DOI:
10.1039/C3RA46160B
(Paper)
RSC Adv., 2014,
4, 11244-11250
Imine bond cross-linked poly(ethylene glycol)-block-poly(aspartamide) complex micelle as a carrier to deliver anticancer drugs
Received
28th October 2013
, Accepted 7th January 2014
First published on 8th January 2014
Abstract
The aim of this study is to develop degradable core-cross-linked polymeric micelles based on two water soluble methoxy-poly(ethylene glycol)-block-poly(aspartamide) (mPEG-PA) copolymers using the pH-sensitive imine bond. The ring opening polymerization of N-carboxyanhydride (NCA) was utilized to prepare the diblock copolymer methoxy-poly(ethylene glycol)-block-poly(benzyl L-aspartate). Amino and aldehyde modified mPEG-PAs were obtained by aminolysis and oxidation. The mixture of these two mPEG-PA polymer precursors was shown to form reversible core-cross-linked micelles by the formation of an imine linkage without the addition of cross-linker or catalyst. The structure of the prepared polymers was studied using NMR and FT-IR spectroscopy. The pH-sensitivity of the micelles was characterized by DLS. The mPEG-PA cross-linked micelle exhibited good biocompatibility according to the MTT assay against NIH/3T3 cells. The hydrophobic anticancer drug doxorubicin (DOX) was selected as a model drug and loaded into the micelles where it could be quickly released at low pH and was relatively stable under physiological conditions. Thereby, such an excellent nanocarrier provides a favorable platform to construct smart drug delivery systems for cancer therapy.
Introduction
In the past few decades amphiphilic block copolymers have been extensively studied as supra-molecular templates to design nanoscale devices for anticancer drugs,1–5 as they can self-assemble into polymeric micelles with core–shell architectures above the critical micelle concentration (CMC).6 Such polymeric micelles can improve the bioavailability of therapeutic agents, prolong the circulation time in blood stream and passively targeting the drug based on the enhanced permeability and retention (EPR) effect.7–9 However, polymeric micelles formed by the self-assembly of amphiphilic copolymers are always too fragile to protect the encapsulated drugs while circulating in blood because, once the copolymer concentration falls below the CMC under the diluting condition of blood circulation, the micelles may disassemble into single chains and induce the payload leak out before reaching the diseased site.10 Several strategies have been investigated to increase the stability of the polymeric micelles, including modification of the core-forming block to reduce the CMC,11–14 covalent attachment of hydrophobic drugs15,16 and covalent cross-linking of the micellar core.
Covalent cross-linking of the micellar core can effectively enhance the stability of the micelles and avoid their dissociation in the extreme dilution conditions of blood below the CMC. It may be attained by various strategies including thermal or photo-induced polymerization and using an external cross-linking reagent.17–20 Though these strategies can effectively increase micelle stability, they may cause other problems, such as slow and incomplete drug release within target cells and difficult clearance of the nanocarriers from the body. Therefore, the major challenge for cancer treatment is to develop nanocarriers that are stable under physiological conditions to efficiently retain the encapsulated drug during blood circulation, and can fall apart in a controlled fashion upon stimulation to quickly release the drug in tumor cells. Up to now, various stimuli-responsive cross-linked polymer micelles have been developed for this purpose. Zhao et al. developed a photo-reversible cross-linked nano-gel, prepared by coumarin-containing ABCs at λ > 310 nm and they further demonstrated de-cross-linking by photo induced cleavage at λ < 260 nm, leading to the disintegration of the micelles.21,22 Kakizawa and Heffernan reported the synthesis of polyion complex micelles with a cross-linked core by disulfide bonds.23,24 The cross-linked micelles were expected to be stable in circulation, but dissociated in response to the stronger reducing environment inside the cell. Besides these, cross-linked micelles with acid-cleavable cross-linkers have been intensively investigated owing to the low pH (about 5.0–6.5) in tumor tissues.25–27 These nanocarriers were able to better retain the encapsulated drug and minimized its premature release while circulating in the blood pool and the stability of such nanocarriers was strongly affected by lowering the pH value, leading to release of the encapsulated drugs. However, most of these micelles need the addition of cross-linker.
Imine is a kind of acid-cleavable linkage, which is stable at physiological pH and hydrolyzes under acidic conditions. As a result of this advantage, imine has been extensively studied in medical biotechnology to develop pH triggered controlled release systems since cancer tissues or cells have a slightly acidic pH.28,29 Herein, we report a methoxy-poly(ethylene glycol)-b-poly(L-aspartamide) (mPEG-PA) complex micelle using two amine and aldehyde modified mPEG-PA precursors, which can be reversibly cross-linked by imine bonds. The micelle structure could be stabilized under physiological conditions via the imine bond cross-linking, and disassemble under the acidic conditions via the cleavage of the imine bonds. This system has some advantages: firstly, the two mPEG-PA copolymer precursors are biocompatible and biodegradable, providing the possibility of their applications in biomedical area, secondly, no addition of cross-linker or catalyst was used during the formation of the imine bond core cross-linked micelles, and finally, the two copolymer precursors have good water solubility. The imine bond is the driving force to form the micelles. Compared with other post core cross-linking micelles there are no super hydrophobic blocks in this system. The latter may inhibit the release of the drug at the diseased site. The copolymer precursors can be quickly dispersed in the aqueous solution as the imine bond cleaves, inducing the exhaustive disintegration of the micelles and providing an accelerated drug release. In this article, the hydrophobic anticancer drug doxorubicin (DOX) was encapsulated into mPEG-PA micelles and their performance as a smart drug delivery system was investigated in terms of the stabilization under physiological conditions and in vitro drug release under pH stimulus.
Experimental details
Materials
α-Methoxy poly(ethylene glycol) (mPEG, Mn = 5000) was purchased from Alfa Aesar. L-Aspartic acid was obtained from United Star Biological Co. 3-Amino-1,2-propanediol was purchased from J&K. 1,2-Ethanediamine, tetrahydrofuran(THF), dimethylformamide (DMF), ethyl acetate and petroleum ether were obtained from Tianjin Chemical Reagent Co. and dried by distillation before use. DOX hydrochloride (DOX·HCl) was supplied by Beijing Huafeng United Technology Co.
Measurements
1H NMR spectra were recorded on a Varian UNITY-plus 400 NMR spectrometer using CF3COOD as a solvent. Tetramethylsilane was used as the internal standard. Fourier transform infrared spectra (FT-IR) were measured using a Bio-Rad FTS6000 spectrophotometer at room temperature. Polymer samples were prepared by dispersing the complexes in a KBr powder and compressing the mixtures to form a plate. The apparent molecular weight (Mn) and the molecular weight distribution (Mw/Mn) of the polymers were determined with a gel permeation chromatograph (GPC) equipped with a Hitachi L-2130 HPLC pump, a Hitachi L-2350 column oven operated at 50 °C and a Hitachi L-2490 refractive index detector. DMF was used as the eluent at a flow rate of 1.0 mL min−1. Molecular weights were calibrated on PMMA standards. The morphologies of the core-cross-linked micelles were observed by transmission electron microscopy (TEM). The digital images were recorded on a JEOL microscope (Japan). To prepare the samples, a small drop of a diluted particle dispersion was spread onto a carbon-coated grid. The zeta potential and size distribution measurements of the core-cross-linked micelle were carried out with a Zetasizer nano ZS90 (Malvern Instruments, U.K.). Besides, the reversibility of zeta potential of the micelles was checked by changing the pH of the stock solution to a required value and the solution was incubated using ultrasounds for 15 min and stirring for another 3 h before the next measurement.
Synthesis of methoxy-poly(ethylene glycol)-block-poly(benzyl L-aspartate) (mPEG-PBLA)
mPEG-PBLA was synthesized according to our previously reported work.30,31 Firstly, mPEG-OH was converted into ω-amino-α-methoxy poly(ethylene glycol) (mPEG-NH2) and then mPEG-NH2 was used as the macroinitiator for the N-carboxyanhydride ring opening polymerization (NCA-ROP) of benzyl L-Aspartate NCA (BLA-NCA).32 In general, mPEG-NH2 and BLA-NCA were dissolved in anhydride DMF and stirred at 35 °C under nitrogen atmosphere for 3 days. Subsequently, ethyl ether was added to form a white precipitate of mPEG-PBLA, which was collected and dried under vacuum at 50 °C overnight.
Synthesis of methoxy-poly(ethylene glycol)-block-poly(amino ethyl L-aspartamide) (mPEG-PAmEA)
mPEG-PBLA (0.23 g) was dissolved in anhydride DMF (7.5 mL) at 35 °C. 1,2-Ethanediamine (0.84 g, excess based on benzyl ester units) was added to the solution and the reaction mixture was stirred at 35 °C for one hour under dry nitrogen atmosphere. The resulting solution was added dropwise into a 10% aqueous acetic acid solution (15 mL). Then the solution was dialyzed against a 0.01 M hydrochloric acid solution and distilled in water for two days. The white powder (mPEG-PAmEA) was obtained after lyophilization.
Synthesis of methoxy-poly(ethylene glycol)-block-poly(propanediol L-aspartamide) (mPEG-PPDA)
mPEG-PBLA (0.23 g) was dissolved in DMF (7.5 mL) at 35 °C. 3-Amino-1,2-propanediol (1.37 g, excess based on benzyl ester units) was added to the solution and the reaction mixture was stirred at 35 °C for one hour under a dry nitrogen atmosphere. The resulting solution was dialyzed against distilled water for two days. The white powder (mPEG-PPDA) was obtained after lyophilization.
Synthesis of methoxy-poly(ethylene glycol)-poly(aldehyde ethyl aspartamide) (mPEG-PAlEA)
mPEG-PPDA was dissolved in distilled water and cooled to 0 °C. An excess amount of aqueous solution of NaIO4 (0.33 M, 12.5 equiv. per 1,2-diol group) was added and stirred for 30 min at 0 °C in the dark. Then excess ethylene glycol was added and reacted for another half hour to make NaIO4 react completely. The resulting solution was dialyzed against distilled water for two days. The white powder (mPEG-PAlEA) was obtained after lyophilization.
Synthesis of the core-cross-linked polymer micelles
mPEG-PAmEA and mPEG-PAlEA were dissolved in DMSO with a concentration of 0.5 μg mL−1. A constant flow pump was used to slowly add the mPEG-PAmEA solution dropwise to the mPEG-PAlEA solution (the number of aldehyde groups of mPEG-PAlEA with respect to the number of amino groups of mPEG-PAmEA was 1
:
1). The mixture was stirred for 24 h and after completing the resulting solution was dialyzed against a 0.01 M NaHCO3 aqueous solution (pH = 8–8.5) for two days.33 TEM was utilized to confirm the formation of the core-cross-linked micelles.
Preparation of DOX-loaded core-cross-linked micelles
DOX-loaded micelles were prepared as follows: certain amounts of mPEG-PAlEA and mPEG-PAmEA were dissolved in DMSO. DOX (0.5 equiv. of core-cross-linked micelle) was dissolved in the mPEG-PAlEA solution. Then the mPEG-PAmEA solution was added dropwise to the mPEG-PAlEA solution slowly using a constant flow pump. The resulting solution was dialyzed against 0.01 M PBS (pH = 7.4) for two days. Finally, DOX-loaded core-cross-linked micelles were lyophilized to give a red powder. The DOX content in the core-cross-linked micelles was measured using a UV-visible spectrophotometer by the absorbance at 485 nm in DMSO. The drug loading efficiency (LE%) and entrapment efficiency (EE%) were calculated by the following equations:
in which Wo, We, and Po are the weight of the drug feeding amount, capsulated drug and core-cross-linked micelle with drug, respectively. The drug loading efficiency and entrapment efficiency were calculated to be 12.7% and 52.9%, respectively.
In vitro drug release from the micelles
DOX-loaded core-cross-linked micelles (20 mg) were dispersed in buffer solutions (4 mL) at pH 7.4 (0.01 M PBS) and 5.0 (0.01 M acetate buffer) before being transferred into dialysis bags (3500 Da cutoff), which were immersed in a 20 mL buffer solution at the same pH value. The vials were shaken at a rate of 120 rpm at 37 °C in a shaking bed under sink conditions. At predetermined time intervals, 5 mL of outside release media was taken out for measurement and replaced with an equal volume of fresh buffer solution. The concentration of DOX was measured by a UV-visible spectrophotometer at 485 nm. The cumulative released DOX was calculated as:
where Ve is the amount of release media taken out every time (5 mL), V0 is the amount of release medium (20 mL), Ci is the concentration of DOX released from the micelles at the displacement time of i, mdrug is the mass of drug used for release and n is the displacement time.
In vitro cytotoxicity test of core-cross-linked micelles
NIH/3T3 cells were used to investigate the cytotoxicity of polymers. NIH/3T3 cells were seeded in a 96-well microtiter plate at an initial density of 1 × 104 cells per well in DMEM complete medium and incubated at 37 °C in 5% CO2. After 24 h of incubation at the above conditions, the core-cross-linked micelles were added to the medium at concentrations ranging from 0 to 200 μg mL−1 (0, 10, 50, 100 and 200 μg mL−1). Each dosage was applied to 6 wells. At 48 h of further incubation, the solutions were aspirated and at the end of this period, the medium of each well was added to MTT dye (100 μL, 0.5 mg mL−1 in PBS) and replaced with 150 μL DMSO after 4 h. The absorbance of each well was measured using a microplate reader (Labsystem, Multiskan, Ascent, Model 354 Finland) at 570 nm, and the fraction of live cells was measured. Six replicates were counted for each sample. The mean values were used as the final data.
Results and discussion
Synthesis and characterization of block copolymers
Kataoka and coworkers reported the aminolysis reaction of poly(benzyl L-aspartate) (PBLA) with diamino ethylene and its derivatives under mild conditions, which is of a high aminolysis rate with no effect on the polymer's molecular weight and molecular weight distribution.32,34 Aminolysis reaction provides a convenient approach for the introduction of functional groups to PBLA. Herein, mPEG-NH2 was used as a macro-initiator to synthesize mPEG-PBLA. Then 1,2-ethanediamine and 3-amino-1,2-propanediol were used as aminolysis reagents to synthesise mPEG-PAmEA and mPEG-PPDA, respectively. Finally, mPEG-PAlEA was obtained by reacting mPEG-PPDA with NaIO4, an oxidant. This synthesis procedure is illustrated in Scheme 1.
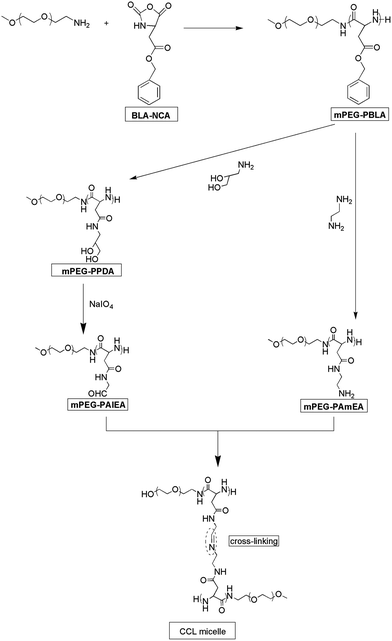 |
| Scheme 1 Synthesis of mPEG-PAmEA, mPEG-PAlEA and core-cross-linked mPEG-PA micelles. | |
The 1H NMR spectra of mPEG-PBLA, mPEG-PAmEA, mPEG-PPDA and mPEG-PAlEA are shown in Fig. 1. The degree of polymerization (DP) was 16 for the PBLA block in mPEG-PBLA, as determined by 1H NMR (Fig. 1a), which is consistent with feedings. The disappearance of the peaks of the benzyl groups at 7.2 and 5.1 ppm confirmed that the aminolysis reaction was successful. The DP of PAmEA and PAlEA are both 16, calculated by comparing the methylene peak of mPEG-NH2 (b) with the methine groups of PAmEA (c in Fig. 1b) and PAlEA (c in Fig. 1d), respectively. GPC of the copolymers, including mPEG-PBLA, mPEG-PAmEA, mPEG-PPDA and mPEG-PAlEA was performed using DMF as the eluent. The results show that the molecular weight of mPEG-PBLA (PDI = 1.3), mPEG-PAmEA (PDI = 1.2), mPEG-PPDA (PDI = 1.2) and mPEG-PAlEA (PDI = 1.2) are 11
500, 10
200, 10
400 and 9800, respectively. All these results indicate that there was no backbone broken during the aminolysis and oxidation.
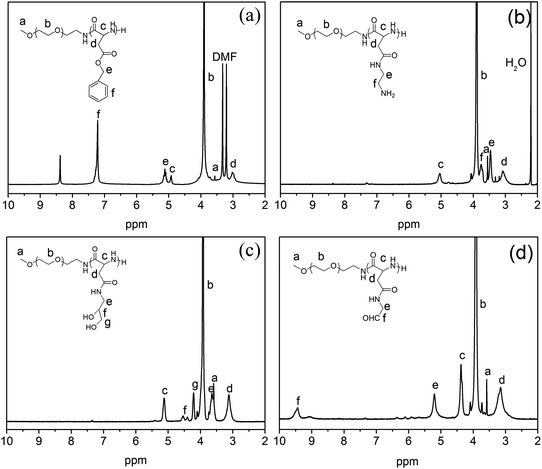 |
| Fig. 1 1H NMR spectra of mPEG-PBLA (a), mPEG-PAmEA (b), mPEG-PPDA (c) and mPEG-PAlEA (d) in CF3COOD. | |
Core-cross-linked mPEG-PA micelles were synthesized through the reaction between the aldehyde of mPEG-PAlEA and the amino of mPEG-PAmEA. The formation of the imine bond was evidenced by the FT-IR spectra. Fig. 2 shows the FT-IR spectra of mPEG-PBLA, mPEG-PAmEA, mPEG-PAlEA and the core-cross-linked mPEG-PA micelles. The disappearance of the benzyl group peak at 1736 cm−1 in Fig. 2b and c confirmed the successful aminolysis reaction. New peak at 1728 cm−1 emerged in Fig. 2c, which corresponds to the stretching of the aldehyde group. It can be observed that the aldehyde stretch peak (1728 cm−1) in mPEG-PAlEA disappeared in the IR spectrum of the mPEG-PA micelles, proving the cross-linking reaction as shown in Scheme 1. Fig. 3 shows the TEM images of an aqueous solution of mPEG-PA micelles at pH 7.4. As shown in Fig. 3, the structure of mPEG-PA micelles is stable and spherically shaped at pH 7.4.
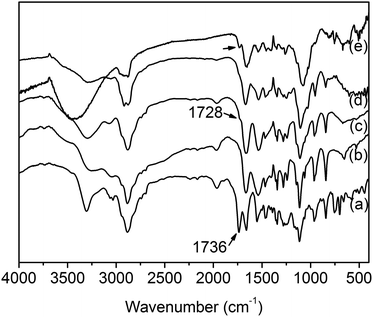 |
| Fig. 2 FT-IR spectra of mPEG-PBLA (a), mPEG-PAmEA (b), mPEG-PAlEA (c), the mPEG-PA micelle at pH 7.4 (d) and pH 5.0 (e). | |
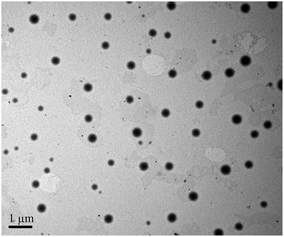 |
| Fig. 3 TEM images of mPEG-PA micelle at pH 7.4. | |
Property of the core-cross-linked micelles in response to pH
Fig. 4 shows the zeta potential of mPEG-PA micelles at different pHs. As discussed before, mPEG-PA core-cross-linked micelles are stable at pH 7.4. Once the environmental pH is decreased to mildly acidic, imine bonds start to cleave and induce the disaggregation of the core-cross-linked micelles. The zeta potential of the core-cross-linked micelles increases with the decrease of environment pH due to the release of amino groups on mPEG-PAmEA. As shown in Fig. 4, the zeta potential is 0 mV at pH 6.4, where the imine bonds start to cleave.
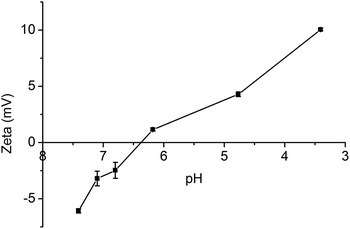 |
| Fig. 4 Zeta potential of the mPEG-PA micelle at different pHs. | |
In order to support this hypothesis, the IR spectrum of mPEG-PA micelles at pH 5.0 is shown in Fig. 2e. As shown in Fig. 2e, once the environmental pH was decreased to acidic, the aldehyde stretch peak (∼1728 cm−1) reappeared due to the release of aldehyde groups on mPEG-PAlEA during the cleavage of the imine bonds.
Fig. 5 shows the time dependence of the average hydrodynamic diameter of mPEG-PA micelles at different pH values. The hydrodynamic size of the polymer micelles at pH 7.4 showed negligible changes over time, suggesting core-cross-linked micelles. However, at pH 5.0, the hydrodynamic size of the mPEG-PA micelles changed from 250 nm to 900 nm in 60 min. This is because the lower pH causes the cleavage of the imine bond and induces the swelling and eventually the disaggregation of the nanoparticles. Corresponding size distributions of micelles at pH 5.0 are given in Fig. 6 to support the process of particle changes. It is worth noting that the disintegration of the micelles without hydrophobic blocks will provide an accelerated drug release at the diseased site.
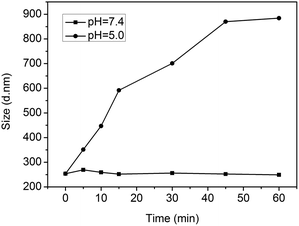 |
| Fig. 5 Time dependence of the average hydrodynamic diameter of mPEG-PA micelles at different pH values. | |
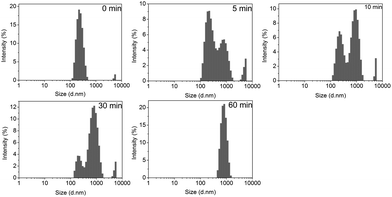 |
| Fig. 6 The size distribution of mPEG-PA micelles versus time at pH 5.0. | |
In vitro drug release
As described above, the acid-cleavable imine linkage can be used in the reversible formation of mPEG-PA micelles and is expected to trigger the release of a model anticancer drug, DOX. The in vitro drug release of DOX from the loaded particles was carried out under a simulated physiological environment (pH 7.4) and in an acidic endosomal condition (pH 5.0) at 37 °C. As shown in Fig. 7, the DOX loaded mPEG-PA complex particles exhibit a well-defined pH-dependent drug release behavior, while at pH 7.4, the drug release rate was relatively slow and slowed down after 3 h with an initial burst release of about 10%. The cumulated release amount was less than 24% after 60 h. On the contrary, the release of nanoparticles in pH 5.0 buffer solutions was much faster. After 60 h, more than 60% of the drug was released at pH 5.0.
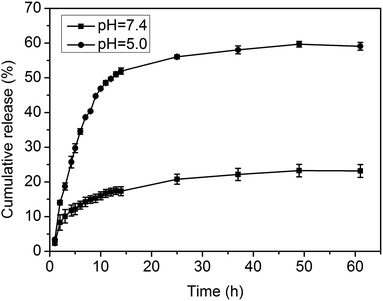 |
| Fig. 7 Release profiles of DOX from mPEG-PA micelles at pH 5.0 and 7.4 at 37 °C. | |
The overall drug release behavior can be described as: at neutral pH, micelles are reversibly cross-linked by imine bonds, which makes it hard for the drug to diffuse out and thus minimizes premature drug release in the blood stream. However, when the environmental pH is lower, core-cross-linked micelles disassemble because of the cleavage of the imine bond, which makes the copolymer dissolve in water and releases DOX molecules. Therefore, using this nanocarrier, drug release could be activated by the acidic conditions in the target cells after reaching the tumor sites.
DOX release kinetics
The Korsmeyer–Peppas semi-empirical equation was used to study the mechanism of DOX release from mPEG-PA micelles:35
where Qt/Qe is the drug fraction released at time t, and kKP and n are the constants corresponding to the structural and the kinetic exponents, which areindicative of the mechanism of drug release, respectively. Although the use of this equation requires detailed statistical analysis, the calculated exponent n gives an indication of the release kinetics. According to the equation for the initial several hours of drug release under various conditions, the correlation coefficient (R2) is higher than 0.99, while the kinetic exponent at pH 7.4 and 5.0 are 0.39 and 0.73, respectively. This indicates that the kinetics of DOX release is a typical Fickian diffusion at pH 7.4. While at pH 5.0, the release of DOX is a random diffusion controlling kinetic, which is combined with the Fickian diffusion and polymer chain loosening. Polymer chain loosening is caused by the imine dissociating at lower pH, leading to cross-linkage break and micelle structure disintegration.
Biocompatibility evaluation of the core-cross-linked micelles
An in vitro cytotoxicity test (MTT assay) was performed using NIH/3T3 cells to evaluate the biocompatibility of mPEG-PA core-cross-linked micelles, and the corresponding results are shown in Fig. 8. The cell viability was compared with control cells that had been incubated in a culture dish without polymer micelles. The cytotoxicity of mPEG-PA core-cross-linked micelles towards NIH/3T3 cells was evaluated at concentrations ranging from 10 to 200 μg mL−1. Polymeric micelles did not show significant cytotoxicity against NIH/3T3 cells. Even at a high concentration (200 μg mL−1), the core-cross-linked micelles exhibited a good biocompatibility after a 48 h incubation. This is associated with the good hydrophilicity of the nanoparticles. Both of the PEG and poly(amino acid) segments are hydrophilic in this system. They may retain more water so that a favourable environment can be maintained for cell proliferation. In addition, it has been reported that a polypeptide with many amino acid units is an excellent source of nitrogen to substantially improve cell viability.36 All these results suggest that core-cross-linked micelles have low cytotoxicity and have potential for biomedical applications. However, it should be noted that this is an in vitro system, a proof-of-concept for a potential useful approach in drug delivery, whose application in real clinical settings will depend on a deeper assessment of their toxicity and immunoreactivity.
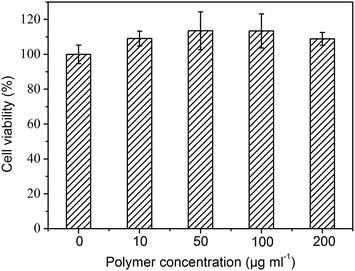 |
| Fig. 8 In vitro cytotoxicity of the core-cross-linked micelles to NIH/3T3 cells. | |
Conclusion
In this study, we demonstrated biodegradable and biocompatible pH-triggered disaggregated core-cross-linked micelles with potential applications in antitumor drug delivery. The micelles were prepared from two mPEG-PA block copolymers, mPEG-PAmEA and mPEG-PAlEA, which were modified by amine and aldehyde groups, respectively. The process of self-association of the block copolymers into core–shell micelles was followed by the cross-linking of the core through imine bonds. DOX as a model drug was loaded into the nanocarriers. The particles possessed a good pH-responsive drug release behavior. Under physiological conditions, the drug loaded core-cross-linked micelles showed strong stability and a small encapsulated drug release. Fast drug release can be achieved under acidic environment conditions due to breakdown of the imine linkages. These nanoparticles are promising for tumor targeted drug delivery.
Acknowledgements
This work was funded by NSFC (no. 51203079), PCSIRT (IRT1257) and the Ph.D. Programs Foundation for New Teachers of Education Ministry of China (no. 20090031120012).
References
- A. Rösler, G. W. M. Vandermeulen and H.-A. Klok, Adv. Drug Delivery Rev., 2001, 53, 95–108 CrossRef.
- S. V. Vinogradov, Curr. Pharm. Des., 2006, 12, 4703–4712 CrossRef CAS.
- G. Riess, Prog. Polym. Sci., 2003, 28, 1107–1170 CrossRef CAS.
- A. Carlsen and S. Lecommandoux, Curr. Opin. Colloid Interface Sci., 2009, 14, 329–339 CrossRef CAS PubMed.
- V. Torchilin, Eur. J. Pharm. Biopharm., 2009, 71, 431–444 CrossRef CAS PubMed.
- S. M. Garg, X.-B. Xiong, C. Lu and A. Lavasanifar, Macromolecules, 2011, 44, 2058–2066 CrossRef CAS.
- G. K. Such, E. Tjipto, A. Postma, A. P. R. Johnston and F. Caruso, Nano Lett., 2007, 7, 1706–1710 CrossRef CAS PubMed.
- H. Suzuki, D. Nakai, T. Seita and Y. Sugiyama, Adv. Drug Delivery Rev., 1996, 19, 335–357 CrossRef CAS.
- J. O. Kim, G. Sahay, A. V. Kabanov and T. K. Bronich, Biomacromolecules, 2010, 11, 919–926 CrossRef CAS PubMed.
- T. K. Bronich, P. A. Keifer, L. S. Shlyakhtenko and A. V. Kabanov, J. Am. Chem. Soc., 2005, 127, 8236–8237 CrossRef CAS PubMed.
- M. L. Adams, D. R. Andes and G. S. Kwon, Biomacromolecules, 2003, 4, 750–757 CrossRef CAS PubMed.
- K. K. Jette, D. Law, E. A. Schmitt and G. S. Kwon, Pharm. Res., 2004, 21, 1184–1191 CrossRef CAS.
- P. Opanasopit, M. Yokoyama, M. Watanabe, K. Kawano, Y. Maitani and T. Okano, Pharm. Res., 2004, 21, 2001–2008 CrossRef CAS.
- M. L. Adams and G. S. Kwon, J. Controlled Release, 2003, 87, 23–32 CrossRef CAS.
- Y. Bae and K. Kataoka, Adv. Drug Delivery Rev., 2009, 61, 768–784 CrossRef CAS PubMed.
- L. Zhou, R. Cheng, H. Tao, S. Ma, W. Guo, F. Meng, H. Liu, Z. Liu and Z. Zhong, Biomacromolecules, 2011, 12, 1460–1467 CrossRef CAS PubMed.
- V. Schmidt, R. Borsali and C. Giacomelli, Langmuir, 2009, 25, 13361–13367 CrossRef CAS PubMed.
- J. S. Kim and J. H. Youk, Polymer, 2009, 50, 2204–2208 CrossRef CAS PubMed.
- X. Xu, J. D. Flores and C. L. McCormick, Macromolecules, 2011, 44, 1327–1334 CrossRef CAS.
- C. Chang, H. Wei, D. Q. Wu, B. Yang, N. Chen, S. X. Cheng, X. Z. Zhang and R. X. Zhuo, Int. J. Pharm., 2011, 420, 333–340 CrossRef CAS PubMed.
- J. Jiang, B. Qi, M. Lepage and Y. Zhao, Macromolecules, 2007, 40, 790–792 CrossRef CAS.
- J. He, X. Tong and Y. Zhao, Macromolecules, 2009, 42, 4845–4852 CrossRef CAS.
- Y. Kakizawa, A. Harada and K. Kataoka, J. Am. Chem. Soc., 1999, 121, 11247–11248 CrossRef CAS.
- M. J. Heffernan and N. Murthy, Ann. Biomed. Eng., 2009, 37, 1993–2002 CrossRef PubMed.
- Y. Chan, T. Wong, F. Byrne, M. Kavallaris and V. Bulmus, Biomacromolecules, 2008, 9, 1826–1836 CrossRef CAS PubMed.
- S. J. Lee, K. H. Min, H. J. Lee, A. N. Koo, H. P. Rim, B. J. Jeon, S. Y. Jeong, J. S. Heo and S. C. Lee, Biomacromolecules, 2011, 12, 1224–1233 CrossRef CAS PubMed.
- V. T. Huynh, S. Binauld, P. L. de Souza and M. H. Stenzel, Chem. Mater., 2012, 24, 3197–3211 CrossRef CAS.
- L. Meng, W. Huang, D. Wang, X. Huang, X. Zhu and D. Yan, Biomacromolecules, 2013, 14, 2601–2610 CrossRef CAS PubMed.
- N. Abdullah Al, J. A. Nam, H. Mok, Y.-k. Lee and S. Y. Park, Macromol. Res., 2012, 21, 92–99 CrossRef.
- G. L. Wu, Z. Wang, H. Lin, J. Zhang, P. C. Sun, G. L. Zhang, Y. N. Wang and J. B. A. Ma, Acta Polym. Sin., 2009, 1232–1237 CrossRef CAS.
- S. Yu, G. Wu, X. Gu, J. Wang, Y. Wang, H. Gao and J. Ma, Colloids Surf., B, 2013, 103, 15–22 CrossRef CAS PubMed.
- A. Koide, A. Kishimura, K. Osada, W.-D. Jang, Y. Yamasaki and K. Kataoka, J. Am. Chem. Soc., 2006, 128, 5988–5989 CrossRef CAS PubMed.
- C. Ding, J. Gu, X. Qu and Z. Yang, Bioconjugate Chem., 2009, 20, 1163–1170 CrossRef CAS PubMed.
- S. Fukushima, K. Miyata, N. Nishiyama, N. Kanayama, Y. Yamasaki and K. Kataoka, J. Am. Chem. Soc., 2005, 127, 2810–2811 CrossRef CAS PubMed.
- M. A. Malana and R. Zohra, DARU Journal of Pharmaceutical Sciences, 2013, 21, 10 CrossRef CAS PubMed.
- M. Obst and A. Steinbüchel, Biomacromolecules, 2004, 5, 1166–1176 CrossRef CAS PubMed.
|
This journal is © The Royal Society of Chemistry 2014 |