thus obtained reacted with H+ producing HO2˙ radicals, which are in turn converted to hydrogen peroxide anions (HO2−). Hydrogen peroxide is then obtained by the reaction of these anions with hydrogen ions. The peroxide can penetrate the fungal cell membranes and disrupt them due to oxidative stress.18,40 The generation of hydrogen peroxide thus forms the basis of antimicrobial activity in such nanoparticles. In the present study, the antifungal tests were all performed without additional light irradiation in a test chamber and therefore the genesis of hydrogen peroxide is facilitated by a vacancy induced photo-mechanism. In a very recent article,41 three kinds of ZnO, namely tetrapod-like ZnO whiskers, nanosized ZnO particles and micron sized ZnO particles, were tested for antimicrobial activity against E. coli and the authors invoked a mechanism based on the oxygen vacancies in the surface layer of ZnO. The particle size and surface area of the three types of ZnO samples were shown to be less influential on the generation of H2O2. In the case of n-ZnS, the presence of S vacancies, substantiated by PL measurements, is the most obvious source of band transitions leading to the generation of H2O2. The observance of fungicidal activity without light irradiation is thus presumed to be due to the availability of sulphur vacancies providing donor levels within the band gap.
The diffusion of Zn2+ in the agar medium and its activity with the cell membranes of the fungi should directly influence the size of the zone of inhibition. It should be noted that the ZnS nanoparticles displayed a zone of inhibition of ∼30 mm diameter. Elemental mapping studies revealed the leaching of n-ZnS into the inhibition zone. The concentration of Zn in the zone of inhibition was highest close to the n-ZnS disc and it gradually decreased along the radius of the zone of inhibition. This suggested the likelihood of an efflux mechanism where Zn2+ ions can cross the fungal cell membrane causing irreversible damage and ultimately, cell death.
The most widely investigated nanoparticle system for antifungal activity is ZnO. The minimum fungicidal concentration reported for ZnO varies between 30–200 μg ml−1 depending on the method of synthesis. In the present study, the MFC of the n-ZnS was observed to be around 300 μg ml−1. The difference in MFC of ZnO and ZnS can presumably be due to the availability of dissolved oxygen species, in ZnO containing medium, arising out of the oxygen related defects like oxygen vacancies.11,41,42 However, due to the large difference in the solubility of ZnO and ZnS in water (ZnO nanoparticles are shown to be soluble up to 7–7.5 mg L−1 while ZnS possesses negligible solubility), the outdoor applications of ZnS based antifungal coatings can presumably be of longer durability.
5. Conclusions
This work, to the best of our knowledge, constitutes the first study of the antifungal properties of ZnS nanoparticles against the increasingly drug resistant fungus Candida albicans. The sonochemical synthetic route produced n-ZnS with S vacancies that led to the generation of hydrogen peroxide in aqueous suspensions. The generation of ROS as the dominating mechanism is confirmed by the observation that the fungicidal activity of the n-ZnS is inhibited by the addition of 4 mM histidine, a scavenger of hydroxyl radical and singlet oxygen. Additionally, elemental mapping studies carried out along the radius of the zone of inhibition revealed the presence of Zn in decreasing concentrations. This efflux mechanism releasing Zn2+ ions also contributes towards the disruption of fungal cell membranes inducing irreversible damage and cell death. Zinc sulphide nanoparticles thus emerge as a potential antifungal agent that can be used to eliminate fungi related infections.
Acknowledgements
The authors acknowledge the Council of Scientific and Industrial Research (CSIR, Government of India) for funding the 12th five year plan project on “IntelCoat” (CSC0114). SCP would like to thank Science Foundation Ireland (SFI/12/ISCA/2493) for funding.
References
- N. Perkas, A. Lipovsky, G. Amirian, Y. Nitzan and A. Gedanken, J. Mater. Chem. B, 2013, 1, 5309 RSC.
- K. Page, R. G. Palgrave, I. P. Parkin, M. Wilson, S. L. P. Savin and A. V. Chadwick, J. Mater. Chem., 2007, 17, 95 RSC.
- C. W. Dunnill, Z. A. Aiken, A. Kafizas, J. Pratten, M. Wilson, D. J. Morgan and I. P. Parkin, J. Mater. Chem., 2009, 19, 8747 RSC.
- C. W. Dunnill, Z. Ansari, A. Kafizas, S. Perni, D. J. Morgan, M. Wilson and I. P. Parkin, J. Mater. Chem., 2011, 21, 11854 RSC.
- H. Bai, Z. Liu and D. D. Sun, Phys. Chem. Chem. Phys., 2011, 13, 6205 RSC.
- S. Ghosh, V. S. Goudar, K. G. Padmalekha, S. V. Bhat, S. S. Indi and H. N. Vasan, RSC Adv., 2012, 2, 930 RSC.
- T. Gordon, M. Kopel, J. Grinblat, E. Banin and S. Margel, J. Mater. Chem., 2012, 22, 3614 RSC.
- A. Lipovsky, Y. Nitzan, A. Gedanken and R. Lubart, Nanotechnology, 2011, 22, 105101 CrossRef PubMed.
- N. E. A. El-Gamel, Dalton Trans., 2013, 42, 9884 RSC.
- I. Perelshtein, E. Ruderman, N. Perkas, T. Tzanov, J. Beddow, E. Joyce, T. J. Mason, M. Blanes, K. Molla, A. Patlolla, A. I. Frenkel and A. Gedanken, J. Mater. Chem. B, 2013, 1, 1968 RSC.
- A. S. Haja Hameed, C. Karthikeyan, S. Sasikumar, V. Senthil Kumar, S. Kumaresan and G. Ravi, J. Mater. Chem. B, 2013, 1, 5950 RSC.
- Y. Wang, F. Huang, D. Pan, B. Li, D. Chen, W. Lin, X. Chen, R. Li and Z. Lin, Chem. Commun., 2009, 6783 RSC.
- S. Agnihotri, S. Mukherji and S. Mukherji, Nanoscale, 2013, 5, 7328 RSC.
- M. Nocchetti, A. Donnadio, V. Ambrogi, P. Andreani, M. Bastianini, D. Pietrella and L. Latterini, J. Mater. Chem. B, 2013, 1, 2383 RSC.
- M. A. Gondal, A. J. Alzahrani, M. A. Randhawa and M. N. Siddiqui, J. Environ. Sci. Health, Part A: Environ. Sci. Eng., 2012, 47, 1413 CrossRef CAS PubMed.
- G.-X. Tong, F.-F. Du, Y. Liang, Q. Hu, R.-N. Wu, J.-G. Guan and X. Hu, J. Mater. Chem. B, 2013, 1, 454 RSC.
- P. K. Stoimenov, R. L. Klinger, G. L. Marchin and K. J. Klabunde, Langmuir, 2002, 18, 6679 CrossRef CAS.
- G. Applerot, A. Lipovsky, R. Dror, N. Perkas, Y. Nitzan, R. Lubart and A. Gedanken, Adv. Funct. Mater., 2009, 19, 842 CrossRef CAS.
- J. Sawai, H. Igarashi, A. Hashimoto, T. Kokugan and M. Shimizu, J. Chem. Eng. Jpn., 1996, 29, 251 CrossRef CAS.
- O. Yamamoto, Int. J. Inorg. Mater., 2001, 3, 643 CrossRef CAS.
- S. Nair, A. Sasidharan, V. V. Divya Rani, D. Menon, S. Nair, K. Manzoor and S. Raina, J. Mater. Sci.: Mater. Med., 2009, 20, 235 CrossRef PubMed.
- Z. H. Yang and C. S. Xie, Colloids Surf., B, 2006, 47, 140 CrossRef CAS PubMed.
- R. Brayner, R. Ferrari-Iliou, N. Brivois, S. Djediat, M. F. Benedetti and F. Fievet, Nano Lett., 2006, 6, 866 CrossRef CAS PubMed.
- G. Li, J. Zhai, D. Li, X. Fang, H. Jiang, Q. Dong and E. Wang, J. Mater. Chem., 2010, 20, 9215 RSC.
- D. W. Synnott, M. K. Seery, S. J. Hinder, G. Michlits and S. C. Pillai, Appl. Catal., B, 2013, 130, 106 CrossRef PubMed.
- D. W. Synnott, M. K. Seery, S. J. Hinder, J. Colreavy and S. C. Pillai, Nanotechnology, 2013, 24, 045704 CrossRef CAS PubMed.
- J. H. Bang, R. J. Helmich and K. S. Suslick, Adv. Mater., 2008, 20, 2599 CrossRef CAS.
- H. R. Pouretedal, A. Norozi, M. H. Keshavarz and A. Semnani, J. Hazard. Mater., 2009, 162, 674 CrossRef CAS PubMed.
- J. S. Hu, L. L. Ren, Y. G. Guo, H. P. Liang, A. M. Cao, L. J. Wan and C. L. Bai, Angew. Chem., Int. Ed., 2005, 44, 1269 CrossRef CAS PubMed.
- S. Ghasemi, S. Abbasi, S. Bahraminejad and B. Harighi, Australas. Plant Pathol., 2012, 41, 331 CrossRef.
- M. Shoeb, R. S. Braj, A. K. Javed, K. Wasi, N. S. Brahma, B. S. Harikesh and H. N. Alim, Adv. Nat. Sci.: Nanosci. Nanotechnol., 2013, 4, 035015 CrossRef.
- M. V. Limaye, S. Gokhale, S. A. Acharya and S. K. Kulkarni, Nanotechnology, 2008, 19, 415602 CrossRef PubMed.
- A. V. Murugan, O. Y. Heng, V. Ravi, A. K. Viswanath and V. Saaminathan, J. Mater. Sci., 2006, 41, 1459 CrossRef CAS.
- R. Brayner, R. Ferrari-Iliou, N. Brivois, S. Djediat, M. F. Benedetti and F. Fiévet, Nano Lett., 2006, 6, 866 CrossRef CAS PubMed.
- Y. Xie, Y. He, P. L. Irwin, T. Jin and X. Shi, Appl. Environ. Microbiol., 2011, 77, 2325 CrossRef CAS PubMed.
- L. Zhang, Y. Jiang, Y. Ding, M. Povey and D. York, J. Nanopart. Res., 2007, 9, 479 CrossRef CAS.
- J. Sawai and T. Yoshikawa, J. Appl. Microbiol., 2004, 96, 803 CrossRef CAS.
- O. Yamamoto, M. Hotta, J. Sawai, T. Sasamoto and H. Kojima, J. Ceram. Soc. Jpn., 1998, 106, 1007 CrossRef CAS.
- S. Makhluf, R. Dror, Y. Nitzan, Y. Abramovich, R. Jelinek and A. Gedanken, Adv. Funct. Mater., 2005, 15, 1708 CrossRef CAS.
- M. Fang, J. H. Chen, X. L. Xu, P. H. Yang and H. F. Hildebrand, Int. J. Antimicrob. Agents, 2006, 27, 513 CrossRef CAS PubMed.
- X. Xu, D. Chen, Z. Yi, M. Jiang, L. Wang, Z. Zhou, X. Fan, Y. Wang and D. Hui, Langmuir, 2013, 29, 5573 CrossRef CAS PubMed.
- J. Becker, K. R. Raghupathi, J. St. Pierre, D. Zhao and R. T. Koodali, J. Phys. Chem. B, 2011, 115, 13844 CAS.
|
This journal is © The Royal Society of Chemistry 2014 |