DOI:
10.1039/C3RA46399K
(Paper)
RSC Adv., 2014,
4, 8341-8349
Controlled growth of spinel NiCo2O4 nanostructures on carbon cloth as a superior electrode for supercapacitors
Received
4th November 2013
, Accepted 5th December 2013
First published on 5th December 2013
Abstract
In this study, the morphology conversion of bimetallic NiCo2O4 nanostructures on carbon fiber cloth (CFC) was achieved via a simple hydrothermal approach with different precursor salts. As expected, the surface morphology has been successfully driven by varying the precursor. Typical NiCo2O4 nanowall-networks and porous nanoflake microstructures have been grown when using the nitrate and chloride precursors respectively. As an advantage of their unique structural features, they have shown different electrochemical activity towards supercapacitor applications. The as-grown NiCo2O4 nanowall-network structure delivers a maximum capacitance of 1225 F g−1 at a high current density of 5 A g−1 and excellent durability. However, a limited specific capacitance of only 844 F g−1 at a current density of 1 A g−1 was achieved for NiCo2O4 nanoflakes. This variation in the electrochemical features such as specific capacitance, rate capability and cyclic stability is mainly due to their structural discrepancies which have been driven by the precursors during the growth process. From this investigation it can be concluded that precursors with different anions also greatly influence the growth kinetics of metal oxide nanostructures. In this case, we suggest that the directly grown NiCo2O4@CFC with the desired microstructure will be a potential electrode for next generation flexible supercapacitors.
Introduction
The design of smart and flexible energy storage devices has received great interest for portable electronics applications.1–4 The supercapacitor is a kind of energy storage device that can compete with lithium batteries with their high power density, good durability, long term cyclic stability and safety.2 In particular, flexible supercapacitors are potential candidates for sensor networks, stretchable integrated circuits and in the field of medical electronics with the required high power density at very short time delay.3 It is well established that the energy storage performance of supercapacitors is directly related to the electrode materials. To date three kinds of electrode materials have been explored to fabricate the supercapacitors. Based on the charge storage mechanism, these have been categorized as follows: carbonaceous materials which store energy by the adsorption of charge at the electrode/electrolyte interfaces.4,5 Alternatively, faradaic type pseudo-capacitive electrode materials including high oxidation state transition metal oxides (RuO2, MnO2, NiO, Co3O4, & NiCo2O4) and conducting polymers (PANI) have also been extensively investigated for supercapacitors.6–24 With the desirable structural features and electrochemical activity, metal oxides have received more attention as supercapacitor electrodes. Recently, directly grown metal oxide nanostructures on conductive substrates have been explored as electrodes for high performance supercapacitors. With a view to flexible devices, the commonly known nickel foam, ITO glass and other metal current collectors are limited due to their lower mechanical strength and non-flexibility. Therefore it is significant to develop and design novel flexible electrodes with the desired electrical conductivity, mechanical integrity and flexibility.
Fabrication of high performance flexible supercapacitors is still a challenging task to researchers because it requires stretchable electrodes with great mechanical strength and the desired electrochemical features. Among various flexible electrodes, carbon fiber cloth (CFC) is an ideal candidate with the required specifications such as superior electrical conductivity, high flexibility and chemical compatibility.2 Further, it acts as a current collector as well as a binder free flexible electrode for supercapacitors. In this regard significant efforts have been made with different kinds of electrode material; Qian Cheng et al. studied PANI deposited etched CFC as a desirable electrode for supercapacitors.4 Recently, Feng Luan et al. reported NiO nanoflakes on carbon fiber cloth for asymmetric supercapacitors.25 Similarly, Ying-Chu Chen et al. have grown MnO2 nanosheets on carbon fiber cloth for flexible supercapacitors.26 Previously, Jingwen Zhao et al. reported Co–Mn layered double hydroxide nanowalls supported on carbon fibers for high performance flexible energy storage devices.27 However, the areal capacitance of these flexible electrodes is still unsatisfactory because of their inadequate morphological features. The change in morphology strongly influences the electrodes’ surface area and porosity which are directly related to the electrochemical activity. It is well established that the final morphology of the electrode is driven by the following factors: synthetic process, hydrolyzing agent and precursor. Recently, Shung Chang et al. prepared the NiO nanoflake and nanonet/nanoflower morphologies by electrodeposition and hydrothermal processes respectively.1 Controlled growth of NiCo2O4 nanowires and nanosheets on carbon nanofibers with different hydrolyzing agents has been reported by Genqiang Zhang and Xiong Wen (David) Lou.28 Similarly, Huanwen Wang and Xuefeng Wang have grown NiCo2O4 nanowires and nanosheets on a carbon cloth substrate by a hydrothermal approach with mixed solvents as well as different hydrolyzing agents.29 Previously, we have designed CeO2 hexagonal nanoplates and nanorods by a facile hydrothermal approach with different hydrolyzing agents.30 In addition, Meher et al. and Qiang Wu et al. reported a detailed morphological evaluation of NiO and CeO2 nanostructures based on the precursors.31,32 Further, all the reports have shown different electrochemical responses towards supercapacitor applications with respect to their morphological features. From the above findings it can be understood that the electrode morphology plays a major role in predicting the electrochemical performance of the material.
Concerning the specific capacitance, NiCo2O4 could be a more desirable electrode material because of its multi-oxidation states and high electrical conductivity. Therefore, numerous efforts have been made to explore NiCo2O4 as a superior electrode for supercapacitors. However, the growth of NiCo2O4 nanostructures on flexible substrates with high mass loading and ultra-high specific capacitance at high current density has not yet been reported and it needs further investigation. To improve the specific capacitance and rate capability, the morphology should be tuned by varying the factors discussed earlier. In this report we have grown NiCo2O4 spinel oxide nanostructures on a carbon fiber cloth substrate with different precursors. The influence of the precursor salt on the growth process, electrode morphology and the corresponding electrochemical performance has been investigated for high performance flexible supercapacitors. As expected, interesting morphological and electrochemical activity have been achieved for these CFC supported NiCo2O4 spinel nanostructures.
Materials and methods
Synthesis of NiCo2O4 nanowall network
Analytical grade nickel nitrate and cobalt nitrate were purchased from Alfa Aesar and used as received without further purification. Highly conductive CFC was obtained from Cobat Carbon Inc. To anchor the NiCo2O4 on carbon fiber cloth a simple hydrothermal approach was employed. A typical synthetic procedure is as follows: Ni(NO3)3·6H2O and Co(NO3)3·6H2O were dissolved in a stoichiometric (Ni
:
Co = 1
:
2) ratio in a reaction vessel containing 160 mL deionized water and ethanol (1
:
1) at equal volume. Further, an excess amount of urea was added under stirring for the homogenous hydrolysis process. Ultrasonically pretreated CFC (4 × 4 cm) was poured into the reaction vessel containing the mixed nitrates solution and kept in an electric oven at 120 °C for 8 h. Finally, the solution was cooled down to room temperature and the CFC was removed from the solution. After washing with deionized water, ethanol and acetone the CFC was dried at 60 °C under vacuum and heat treated at 350 °C for 2 h for oxidation.
Synthesis of NiCo2O4 nanoflakes
To grow the NiCo2O4 nanoflakes on CFC, analytical grade NiCl2·4H2O and CoCl2·4H2O were dissolved in an equal volume of ethanol and water mixed solvent in a stoichiometric ratio (Ni
:
Co = 1
:
2). Then, an excess amount of urea was added to the solution and the stirring was continued to obtain a clear pink colored homogenous solution. Ultrasonically pretreated CFC (4 × 4 cm) was poured into the reaction vessel and kept at 120 °C for 8 h in an electric oven. After cooling down to room temperature, the CFC was taken out and cleaned ultrasonically with deionized water, ethanol and acetone. The as obtained CFC was dried at 60 °C under vacuum followed by heat treatment for 2 h at 350 °C.
Characterization techniques
Crystalline phases of the products were investigated by powder X-ray diffraction (Bruker, D2-PHASER) with Cu Kα (λ = 1.5406 Å). The morphologies of the samples were examined by high-resolution scanning electron microscopy (HRSEM; FEI QUANTA FEG200) and high resolution transmission electron microscopy (HRTEM; TECNAI operated at 200 kV). Raman spectra of the samples were taken under visible excitation at 514.3 nm using a Confocal Laser Raman Spectrophotometer. The N2 adsorption and desorption measurements were performed with the Quantachrome QuadraWin (Version 5.02) analyzer.
Electrochemical measurements with three electrode configuration were carried out in a CHI 7007C electrochemical workstation at room temperature. 3 M KOH solutions were used as the supporting electrolyte. The mass of the active materials was calculated from the weight difference of the CFC before and after growth of NiCo2O4. As grown NiCo2O4 on carbon fiber with a typical mass of ∼1 mg was used directly as the working electrode. Platinum wire and the standard calomel electrode (SCE) were used as the counter and reference electrodes respectively. Electrochemical impedance spectroscopy (EIS) measurements were carried out by an applying an AC voltage with 5 mV amplitude in a frequency range from 0.1 Hz to 100 kHz at open circuit potential.
Results and discussion
Phase analysis
The crystallographic structures of the products were analyzed by powder X-ray diffraction as shown in Fig. 1. All three patterns show the dominant diffraction peaks at 25.8° and 42.9° due to the carbon fiber cloth. With the precursor, a typical XRD pattern was observed and shows new diffraction peaks as shown in Fig. 1b and c. The peak positions and their relative intensities can be directly indexed to the characteristic spinel NiCo2O4, and are consistent with the standard JCPDS (#73-1702) card.18–21 The NiCo2O4 nanowall microstructures show the broad diffraction peaks at 30.7°, 36.5° and 63.6° associated with the (220), (311) and (440) planes of the NiCo2O4 spinel. Whereas, the NiCo2O4 nanoflake microstructures show all the characteristic diffraction peaks which are positioned at 18.8°, 30.7°, 36.5°, 43.5°, 58.9° and 63.6° as shown in Fig. 1c. Broad diffraction peaks with lower intensity are ascribed to the formation of nanocrystallites. No additional diffraction peaks related to NiO or Co3O4 were observed which confirms the phase purity of the compound. From the XRD patterns it can be observed that the crystalline nature and crystallite size of the ternary oxide are directly related to the material growth rate which depends on the nature of the precursors and their anion size.31
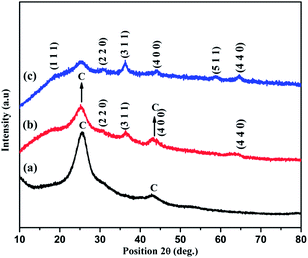 |
| Fig. 1 XRD patterns of pure CFC (a), as grown NiCo2O4 nanowalls (b) and self-aggregated nanocrystals (c). | |
Raman studies
The growth of NiCo2O4 on carbon fiber cloth was further confirmed with laser Raman spectra as shown in Fig. 2. The spectrum of pure CFC shows disordered D-band carbon and graphitic G-band carbon bands at 1353 cm−1 and 1581 cm−1 respectively.33–36 The peak at 2570 cm−1 represents the second order G-band vibration of the carbon. After the growth of NiCo2O4, the new bands are visible in the low wavenumber region (400–700 cm−1) as shown in Fig. 2 inset. These bands are mainly associated with Ni–O and Co–O vibrations of the NiCo2O4 spinel oxide.33,35 Noticeably, the band signals positioned at 190 cm−1, 459 cm−1, 505 cm−1 and 655 cm−1 are assigned to the F2g, Eg, F2g, and A1g active modes of the NiCo2O4 cubic structure which further supports the growth of NiCo2O4 on the CFC surface.35
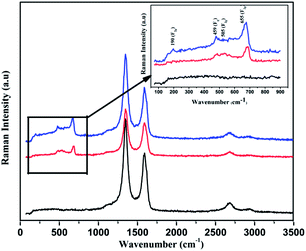 |
| Fig. 2 Raman spectra of pure carbon fiber cloth (black) and NiCo2O4 grown on carbon fiber cloth with nitrate precursor (red) and with chloride precursor (blue). | |
Morphological analysis
The morphological analysis of the NiCo2O4 nanostructures on CFC was directly carried out by HRSEM. Fig. 3a–d and 4a–d illustrate the SEM images of the NiCo2O4 nanostructures grown with nitrate and chloride precursors respectively. The low and high magnification SEM images show the dense growth of NiCo2O4 nanostructures on the CFC surface. From Fig. 3 it is observed that well connected nanowall network microstructures were grown during nitrate–urea hydrolysis. The average wall thickness was found to be in the range of ∼10–13 nm. Surprisingly, a typical self-aggregated NiCo2O4 nanoflakes morphology was observed for chloride–urea hydrolysis. The changes in morphology with the precursor are mainly attributed to the variation in the pH of the solution during the growth process. These results further confirm that precursors with different sized anions indirectly change the morphology of NiCo2O4 under hydrothermal conditions. A similar result has been reported by Meher et al. for NiO nanostructures.31 However the change in morphology and the influence of intercalated anions on the internal microstructure is not clear yet. The energy dispersive X-ray absorption spectra of the NiCo2O4 nanowalls and nanoflakes are shown in Fig. 5a and b respectively. They further designate the presence of functional elements (Ni, Co, O & C) at appropriate concentrations in the composite spinel oxides.
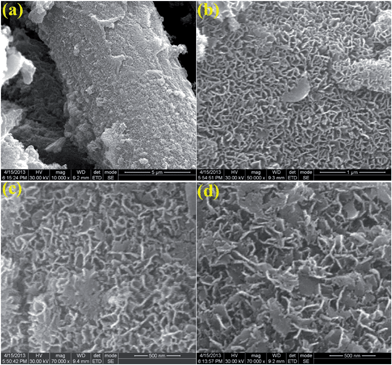 |
| Fig. 3 HRSEM images of as-grown NiCo2O4 nanowall superstructures with different magnifications. | |
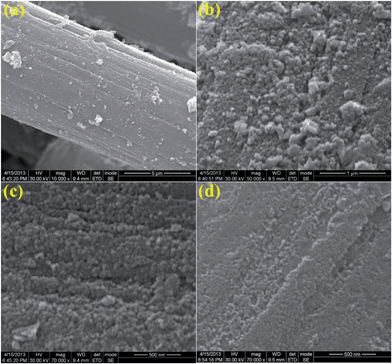 |
| Fig. 4 HRSEM images of as-grown self-aggregated NiCo2O4 nanocrystals with different magnifications. | |
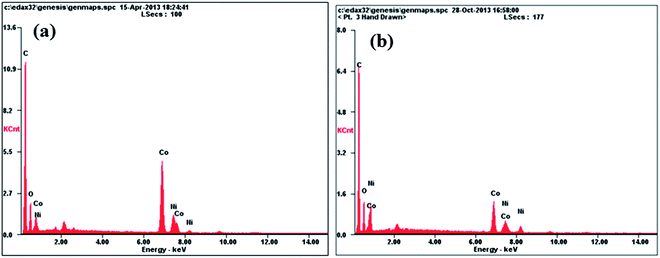 |
| Fig. 5 EDX spectra of NiCo2O4 nanowall superstructures (a) and self-aggregated nanocrystals (b). | |
In order to evaluate the clear structural features, HRTEM studies were carried out and are shown in Fig. 6 and 7. Fig. 6a–c display the TEM images of NiCo2O4 nanowalls scratched off from the carbon cloth, which indicate that the fine nanocrystals with approximate diameter of 10–20 nm have connected together and form the nanowall network over the CFC surface. From the HRTEM image (Fig. 6c), the measured lattice spacing of ∼0.28 nm corresponds to the (311) crystal plane of the spinel NiCo2O4. A typical selected area electron diffraction (SAED) pattern (Fig. 6d) demonstrates the polycrystalline nature of the NiCo2O4 nanowalls. Further, all the diffraction rings in the SAED pattern can be indexed to the cubic spinel NiCo2O4 crystal structure and are consistent with the XRD analysis. Similarly, TEM images of NiCo2O4 nanoflakes scratched off from the CFC are shown in Fig. 7a–c which clearly evidence the presence of self-aggregated nanocrystals with 15–30 nm diameters. The estimated d-spacing of the (311) plane of the NiCo2O4 nanoflakes is found to 0.29 nm which is consistent with the standard JCPDS (#73-1702) file. Further, the cubic spinel crystal structure was demonstrated by the observed SAED pattern and its intense rings (Fig. 7d).
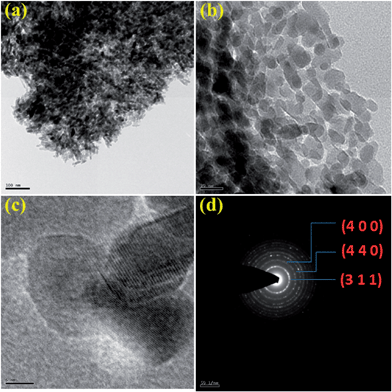 |
| Fig. 6 TEM images of as-grown NiCo2O4 nanowall structures scratched off from the CFC (a–c) and the corresponding SAED pattern (d). | |
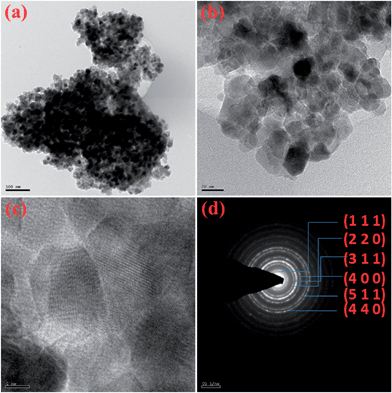 |
| Fig. 7 TEM images of as-grown self-aggregated NiCo2O4 nanocrystals scratched off from the CFC (a–c) and the corresponding SAED pattern (d). | |
A typical NiCo2O4 nanostructure growth mechanism is proposed as follows: here the urea has control of the hydrolysis process and produces a large number of OH− ions during hydrothermal decomposition. A typical hydrolysis process of urea is as follows.21,30,32
CO(NH2)2 (excess) + nH2O → nNH4OH + CO2↑ |
nNH4OH (excess) → nNH4+ + nOH− |
(Ni2+, 2Co2+) + nOH− → (Ni, Co2)(OH)6 + 1/2O2 → NiCo2O4 + 3H2O |
The urea hydrolysis mechanism includes the formation of excess ammonium hydroxide followed by the production of more hydroxyl ions at the end. Under hydrothermal conditions, the decomposition of ammonium to ammonia can raise the solution pH to ∼8 and produce more mixed hydroxide precipitates.37 During hydrolysis both Ni2+ and Co2+ metal cations were electrostatically adsorbed by the porous CFC substrate. During the extended hydrothermal process, the corresponding anion intercalated mixed double hydroxides grew over the CFC surface. After calcination at 350 °C for 2 h, the mixed hydroxides released the intercalated anions and internally bonded water molecules to form the spinel NiCo2O4. According to Meher et al. and Qiang Wu et al., the intercalated anions directly influence the final morphology of the materials.31,32 In this study, the controlled growth of NiCo2O4 with nanowall structures was observed when nitrate precursors were used. This may be attributed to the controlled and homogenous nucleation associated with the urea–nitrate hydrolysis. Surface adsorbed NO3− anions are significant influences on the growth kinetics due to their larger size which leads to the typical nanowall microstructures with open voids. For chloride–urea hydrolysis, the solution pH might be higher (∼≥9) and it increases the nucleation rate considerably. This results in the growth of self-aggregated nanocrystals. Finally, NiCo2O4 nanoflakes were formed on the CFC surface after the decomposition of intercalated anions and water molecules. This result further supports the morphology conversion by precursors with different anions as reported earlier.31
Surface area analysis
Fig. 8a and b present the N2 adsorption and desorption isotherms of the as-grown NiCo2O4 nanowalls and nanoflakes respectively. Both samples show the typical hysteresis loop in the relative pressure range of 0.7–1.0 P/P0 and demonstrate the presence of mesopores. The measured Brunauer–Emmett–Teller (BET) surface area value for the NiCo2O4 nanowalls and nanoflakes are 84.32 and 38.22 m2 g−1.38,39 From the Barret–Joyner–Halenda (BJH) pore size distribution curve shown in Fig. 8 (insets), the wide ranging distribution of mesopores can be observed. The NiCo2O4 nanowall structures show the desired pore size distribution at ∼10 nm (Fig. 8a inset) with a pore volume of up to 0.02 cm3 g−1. Whereas the NiCo2O4 nanoflakes exhibit micro/meso range pores with a pore volume of 0.01 cm3 g−1 (Fig. 8b inset). From this observation it can be concluded that the NiCo2O4 nanowall structures have high surface area, large pore volume and narrow pore distribution that can lead to their superior electrochemical performance.
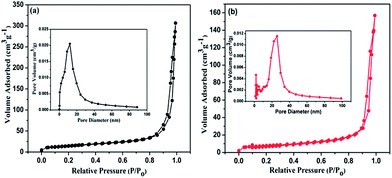 |
| Fig. 8 Nitrogen adsorption and desorption isotherms of NiCo2O4 nanostructures: (a) nanowall superstructures and (b) self-aggregated nanocrystals and the insets show the corresponding BJH pore size distributions. | |
Electrochemical studies
It is quite interesting to evaluate the electrochemical performance of the same material with different morphologies. First, the electrochemical performance of the as-grown NiCo2O4 nanowall structures was studied by cyclic voltammetry and charge/discharge studies within the potential range of −0.1–0.45 V vs. SCE. Fig. 9a shows the CV curves of the NiCo2O4 nanowall structures at various scan rates ranging from 2–20 mV s−1. When compared to the pure carbon cloth (not shown here), the visible deviation in shape of the CV curve reveals the pseudo-type capacitive characteristics of the sample. The possible redox mechanism is as follows:18,29 |
NiCo2O4 + OH− + H2O ↔ NiOOH + 2CoOOH + 2e−
| (1) |
|
CoOOH + OH− ↔ CoO2 + H2O + e−
| (2) |
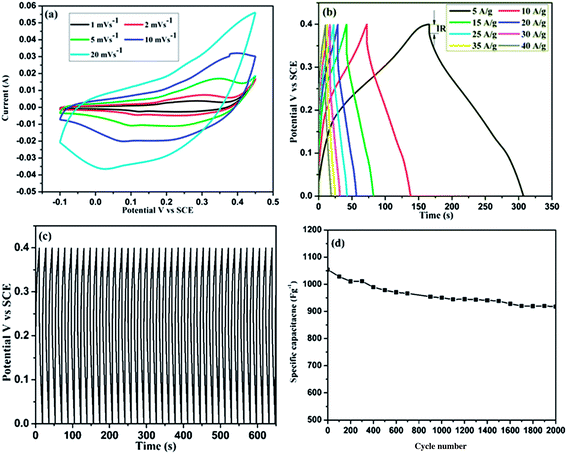 |
| Fig. 9 Electrochemical performances of NiCo2O4 nanowall superstructures: (a) CV curves at various scan rates, (b) charge/discharge voltage profiles at various current densities, (c) continuous charge/discharge cycles at the constant current density of 20 A g−1 and (d) the capacitance cycling performance at a constant current density of 20 A g−1. | |
When the scan rate increased, the shape of the CV curve changed gradually and the corresponding redox current also increased which indicates the controlled diffusion process within the electrode.40 The well resolved pair of redox peaks in the CV curve directly evidence the faradaic reaction associated with the Ni2+/Ni3+ or Co2+/Co3+ on the electrode surface.19 The difference in the CV curve clearly indicates the effective contribution of the CFC substrate in the electrochemical reaction. Therefore it could not be negligible for these nanowall structures. From the CV curve the average specific capacitance value can be estimated using the following relation as reported earlier:24
|
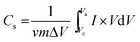 | (3) |
where
Cs is the specific capacitance (F g
−1),
I is the charging or discharging current,
v is the scan rate (V s
−1),
m is the mass of the active material (g) and Δ
V is the potential window (
Va −
Vc). After eliminating the substrate contribution (∼45 F g
−1 at 1 mV s
−1), the observed specific
Cs value for the NiCo
2O
4 nanowall structures is about 1214 F g
−1 at 1 mV s
−1. Further increasing the scan rate 20-fold, it retains up to 78% of the
Cs, which is ascribed to the high rate capability of the electrode. For further confirmation constant current charge/discharge measurements were employed.
Fig. 9b shows the typical charge/discharge profile of the NiCo
2O
4 nanowall structures with different current densities. In order to avoid the oxygen evolution, the electrode was charged and discharged within the potential range of 0–0.4 V
vs. SCE at different current densities ranging from 5–40 A g
−1. The good symmetry and nearly triangular shape of the charge/discharge curves for all the current densities further elucidate the good capacitive characteristics of the material even at high current density. Conversely, the immediate voltage (iR) drop during discharge represents the internal resistance of the component. From the charge/discharge curve, the specific capacitance value can be calculated using the following relation
24 |
 | (4) |
where
I is the discharge current, Δ
t is the discharge time,
m is the active material mass, and Δ
V is the potential difference (0.4 −
IR V). The estimated
Cs value for the stacked nanowall structures is 1225 F g
−1 at a constant current density of 5 A g
−1. When the current density was increased to 40 A g
−1, the corresponding
Cs value reduced to 996 F g
−1 as shown by the fast electron transfer at high current density. A relatively high
Cs value at high current density suggests the desired nanowall superstructures with direct contact on CFC leads to fast and efficient energy storage within the electrode. To evaluate the cyclic performance and electrochemical stability of the electrode, the material was tested by continuous charging–discharging measurements at a high current density of 20 A g
−1 for 2000 cycles as shown in
Fig. 9c. The NiCo
2O
4 nanowall structures show an excellent electrochemical stability and exhibit a
Cs of ∼917 F g
−1 after 2000 cycles. The overall efficiency of these NiCo
2O
4 nanowall network structures is about 87%, which is much higher than those of similar NiCo
2O
4 nanostructures reported earlier.
18–20,29 The observed ultra-high specific capacitance and excellent cyclic stability of the CFC supported NiCo
2O
4 open network nanowall superstructures may offer the following structural advantages for electrochemical activity: the strongly interconnected open network offers a large space for electrolyte ion diffusion and acts as an ion reservoir. High surface area and mesoporous microstructure could enable the core active sites in the redox process. Further, the thin wall nanostructures facilitate easy ion diffusion and enhance the specific capacitance significantly. In addition to the above the CFC backbones strengthen the binding between CFC and NiCo
2O
4 which leads to the admirable cyclic stability over 2000 cycles.
Similarly, hydrothermally grown NiCo2O4 nanoflakes@CFC was evaluated as an electrode for supercapacitors. The CV curves of self-aggregated NiCo2O4 nanoflake structures at various scan rates are shown in Fig. 10a. They show a well resolved pair of redox peaks within the potential range −0.2–0.4 V corresponding to the faradaic redox reaction based on the mechanisms (1) & (2) associated with Ni2+ or Co2+/3+.41 When the scan rate increases from 2 to 50 mV s−1, the oxidation and reduction peaks shift to higher and lower potentials respectively, and the corresponding peak current increases which is ascribed to the rapid transport of electrons and ions within the electrode.28 According to eqn (3), the estimated Cs value for NiCo2O4 nanoflakes at 2 mV s−1 is 896 F g−1 and it decreases to 677 F g−1 when the scan rate increases to 50 mV s−1. This behavior is obvious at higher scan rates; the electrolyte ion diffusion to inner active sites was limited. In this case, the contribution of CFC is considerably lower and is only ∼13 F g−1, which confirms the predominant pseudo-capacitive characteristics of the material. It was further confirmed by constant current charge–discharge measurements as shown in Fig. 10b. With the current rate NiCo2O4 nanoflakes show a non-linear charge–discharge profile with good symmetry which reveals the dominant pseudo-type capacitive nature of the material. From the discharge curve, Cs was measured using relation (4) and it was found to be 844 F g−1 at a constant current density of 1 A g−1. Further, it retains the maximum Cs of 667 F g−1 at 20 A g−1 as well. This may be attributed to the limited ion transport within the electrode surface due to the self-aggregation between nanoflakes.42 The electrochemical stability and cycle life of the NiCo2O4 nanoflakes were tested by continuous charge–discharge measurements at a constant current density of 20 A g−1 as shown in Fig. 10c. The NiCo2O4 nanoflakes show remarkable durability and they retain the specific capacitance of 527 F g−1 after 2000 cycles (Fig. 10d). The efficiency of the electrode was 79% indicating the superior electrochemical activity of the material. It is mainly due to the desired mesoporous microstructure with reasonable surface area. Additionally, the flexible CFC support tolerates the stress and volume changes associated with fast ion intercalation and de-intercalation during the faradaic reaction.
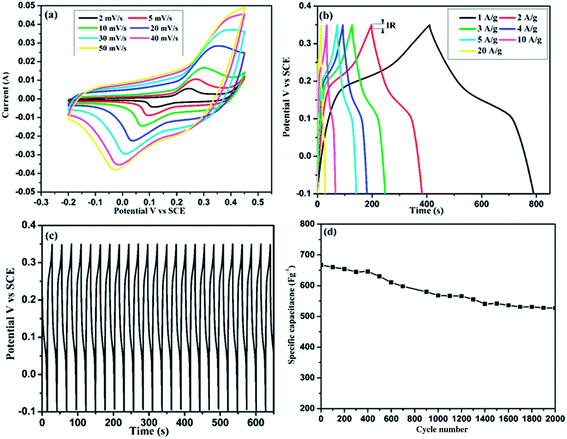 |
| Fig. 10 Electrochemical performances of as-grown self-aggregated NiCo2O4 nanocrystals: (a) CV curves at various scan rates, (b) charge/discharge voltage profiles at various current densities, (c) continuous charge/discharge cycles at the constant current density of 20 A g−1 and (d) the capacitance cycling performance at a constant current density of 20 A g−1. | |
For a better understanding electrochemical impedance spectral (EIS) analysis was performed at the open circuit potential over the frequency range from 1 Hz to 100 kHz. Fig. 11 shows a visible semicircle in the high frequency region and a sloped line at low frequency for both samples. The internal resistance of the electrode material consists of the following terms: the solution resistance Rs, charge transfer resistance Rct, ion diffusion resistance W and the corresponding double layer capacitance Cdl.19,43 The measured charge transfer resistance (Rct) values are 0.75 Ω for the NiCo2O4 nanowall superstructures and 1.25 Ω for the NiCo2O4 nanoflakes. The low Rct value for the nanowall structures may be attributed to the direct bonding between CFC and the ordered open network of NiCo2O4. In the case of the nanoflakes, the reduced electrical conductivity substantiates the weak adhesion of the NiCo2O4 nanoflakes to the CFC substrate. At the mid-frequency region in the EIS spectra, the curved portion followed by the straight line is associated with the electrolyte penetration within the electrode.2 The curved tail in the Nyquist plot can be assigned to a Warberg diffusion process at the CFC||NiCo2O4 interfaces as evidenced by the IR drop in the charge/discharge curves. The results derived from the EIS spectra strongly evidence that the CFC supported NiCo2O4 exhibits superior electrical conductivity which enables rapid ion and electron transport during the redox process.
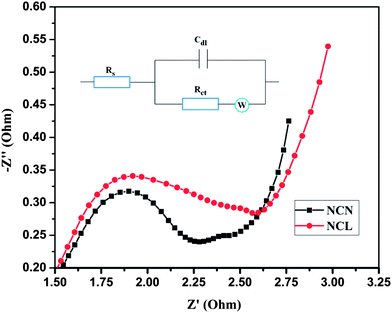 |
| Fig. 11 Electrochemical impedance spectra of NiCo2O4 nanowall structures (black) and self-aggregated nanocrystals (red) and the inset represents the corresponding equivalent circuit. | |
Notably, in this investigation, it is proven that precursors with different anions have great influences on the final morphology of the material. Further, it is confirmed that the precursor driven morphologies exhibit different electrochemical performances when they were used as supercapacitor electrodes. Based on the electrochemical performances it is interesting to highlight the desired structural features of the electrode. The ultra-high specific capacitance and high rate capability of the NiCo2O4 nanowall superstructures may resulted from the following structural advantages: the nanowalls with open-networks provide a large volume for electrolyte ion accumulation by acting as an ion reservoir. Further, the well separated thin nanowall structures enable core active sites for the redox process by reducing the ion diffusion path. In addition, the strong adhesion and mechanical integrity between CFC and the NiCo2O4 nanowalls lead to fast electron transfer even at high current density.38 Similarly, the NiCo2O4 nanoflake microstructures have shown noticeable changes in electrochemical activity due to the following structural discrepancies: well crystalline porous nanoflake microstructures offered only a limited surface area for the redox process. Moreover, the self-aggregation between the nanoflakes decreases the electron and ion transport rate considerably which limits their rate capability when compared to the NiCo2O4 nanowall structures. The weak adhesion with the CFC substrate reduces their electronic conductivity as well as electrochemical activity. However, their unique mesoporous microstructure supported by CFC could be the main reason for the observed electrochemical response of these self-aggregated NiCo2O4 nanoflake structures. From this investigation it could be concluded that precursors with different counter anions also influenced the nanoparticle growth and the internal microstructure such as morphology, crystallite size, surface area and pore size that are directly related to the electrochemical performance of the material. In this study, hydrothermally grown NiCo2O4 nanowall networks could be a potential electrode material for high rate flexible supercapacitors.
Conclusions
In conclusion, we have tuned the morphology of NiCo2O4 nanostructures supported on carbon fiber cloth by using different precursors under hydrothermal conditions. Further we have proved the influence of the precursor on growth kinetics and structure–property relations. Interestingly, based on the precursor, different morphologies and electrochemical activities have been observed for the same material. Well-designed open network NiCo2O4 nanowall structures supported by a CFC backbone endow the electrode with ultra-high specific capacitance and high rate capability. Feasible adhesion to CFC, fast electron transport and high surface area of the NiCo2O4 nanowall architectures have facilitated their superior electrochemical performance as well as admirable cyclic stability. A significant change in electrochemical activity was observed for the NiCo2O4 nanoflake structures which is mainly due to their morphological discrepancies. However, their unique mesorporous microstructure on the CFC substrate and reasonable surface area could be responsible for the observed high specific capacitance and durability. These results demonstrate that the direct anchoring of NiCo2O4@CFC with the desired microstructure could be a superior electrode for high performance flexible supercapacitors.
References
- S. Cheng, L. Yang, Y. Liu, W. Lin, L. Huang, D. Chen, C. P. Wong and M. Liu, J. Mater. Chem. A, 2013, 1, 7709–7716 CAS
. - S. Wang and R. A. W. Dryfe, J. Mater. Chem. A, 2013, 1, 5279–5283 CAS
. - Q. Wang, X. Wang, B. L. Gang, X. Hou, D. Chen and G. Shen, J. Mater. Chem. A, 2013, 1, 2468–2473 CAS
. - Q. Cheng, J. Tang, J. Ma, H. Zhang, N. Shinya and L.-C. Qin, J. Phys. Chem. C, 2011, 115, 23584–23590 CAS
. - L. L. Zhang, Y. Gu and X. S. Zhao, J. Mater. Chem. A, 2013, 1, 9395–9408 CAS
. - K. Juodkazis, J. Juodkazytė, V. Šukienė, A. Grigucevičienė and A. Selskis, J. Solid State Electrochem., 2008, 12, 1399–1404 CrossRef CAS PubMed
. - X. Wu, Y. Zeng, H. Gao, J. Su, J. Liu and Z. Zhu, J. Mater. Chem. A, 2013, 1, 469–472 CAS
. - W. Deng, Y. Liu, Y. Zhang, F. Lu, Q. Chena and X. Ji, RSC Adv., 2012, 2, 1743–1745 RSC
. - S. K. Meher, P. Justin and G. R. Rao, ACS Appl. Mater. Interfaces, 2011, 3, 2063–2073 CAS
. - Y. Huang, Y. Li, Z. Hu, G. Wei, J. Guo and J. Liu, J. Mater. Chem. A, 2013, 1, 9809–9813 CAS
. - L. Y. Chen, J. L. Kang, Y. Hou, P. Liu, T. Fujita, A. Hirata and M. W. Chen, J. Mater. Chem. A, 2013, 1, 9202–9207 CAS
. - L. Hou, C. Yuan, L. Yang, L. Shen, F. Zhang and X. Zhang, RSC Adv., 2011, 1, 1521–1526 RSC
. - X.-H. Xia, J.-P. Tu, X.-L. Wang, C.-D. Gu and X.-B. Zhao, Chem. Commun., 2011, 47, 5786–5788 RSC
. - D. Liu, Q. Wang, L. Qiao, F. Li, D. Wang, Z. Yang and D. He, J. Mater. Chem., 2012, 22, 483–487 RSC
. - H. Jiang, T. Sun, C. Li and J. Ma, J. Mater. Chem., 2012, 22, 2751–2756 RSC
. - S. K. Meher and G. R. Rao, J. Phys. Chem. C, 2011, 115, 25543–25556 CAS
. - R. B. Rakhi, W. Chen, D. Cha and H. N. Alshareef, Nano Lett., 2012, 12, 2559–2567 CrossRef CAS PubMed
. - N. Padmanathan and S. Selladurai, Ionics, 2013, 19, 1535–1544 CrossRef CAS
. - J. Xiao and S. Yang, RSC Adv., 2011, 1, 588–595 RSC
. - H. Jiang, J. Ma and C. Li, Chem. Commun., 2012, 48, 4465–4467 RSC
. - C. Yuan, J. Li, L. Hou, X. Zhang, L. Shen and X. W. (David) Lou, Adv. Funct. Mater., 2012, 22, 4592–4597 CrossRef CAS
. - Z. Cai, L. Li, J. Ren, L. Qiu, H. Lin and H. Peng, J. Mater. Chem. A, 2013, 1, 258–261 CAS
. - G. M. Suppes, B. A. Deore and M. S. Freund, Langmuir, 2008, 24, 1064–1069 CrossRef CAS PubMed
. - S. Biswas and L. T. Drzal, Chem. Mater., 2010, 22, 5667–5671 CrossRef CAS
. - F. Luan, G. Wang, Y. Ling, X. Lu, H. Wang, Y. Tong, X.-X. Liu and Y. Li, Nanoscale, 2013, 5, 7984–7990 RSC
. - Y.-C. Chen, Y.-K. Hsu, Y.-G. Lin, Y.-K. Lin, Y.-Y. Horng, L.-C. Chen and K.-H. Chen, Electrochim. Acta, 2011, 56, 7124–7130 CrossRef CAS PubMed
. - J. Zhao, J. Chen, S. Xu, M. Shao, D. Yan, M. Wei, D. G. Evans and X. Duan, J. Mater. Chem. A, 2013, 1, 8836–8843 CAS
. - G. Zhang and X. W. (David) Lou, Sci. Rep., 2013, 3, 1470 CAS
. - H. Wang and X. Wang, ACS Appl. Mater. Interfaces, 2013, 5, 6255–6260 CAS
. - N. Padmanathan and S. Selladurai, RSC Adv., 2014, 4, 6527–6534 RSC
. - S. K. Meher, P. Justin and G. R. Rao, Nanoscale, 2011, 3, 683–692 RSC
. - Q. Wu, F. Zhang, P. Xiao, H. Tao, X. Wang and Z. Hu, J. Phys. Chem. C, 2008, 112, 17076–17080 CAS
. - H.-W. Wang, Z.-A. Hu, Y.-Q. Chang, Y.-L. Chen, H.-Y. Wu, Z.-Y. Zhang and Y.-Y. Yang, J. Mater. Chem., 2011, 21, 10504–10511 RSC
. - M. Zdrojek, W. Gebicki, C. Jastrzebski, T. Melin and A. Huczko, Solid State Phenom., 2004, 99(265), 1–4 Search PubMed
. - L. Huang, D. Chen, Y. Ding, S. Feng, Z. L. Wang and M. Liu, Nano Lett., 2013, 13, 3135–3139 CrossRef CAS PubMed
. - P. J. Pauzauskie, A. Jamshidi, J. M. Zaug, S. Baker, T. Y.-J. Han, J. H. Satcher Jr and M. C. Wu, Adv. OptoElectron., 2012, 869829 Search PubMed
. - A. Malak-Polaczyk, C. Vix-Guterl and E. Frackowiak, Energy Fuels, 2010, 24, 3346–3351 CrossRef CAS
. - Y. S. Tao, H. Kanoh, L. Abrams and K. Kaneko, Chem. Rev., 2006, 106, 896–910 CrossRef CAS PubMed
. - H. L. Wang, Q. M. Gao and L. Jiang, Small, 2011, 7, 2454–2459 CAS
. - Q. Wang, B. Liu, X. Wang, S. Ran, L. Wang, D. Chen and G. Shen, J. Mater. Chem., 2012, 22, 21647–21653 RSC
. - R. R. Salunkhe, K. Jang, S.-W. Lee and H. Ahn, RSC Adv., 2012, 2, 3190–3193 RSC
. - Y. Q. Wu, X. Y. Chen, P. T. Ji and Q. Q. Zhou, Electrochim. Acta, 2011, 56, 7517–7522 CrossRef CAS PubMed
. - T. Wu, J. Li, L. Hou, C. Yuan, L. Yang and X. Zhang, Electrochim. Acta, 2012, 81, 172–178 CrossRef CAS PubMed
.
|
This journal is © The Royal Society of Chemistry 2014 |
Click here to see how this site uses Cookies. View our privacy policy here.