DOI:
10.1039/C3RA45938A
(Paper)
RSC Adv., 2014,
4, 3340-3347
Interactions between Th(IV) and graphene oxide: experimental and density functional theoretical investigations†
Received
19th October 2013
, Accepted 23rd October 2013
First published on 25th October 2013
Abstract
Graphene oxide (GO) has been receiving increasing research efforts in recent years because of its wide applications in various scientific fields. In this work the sorption of Th(IV) onto graphene oxide (GO) was studied using a batch method under ambient conditions. The sorption kinetics were found to be fast and fitted the pseudo-second-order model very well, with an equilibrium time of about 10 min. The sorption is strongly dependent on the solution pH but independent of the ionic strength of the solution. The maximum sorption capacity of as high as 214.6 mg g−1 can be achieved at pH 2.60 ± 0.05, and Th(IV) can be desorbed readily from the GO with 1.0 M HNO3. The thermodynamic investigations revealed that the sorption of Th(IV) on the GO was an endothermic and spontaneous process. The Scanning Electron Microscopy (SEM) results indicated obvious surface morphology changes of the GO induced by Th(IV) sorption. Furthermore, the interaction mechanism of Th(IV) and the GO was investigated by infrared (IR) spectroscopy and extended X-ray absorption fine structure (EXAFS) spectroscopy combined with density functional theory (DFT) calculations. The results of EXAFS indicated that Th(IV) was bonded to ∼8 or 9 oxygen atoms and the average bond length of Th–O was estimated to be ∼2.45 Å in the first coordination shell. The DFT calculations further confirm the rationality of experimental and the EXAFS results. This work demonstrates the tremendous potential opportunities offered by GO in pre-concentration and removal of thorium and other tetravalent actinides for the recovery and remediation of the environment.
Introduction
Nuclear energy is currently undergoing a rapid development and attracting wide research efforts because of the increasing energy demand. It has also been regarded as a reasonable solution towards global climate change and the shortage of fossil fuels. In the meanwhile, large amounts of radioactive wastes are worrisomely released into the environment due to nuclear fuel cycle and mining operations, which would impose a long-term threat to the surface and subsurface environment.1 Hence, the removal of long-lived toxic radionuclides is an important environmental concern. There have been many researches concerning the sorption of uranium by different materials.2–7 However, thorium, as a potential nuclear resource, is attracting more and more attention due to the shortage of uranium in the near future. Highly efficient immobilization and removal of thorium from water bodies would be of extreme importance from point of view of environmental remediation and full utilization of thorium resources. On the other hand, Th(IV) is usually regarded as an analog of other tetravalent actinides, studying the sorption and migration behaviors of Th(IV) instead of other much more radioactive tetravalent actinides can provide useful information for understanding their migration mechanisms in surface and subsurface environments.
The sorption behaviors and interaction mechanisms of the solid–liquid phase interface of Th(IV) with various traditional environment-associated materials, such as hematite,8 bentonite,9 zeolites10 and muscovite,11 etc., have been extensively studied. For instance, Sharma and co-workers10 studied the sorption behavior of Th(IV) on zeolite, and found that the sorption capacity increased largely with increasing solution pH and temperature. Pan et al.9 found that K+, Mg2+ and Ca2+ obviously restrained Th(IV) sorption on bentonite; the influences of anions such as NO3−, ClO4− and Br− are negligible, whereas F− and PO43− can dramatically enhance the Th(IV) sorption. However, for high enrichment of Th(IV) and environmental remediation, the above mentioned materials usually suffer from either low sorption capacity or efficiency, which limit their applications for the effective removal of Th(IV) from aqueous solutions.
Carbon materials normally have relatively excellent thermal, radiation and chemical stability compared to organic exchange resins and other inorganic sorbents. Another merit of carbon materials in actinide pre-concentration is that the sorption and desorption of metal ions could be readily performed and carbon materials themselves can be fully incinerated, which makes them suitable for environmental remediation. Graphene oxide (GO), the oxidized graphene sheets, is a typical carbon material with outstanding performance. It contains abundant oxygen-containing functional groups on the surface, and has been considered to be an excellent material to remove heavy metal ions from aqueous solutions due to its eminent sorption properties. Sun et al.,12 for example, pointed out that the sorption capacity of Eu(III) onto the GO at pH 4.5 and 6.0 was as high as 161.29 mg g−1 and 175.44 mg g−1, respectively. Recently, we found that the maximum sorption capacity of U(VI) onto the GO reached 299 mg g−1 at pH 4.0.13 Additionally, there were some reports about the sorption of heavy metal ions with graphene composite materials.14–17 However, to the best of our knowledge, the investigation about the sorption of Th(IV) onto the GO is still lacking. It is well known that the chemical properties of thorium and uranyl ions are quite different. Uranyl ions are linear and oxo group containing whereas thorium cations are bare ions and more positively charged. Also, Th(IV) is more tended towards hydrolysis at a lower pH than U(VI). So the sorption property of Th(IV) on GO may be very different from that of U(VI).
Keeping this in mind, we systematically studied the sorption behaviors of Th(IV) on GO in this work. The objectives of this work were to (1) investigate the effect of solution pH, contact time, initial Th(IV) concentration, ionic strength, solid-to-liquid ratio (ms/VGO) for Th(IV) sorption on the GO using a batch method; (2) investigate the desorption and regenerating performance of the GO; (3) discuss the mechanism of the interaction between Th(IV) and the GO using Th LIII-edge EXAFS spectroscopy and FTIR; (4) provide insights into the sorption process and bonding nature of the sorption complexes by density functional theory (DFT) calculations in order to further confirm the rationality of experimental and the EXAFS results. Our results indicate the tremendous potential opportunities of GO in preconcentration and cleanup of thorium and other tetravalent actinides.
Experimental
Chemicals and reagents
Th(NO3)4·5H2O was obtained from Merck Co. The stock solution of Th(IV) was prepared by dissolving appropriate amounts of Th(NO3)4·5H2O in nitric acid solution for inhibition of the hydrolysis of Th(IV). All other chemicals were of analytical grade or better and used without further purification. All testing solutions were prepared with Milli-Q water.
Synthesis and characterization of GO
Graphene oxide was prepared from natural graphite powder (Alfa Aesar, >99.9995%) by the Hummers method using NaNO3, H2SO4, and KMnO4,18 and characterized by atomic force microscopy (AFM), scanning electron microscopy (SEM), UV-Vis absorption spectroscopy (UV-Vis), Fourier transform infrared spectroscopy (FT-IR), X-ray photoelectron spectroscopy (XPS), and Raman spectroscopy. The detailed methods of GO preparation and characterization have been described in our previous work.13
Sorption and desorption experiments
All sorption experiments were carried out using a batch method in air at 293 K except for the thermodynamic study. The solution pH was adjusted by using negligible volumes of HNO3 or NaOH. After shaking for 2 h to achieve sorption equilibrium, the solid phase was separated from the solution by using a 0.45 μm nylon membrane filter. The concentration of thorium in the filtrate was determined by UV-Visible spectrometry using arsenazo-III as the chromogenic agent. Reference experiments in the absence of GO were carried out simultaneously to evaluate the possible sorption of thorium onto the glass vessel wall and the filter membrane, which was demonstrated to be negligible.
In desorption experiment, after stirring the samples with different concentrations of HNO3 for 2 h, the desorption equilibrium was obtained, and then the solid phase was separated from the solution by filtration with a 0.45 μm nylon filter membrane. Subsequently, the Th(IV) concentration in filtrate was determined. Desorption efficiency is calculated from the following equation:
|
 | (1) |
In the resorption experiments, the experimental conditions were kept the same as in the previous sorption experiments with fresh sorbents.
EXAFS analysis
Th LIII-edge fluorescence measurements were performed at the Beijing Synchrotron Radiation Facility using a lytle-type ion chamber detector. EXAFS data analysis was performed by using Athena and Artemis interfaces to IFFEFIT 7.0 software. EXAFS samples preparation and analysis of the data are described in detail in the ESI (S-6†).
Theoretical methods of DFT calculations
Density functional theory (DFT) calculations were carried out with Gaussian 09
19 at the B3LYP level of theory.20–22 The adopted small-core RECPs include 60 electrons in the core for Th, while for the light atoms C, H, O, and N, the polarized all-electron 6-31G(d) basis sets were used for geometry optimizations. Based on the optimized structures, Gibbs free energies (Gg) were calculated with zero-point-energy (ZPE) and thermal corrections at the B3LYP/6-311G(d,p)/RECP level of theory in the gas phase (298.15 K, 0.1 MPa). The solvation Gibbs free energies (Gsol) were gained by addressing bulk solvation effects with the SMD continuum solvation model23 with the UAKS (united atom topological model) atomic radii at the B3LYP/6-311G(d, p)/RECP level of theory. All solution phase calculations were carried out in water. It has been found that continuum solvation models could produce reliable free energies in solution for the actinide species with the first coordination sphere treated explicitly.24 The Mayer bond orders (MBO) and natural population analysis (NPA)25–27 were carried out at the same level of theory.
Results and discussion
General conditions of Th(IV) sorption on GO
To investigate the Th(IV) sorption properties on GO, we initially tested the general sorption conditions, such as solution pH, contact time, ionic strength and solid-to-liquid ratio. The solution pH is thought to be a significant factor affecting the metal ions’ sorption on the sorbent, because it obviously influences the species of metal ions,28 together with the surface charge and the active sites of the sorbent in the solution. Herein, the Th(IV) sorption by GO at pH ranging from 1.0 to 4.0 was performed to assess the effect of solution pH. The pH value was limited to within 4.0 in case the soluble form of Th(IV) ions would be converted into insoluble species according to solubility calculations.28 In the study of the pH range, we found that the sorption percent increases rapidly with increasing the solution pH and reaches ∼100% at pH 4.0 (Fig. 1A). The sorption kinetics is rapid and a contact time of 10 min is enough to reach the equilibrium (Fig. 1B). The sorption of Th(IV) onto GO fits the pseudo-second-order kinetic model better than the pseudo-first-order kinetic model (ESI, Fig. S4 and Table S1†). The ionic strength has no obvious effect on the Th(IV) sorption on GO under our experimental conditions (Fig. 1C). The strong pH dependent and ionic strength independent sorption of Th(IV) on GO indicate that the sorption mechanism is mainly dominated by the inner-sphere surface complexation rather than ion exchange. With the solid-to-liquid ratio increasing from 0.1 g L−1 to 0.5 g L−1, the Th(IV) sorption percent increases from ∼20% to ∼75%, which is due to the increased absolute binding sites of the sorbent. Moreover, a higher removal percent can be obtained by increasing the GO mass appropriately from the trend of the graph (ESI, Fig. S5†).
 |
| Fig. 1 Adsorption of Th(IV) on GO. (A) Effect of pH. C0(Th) = 120 mg L−1, CGO = 0.36 g L−1, and t = 240 min. (B) Effect of contact time and the pseudo-second-order kinetic model (insert graph). C0(Th) = 120 mg L−1, CGO = 0.36 g L−1, and pH = 2.60 ± 0.05. (C) The effect of ionic strength. C0(Th) = 120 mg L−1, CGO = 0.36 g L−1, and pH = 2.60 ± 0.05. (D) Sorption isotherm. CGO = 0.36 g L−1, and pH = 2.60 ± 0.05. The solid curve represents the fitting by a Langmuir isotherm model and the dashed line represents the fitting by a Freundlich isotherm model. | |
Sorption isotherm
The sorption isotherm experiment was carried out with the initial concentrations of Th(IV) varied from 5 to 150 mg L−1. As one can see in Fig. 1D, the sorption of Th(IV) on GO has a sharp increase when the Ce (Ce is the concentration of Th(IV) in solution at equilibrium (mg L−1)) is below 5 mg L−1, then the curve reaches a plateau when Ce is over 20 mg g−1, although qe (qe is the quantity of the sorbed Th(IV) at equilibrium (mg g−1)) still slightly increases with the augmentation of Ce . As a result, a saturation sorption capacity of ∼210 mg g−1 can be determined through curve fitting.
To investigate the sorption mode of Th(IV) on the GO, the Langmuir and Freundlich isothermal models were both used to fit the equilibrium data. The constants of the two models from curve fitting are listed in the ESI (Table S2†). The high correlation coefficients (R2 > 0.99) for both models suggest that the both isotherms fit the sorption data very well. Hence, it is very likely that the sorption of Th(IV) cations mainly occurs in a monolayered pattern on the surface of the GO. The higher value of kF (kF is the Freundlich constant related to the sorption capacity) indicates that the GO has a higher sorption capacity and affinity for Th(IV). The relatively bigger kL (kL is a Langmuir constant indirectly related to sorption capacity and energy of sorption (L mg−1)) and n (n is the Freundlich constant related to the sorption intensity) indicate the strong bonding of Th(IV) with the sorbent. The maximum sorption capacity obtained from the Langmuir fitting is 214.6 mg g−1, which is much higher than other carbon materials, such as 21.28 mg g−1 for activated carbon29 and 13.6 mg g−1 for oxidized multi-wall carbon nanotubes.30 For comparison with other sorbents, some results of Th(IV) sorption by various materials are listed in ESI (Table S3†). As one can see, the performance of the GO is quite competitive, ranking it among the most effective sorbents for Th(IV) removal even at lower pH. The huge sorption capacity of the GO might be due to the abundant oxygen containing functional groups on the surface of the GO. Moreover, GO is much easier to prepare than the chelator–integrated composite materials and has more excellent acid-resistant properties than sorbents like chitosan and zeolite.31 All of these reveal that as a sorbent, GO is more acid-resistant and could be a very promising material to remove Th(IV) cations from aqueous solutions.
Thermodynamic data
The effect of temperature on the GO sorption for Th(IV) was tested at five different temperatures of 293, 303, 313, 323 and 343 K using a water bath. The results are displayed in Fig. 2. As one can see, the equilibrium sorption capacity of Th(IV) increases with increasing the temperature, which suggests that higher temperatures would be more favorable for Th(IV) sorption on GO.
 |
| Fig. 2 The effect of temperature on the Th(IV) sorption and lnKd as a function of 1/T. C0(Th) = 120 mg L−1, CGO = 0.36 g L−1 and pH = 2.60 ± 0.05. | |
To investigate the nature of the sorption process, the changes of the standard Gibbs free energy (ΔG0), standard enthalpy (ΔH0) and entropy (ΔS0) were calculated from the experimental data. ΔH0 and ΔS0 can be calculated from the linear curve of lnKd (Kd is the distribution coefficient (ml g−1) of Th(IV) in sorbent and in solution) as a function of 1/T (Fig. 2) by using the eqn S5† and ΔG0 can be calculated by eqn S6† listed in the ESI. The negative values of ΔG0 (−19.82, −21.39, −22.97, −24.54, −27.68 kJ mol−1 at 293, 303, 313, 323 and 343 K, respectively) indicate that the sorption of Th(IV) on the GO is a spontaneous process. In addition, the fact that ΔG0 decreases with increasing the temperature indicates that the higher temperature would be more favorable for the Th(IV) sorption. The positive value of ΔH0 (26.25 kJ mol−1) indicates that the sorption of Th(IV) on the GO could be an endothermic process. Furthermore, the positive value of ΔS0 (157.24 J (mol−1 K−1)) reveals an increase of randomness for the Th(IV) ions sorption onto the active sites of the sorbent.
Reusability of the sorbent
The reusability is an important consideration when evaluating the economy and applicability of sorbents. Hence, the desorption and resorption behaviors of the GO were studied. The desorption experiments were performed with 0.1, 0.5 and 1.0 M HNO3 in this work, and the desorption efficiencies attained are ∼68%, ∼91% and ∼97%, respectively, which implies that nearly all the Th(IV) adsorbed onto the GO could be completely desorbed with 1.0 M HNO3.
In the resorption experiments, the sorption properties of the reclaimed GO desorbed with 1.0 M HNO3 were compared with the fresh GO. Based on several parallel experiments, a high sorption capacity was achieved with the reclaimed GO and reached up to ∼90% compared to the fresh GO, the small difference might be mainly due to the adsorbed Th(IV) could not be entirely desorbed and the surface properties of the GO which might change to some extent. Nevertheless, the high sorption capacity of the reclaimed GO still demonstrates the regenerability and reusability of the GO.
FT-IR analysis
The FT-IR spectra of different GO samples are shown in Fig. 3. For all samples, the strong peak at a wavenumber of 1740 cm−1 can be assigned to the C
O stretching vibration of the carbonyl or carboxyl groups, the peak at 1620 cm−1 is attributed to the vibration of C
C from the graphene framework, the peak at 1380 cm−1 represents the bending vibration of C–OH groups, the 1220 and 1050 cm−1 peaks can be assigned to the C–O stretching.32 All of the related peaks of the GO reveal that the natural graphite has been oxidized successfully and large amounts of oxygen-containing functional groups are available on the surface of the GO.
 |
| Fig. 3 The infrared spectra of different GO samples. Conditions: a, sorption with pH = 2.0 and C0(Th) = 120 mg L−1; b, sorption with pH = 3.0 and C0(Th) = 120 mg L−1; c, sorption with pH = 3.0 and C0(Th) = 240 mg L−1; d, desorption with 1.0 M HNO3; e, fresh GO. | |
In comparison with the spectrum of the fresh GO (Fig. 3e), the changes of the peaks at 1740 cm−1, 1380 cm−1 and 1220 cm−1 (Fig. 3a–3c) suggest that the interactions between GO and Th(IV) could change the vibrational mode of the C
O and C–OH groups. Furthermore, with the increase of Th(IV) sorption on the GO, the intensity of the peak at 1740 cm−1 decreases gradually, as shown in Fig. 3a–c. One also can see the spectrum of the reclaimed GO (Fig. 3d) remains nearly unchanged compared to the fresh GO, which implies that the functional groups of GO are not destroyed after the sorption and desorption process and the GO has excellent regeneration abilities.
Scanning Electron Microscope (SEM) measurements
To investigate the sorption-induced morphology changes of the GO, SEM measurements were performed. The images of different GO samples are shown in Fig. 4. To avoid the influence of other factors, we kept all the experimental conditions identical. Namely, the solution pH was kept as 2.60 ± 0.05 and the stirring time was 2 h for all the GO samples. The SEM image of the fresh GO (Fig. 4A) shows that thin and slightly crumpled sheets are available on the GO surface, which demonstrates the formation of individual GO sheets. After Th(IV) ions are adsorbed onto the GO surface, protuberant folds can be formed (Fig. 4B), which might be due to the conglomeration of Th(IV) on the crumpled sheets of the GO surface. To identify whether this is the case, a reference SEM sample in the absence of Th(IV) was carefully prepared. The experimental conditions for the reference sample were identical to other samples, except that, in the reference sample, the GO was equilibrated with equal molar concentration of NaNO3 (0.517 mM) instead of Th(IV) solution. The SEM image of the reference sample is shown in Fig. 4C. We can see that it nearly has no change compared to the fresh GO (Fig. 4A). Fig. 4D presents a high resolution image of the flat area in Fig. 4B (the circled area) and we can observe small particles that have emerged on the GO surface, which looks obviously different to the image of the fresh GO. Additionally, we tried to find areas with similar surface morphology as the GO (Fig. 4A) in Fig. 4B but failed. Hence, we can safely conclude that the presence of Na+ cannot change the morphology of the GO surface, but the sorption of Th(IV) can induce protuberant folds of the GO. To the best of our knowledge, this is the first direct observation of the morphological changes of GO induced by metal ion sorption.
 |
| Fig. 4 SEM images. (A) fresh GO; (B) Th(IV)-loaded GO; (C) fresh GO equilibrated with 0.517 mM NaNO3 for 2 h (the same conditions with Th(IV) sorption); and (D) the magnification of the image (B). | |
EXAFS analysis
EXAFS spectroscopy has been widely used to confirm the interaction mechanisms between heavy metal ions and solid phases at the molecular level.33–36 From this element-specific short-range structural and chemical information such as bond distance, coordination number, and type of near neighbors can be obtained. Herein, the Th LIII-edge k3-weighted EXAFS spectra, χ(k), and the corresponding Fourier transformed (FT) radial distribution functions are shown in Fig. 5.
 |
| Fig. 5 (A) Th LIII-edge k3-weighted EXAFS spectra and (B) corresponding Fourier transforms of the selected sorption samples. a, pH = 2.0, and C0(Th) = 120 mg L−1; b, pH = 3.0, and C0(Th) = 120 mg L−1; c, pH = 3.0, and C0(Th) = 240 mg L−1; d, Th(OH)4. The solid line represents EXAFS results and the dashed line represents the fitted results. | |
Fig. 5B illustrates that all sample spectra exhibit an intense FT peak at 1.9 Å, corresponding to a phase-corrected value of ∼2.4–2.5 Å. This peak represents O atoms comprising the first coordination shell of the Th4+ ions adsorbed on the GO. Reasonable fittings are followed and the results are presented in Table 1. As one can see, the three samples have approximately the same metric parameters, 8.1–8.7 oxygen atoms at 2.45–2.46 Å. The lower coordination number of Th may be due to the lamellar structure of the GO and this structure has some steric effects for the full coordination of Th(IV) to the GO. The Th–O bond lengths obtained in our work are in agreement with the literature.34,37 Additionally, all sample spectra are obviously different from the reference one (Fig. 5B-d), indicating that under the experimental conditions, Th4+ does not deposit on the GO in the form of oxide or hydroxide precipitate. Rothe et al.38 reported that the Th(IV) solution of C0(Th) 1160 mg L−1 at pH 3.02 just started to hydrolyze. Therefore, the predominant species of Th(IV) cations in the currently examined solutions is Th4+. It is well known that Th4+ is a typical hard Lewis acid and displays a strong affinity for oxygen-containing donor ligands. The abundant oxygen-containing functional groups on the surfaces of GO therefore have the capacity to form stable inner-sphere complexes with Th4+ rather than simple physical sorption. Thus, the strong interactions between Th4+ and the GO as well as the high sorption capacity are reasonable.
Table 1 Metric parameters extracted by Least-Squares fitting analysis of EXAFS spectraa
Path |
R (Å)e |
Nf |
σ2 (Å2)g |
ΔE (eV)h |
R-factorI |
Sorption conditions: bpH = 2.0 and C0(Th) = 120 mg L−1, cpH = 3.0 and C0(Th) = 120 mg L−1, and dpH = 3.0 and C0(Th) = 240 mg L−1. eInteratomic distance. fCoordination numbers of neighbors. gDebye−Waller factor. hDifference in the threshold Fermi level between the data and theory. IGoodness-of-fit parameters. |
Th-Ob |
2.46 |
8.1 |
0.0098 |
3.0 |
0.0099 |
Th-Oc |
2.45 |
8.3 |
0.0116 |
2.7 |
0.0101 |
Th-Od |
2.45 |
8.7 |
0.0135 |
2.1 |
0.0118 |
DFT calculations of Th(IV)–GO interactions
According to the results of the EXAFS and the IR spectrum of different GO samples discussed above, the carboxyl or hydroxyl oxygen atoms could bond to Th4+ forming different binding motifs. Here, at the B3LYP/6-31G(d)/RECP level of theory, seven structures with Th(IV) coordinated to carboxyl or hydroxyl oxygen atoms on the edge and middle sites of GO were optimized (Fig. 6). Considering the stronger coordinating ability of NO3− than H2O molecules to Th4+, only NO3− in the first coordination shell of Th4+ were taken into account. As shown in Fig. 6, for A to C, three of the nitrate anions act as bidentate ligands and the other nitrate anion coordinates monodentately to Th4+, whereas all the nitrate anions are coordinated as bidentate ligands for D to G. Thus, the structures of A to C and D to G exhibit 8-fold and 9-fold metal coordination, respectively. The bond lengths between Th atoms and carboxyl or hydroxyl oxygen atoms for the optimized structures were summarized in the ESI (Table S4†). All the Th–O bond lengths for the hydroxyl oxygen atoms are longer than those for the carboxyl oxygen atoms, which suggests that the carboxyl oxygen atoms have stronger coordination ability than hydroxyl. Moreover, both the coordination number and bond lengths were in good agreement with our EXAFS data, indicating that the employed theoretical method is reasonable for modeling Th(IV) complexes.
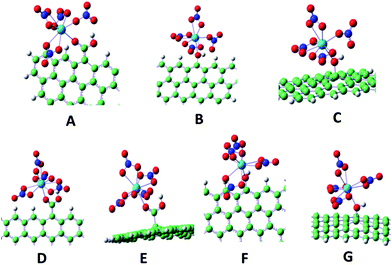 |
| Fig. 6 Optimized binding geometries for the coordination of Th(IV) with GO. Red, white, blue, green and light blue spheres represent O, H, N, C and Th, respectively. | |
The changes of the Gibbs free energies (ΔG) for the complexing reactions were calculated at the B3LYP/6-311G(d,p)/RECP level of theory in the gas phase and aqueous solution. As listed in Table 2, the changes of the Gibbs free energies for all motifs A-G are about −2000 kcal mol−1 in the gas phase and −600 kcal mol−1 in solution. These very negative values indicate that the coordination motifs A–G are all favorable in the gas phase and aqueous solution, which was in good agreement with the high sorption capacity of Th(IV) on GO in our experiments. In addition, the difference of the ΔG values in the gas phase and aqueous solution suggested that solvation energy plays a significant role in the sorption process of Th(IV) on GO.
Table 2 Changes in the Gibbs free energy (kcal mol−1) for the reactions of Th(IV) with GO in the gas and aqueous solution obtained by the B3LYP method
Reactions |
ΔGgas |
ΔGsol |
GO–COOH + 4NO3− + Th4+ → A |
−1970.2 |
−595.2 |
GO–OH + 4NO3− + Th4+ → B |
−1955.7 |
−590.3 |
GO–mOH + 4NO3− + Th4+ → C |
−1964.7 |
−596.6 |
GO–COOH + 4NO3− + Th4+ → D |
−1973.6 |
−600.3 |
GO–mCOOH+4NO3− + Th4+ → E |
−1978.3 |
−603.7 |
GO–OH + 4NO3− + Th4+ → F |
−1959.8 |
−592.9 |
GO–mOH + 4NO3− + Th4+ → G |
−1968.5 |
−601.0 |
In order to provide insight into the bonding nature of these sorption complexes, Mayer bond orders (MBO) and natural population analysis (NPA)25–27 analysis were performed for all motifs A-G. As show in the ESI (Table S5†), the calculated MBO for the Th–O bonds of all structures were from 0.210 to 0.458, indicating that the bonding interactions between Th4+ and the ligands have significant ionic character and the electrostatic interaction dominates these bondings. On the basis of NPA analysis for the Th(IV) atoms, the very positive natural charges on the Th atoms and the negative charges on the O atoms confirmed that the carboxyl and hydroxyl oxygen atoms have stronger coordinating ability to the Th atoms.
Conclusions
The advantages of using GO for the sorption of Th(IV) have been demonstrated. The huge contact area offered by GO maximizes the interaction of the covalently attached oxygen-containing functional groups with the Th(IV) ions. The GO exhibited a high sorption capacity for Th(IV) with a maximum of 214.6 mg g−1 at a relatively low pH of 2.60 ± 0.05. This is attributable to the sufficient exposure of active sites of abundant oxygen-containing functional groups on the GO surface and the huge surface area of GO. The strong sorption property is particularly reflected in the very fast kinetics of sorption of Th(IV) by GO with an equilibrium time of about 10 min. From the results of Th(IV) sorption on GO, we can identify that GO is a suitable material for the enrichment and removal of thorium and other tetravalent actinides from water bodies. The high sorption capacity and fast sorption rate make GO a promising candidate for application in nuclear fuel effluents as well as other related polluted waters.
Acknowledgements
This work was supported by the Natural Science Foundation of China (Grants 91326202, 11205169, 11275219, 21261140335, 91026007, 11105162 and 21101157) and the “Strategic Priority Research program” of the Chinese Academy of Sciences (Grants.XDA030104). We also acknowledge the crew of 1W1B beamline of Beijing Synchrotron Radiation Facility and Shanghai Synchrotron Radiation Facility for their constructive assistance in the course of EXAFS measurements and data analyses. The theoretical results described in this work were obtained on the ScGrid of Supercomputing Center, Computer Network Information Center of Chinese Academy of Sciences.
Notes and references
- N. Chapman and A. Hooper, P. Geologist. Assoc., 2011, 123, 46–63 CrossRef PubMed.
- X. L. Wang, L. Y. Yuan, Y. F. Wang, Z. J. Li, J. H. Lan, Y. L. Liu, Y. X. Feng, Y. L. Zhao, Z. F. Chai and W. Q. Shi, Sci. China Chem., 2012, 55, 1705–1711 CrossRef CAS.
- L. Y. Yuan, Y. L. Liu, W. Q. Shi, Y. L. Lv, J. H. Lan, Y. L. Zhao and Z. F. Chai, Dalton Trans., 2011, 40, 7446–7453 RSC.
- L. Y. Yuan, Y. L. Liu, W. Q. Shi, Z. J. Li, J. H. Lan, Y. X. Feng, Y. L. Zhao, Y. L. Yuan and Z. F. Chai, J. Mater. Chem., 2012, 22, 17019–17026 RSC.
- S. Das, A. K. Pandey, A. A. Athawale and V. K. Manchanda, J. Phys. Chem. B, 2009, 113, 6328–6335 CrossRef CAS PubMed.
- G. Tian, J. Geng, Y. Jin, C. Wang, S. Li, Z. Chen, H. Wang and Y. Zhao, J. Hazard. Mater., 2011, 190, 442–450 CrossRef CAS PubMed.
- L. Al-Attar and A. Dyer, J. Mater. Chem., 2002, 12, 1381–1386 RSC.
- P. Reiller, F. Casanova and V. Moulin, Environ. Sci. Technol., 2005, 39, 1641–1648 CrossRef CAS.
- D. Pan, Q. Fan, P. Li, S. Liu and W. Wu, Chem. Eng. J., 2011, 172, 898–905 CrossRef CAS PubMed.
- P. Sharma and R. Tomar, J. Colloid Interface Sci., 2011, 362, 144–156 CrossRef CAS PubMed.
- M. Schmidt, S. S. Lee, R. E. Wilson, L. Soderholm and P. Fenter, Geochim. Cosmochim. Acta, 2012, 88, 66–76 CrossRef CAS PubMed.
- Y. B. Sun, Q. Wang, C. L. Chen, X. L. Tan and X. K. Wang, Environ. Sci. Technol., 2012, 46, 6020–6027 CrossRef CAS PubMed.
- Z. J. Li, F. Chen, L. Y. Yuan, Y. L. Liu, Y. L. Zhao, Z. F. Chai and W. Q. Shi, Chem. Eng. J., 2012, 210, 539–546 CrossRef CAS PubMed.
- W. J. Zhang, X. H. Shi, Y. X. Zhang, W. Gu, B. Y. Li and Y. Z. Xian, J. Mater. Chem. A, 2013, 1, 1745–1753 CAS.
- Y. M. Ren, N. Yan, J. Feng, J. Ma, Q. Wen, N. Li and Q. Dong, Mater. Chem. Phys., 2012, 136, 538–544 CrossRef CAS PubMed.
- J. Li, S. W. Zhang, C. L. Chen, G. X. Zhao, X. Yang, J. X. Li and X. K. Wang, ACS Appl. Mater. Interfaces, 2012, 4, 4991–5000 CAS.
- M. C. Liu, C. L. Chen, J. Hu, X. L. Wu and X. K. Wang, J. Phys. Chem. C, 2011, 115, 25234–25240 CAS.
- L. J. Cote, F. Kim and J. Huang, J. Am. Chem. Soc., 2008, 131, 1043–1049 CrossRef PubMed.
- M. J. Frisch, G. W. Trucks, H. B. Schlegel, G. E. Scuseria, M. A. Robb, J. R. Cheeseman, G. Scalmani, V. Barone, B. Mennucci and G. A. Petersson, et al., Gaussian, Inc., Wallingford, CT, 2009.
- A. W. Ehlers and G. Frenking, J. Am. Chem. Soc., 1994, 116, 1514–1520 CrossRef CAS.
- A. D. Becke, J. Chem. Phys., 1993, 98, 5648–5652 CrossRef CAS PubMed.
- C. Lee, W. Yang and R. G. Parr, Phys. Rev. B: Condens. Matter Mater. Phys., 1988, 37, 785–789 CrossRef CAS.
- A. V. Marenich, C. J. Cramer and D. G. Truhlar, J. Phys. Chem. B, 2009, 113, 6378–6396 CrossRef CAS PubMed.
- G. Schreckenbach and G. A. Shamov, Acc. Chem. Res., 2010, 43, 19–29 CrossRef CAS PubMed.
- J. P. Foster and F. Weinhold, J. Am. Chem. Soc., 1980, 102, 7211–7218 CrossRef CAS.
- A. E. Reed, R. B. Weinstock and F. Weinhold, J. Chem. Phys., 1985, 83, 735–746 CrossRef CAS PubMed.
- A. E. Reed, L. A. Curtiss and F. Weinhold, Chem. Rev., 1988, 88, 899–926 CrossRef CAS.
- S. Teksoz, C. Acar and P. Unak, J. Chem. Eng. Data, 2009, 54, 1183–1188 CrossRef CAS.
- C. Kuumltahyalinodot and M. Eral, J. Nucl. Mater., 2010, 396, 251–256 CrossRef PubMed.
- X. Wang, C. Chen, X. Li, D. Zhao and X. Tan, Colloids Surf., A, 2007, 302, 449–454 CrossRef PubMed.
- D. Baybas and U. Ulusoy, Appl. Clay Sci., 2011, 51, 138–146 CrossRef CAS PubMed.
- S. Stankovich, R. D. Piner, S. T. Nguyen and R. S. Ruoff, Carbon, 2006, 44, 3342–3347 CrossRef CAS PubMed.
- Y. B. Sun, C. L. Chen, X. L. Tan, D. D. Shao, J. X. Li, G. X. Zhao, S. B. Yang, Q. Wang and X. K. Wang, Dalton Trans., 2012, 41, 13388–13394 RSC.
- F. Seco, C. Hennig, J. d. Pablo, M. Rovira, I. Rojo, V. Marti, J. Giménez, L. Duro, M. Grivé and J. Bruno, Environ. Sci. Technol., 2009, 43, 2825–2830 CrossRef CAS.
- Z. Wang, S. W. Lee, J. G. Catalano, J. S. Lezama-Pacheco, J. R. Bargar, B. M. Tebo and D. E. Giammar, Environ. Sci. Technol., 2013, 47, 850–858 CrossRef CAS PubMed.
- H. B. Jung, M. I. Boyanov, H. Konishi, Y. Sun, B. Mishra, K. M. Kemner, E. E. Roden and H. Xu, Environ. Sci. Technol., 2012, 46, 7301–7309 CrossRef CAS PubMed.
- R. Dahn, A. M. Scheidegger, A. Manceau, E. Curti, B. Baeyens, M. H. Bradbury and D. Chateigner, J. Colloid Interface Sci., 2002, 249, 8–21 CrossRef PubMed.
- J. Rothe, M. Denecke, V. Neck, R. Müller and J. Kim, Inorg. Chem., 2002, 41, 249–258 CrossRef CAS PubMed.
Footnote |
† Electronic supplementary information (ESI) available: The details for synthesis and characterization methods of GO, the kinetic models, the Langmuir and Freundlich isotherm models, the calculation of thermodynamic data, corresponding 6 figures and 5 tables describing the models and the effect of solid-to-liquid, Th–O bond lengths and MBO and natural charges. See DOI: 10.1039/c3ra45938a |
|
This journal is © The Royal Society of Chemistry 2014 |