DOI:
10.1039/C3RA45893H
(Paper)
RSC Adv., 2014,
4, 3701-3705
“Turn-on” fluorescent detection of cyanide based on polyamine-functionalized carbon quantum dots†
Received
17th October 2013
, Accepted 13th November 2013
First published on 15th November 2013
Abstract
A simple, sensitive, rapid fluorescence (FL) sensor has been developed to detect cyanide ions (CN−) based on the branched polyethylenimine-capped carbon quantum dots (BPEI-GQDs). The amino groups at the surfaces of BPEI-GQDs can selectively and sensitively capture copper ions (Cu2+) to form absorbent cupric amine, which can quench the FL of BPEI-GQDs through an inner filter effect. CN− can combine strongly with Cu2+ to [Cu(CN)x]n− species, preventing Cu2+ from being captured by the amino groups of BPEI-GQDs. As a result, Cu2+ can't quench the FL of BPEI-GQDs anymore in the presence of CN−. In other words, CN− can “turn on” the FL signal of BPEI-GQDs/Cu2+ system, producing a “recovered” FL signal. After optimizing experimental conditions, we demonstrated that this easy methodology could offer a sensitive, selective, simple and rapid method for detection of CN− with a detection limit of 0.65 μM (S/N = 3) and a linear response range of 2 to 200 μM.
Introduction
Carbon based dots (CDs) mainly include carbon nanoparticles of less than 10 nm in size (so-called carbon quantum dots, CQDs) and graphene nanosheets of usually less than 10 nm in width (so-called graphene quantum dots, GQDs).1,2 CDs usually show unique optical properties like those of semiconductor based quantum dots (QDs). Furthermore, they also show many additional advantages, such as low cytoxicity, good stability, easy preparation, and environmental friendliness. Therefore, CQDs and GQDs have been proposed to be promising candidates to replace conventional QDs, and have attracted more and more attention.3–5 To date, many easy methods have been developed to prepare various fluorescent CDs. The obtained CDs were also applied in many fields, such as photovoltaic devices,6,7 cellular imaging,8,9 drug delivery,9,10 and particularly analytical detections.11,12 As fluorescence (FL) probes for analytical applications, CDs are demanded to be highly fluorescent and specially functionalized for the analyte. In our previous study, the mixture of citric acid (CA) and branched polyethylenimine (BPEI) was heated at 200 °C to prepare highly fluorescent (42.5% FL quantum yield) BPEI functioned CQDs (BPEI-CQDs).13 In our further study, it was found that the amino groups of the BPEI-CQDs could selectively capture copper ions (Cu2+) to form an absorbent cupric amine at the surface of CQDs, resulting in a strong quenching of the CQDs' FL through an inner filter effect. Finally, a “turn-off” fluorescent sensing system for detecting Cu2+ in water samples was developed.12 As well known, cyanide ions (CN−) can combine strongly with Cu2+ to form [Cu(CN)x]n− species (eqn (1) and (2)).14–16 |
2Cu2+ + 4CN− = 2CuCN + (CN)2
| (1) |
|
CuCN + (X − 1)CN− = [Cu(CN)x]n−
| (2) |
where x = 2–4 and is generally 2 or 4. The stability constants of [Cu(CN)2]− and [Cu(CN)4]3− are 1.00 × 1024 and 2.00 × 1030, respectively. That is to say, CN− can hinder the formation of cupric amine between the amino groups of BPEI-GQDs and Cu2+. In other words, in the presence of CN−, Cu2+ should not quench the FL signal of BPEI-GQDs anymore. Herein, we demonstrate a fluorescent “turn-on” sensing system for CN− based on the BPEI-GQDs/Cu2+ system.
CN− is one of the most toxic anions. It can combine with the ferric iron in the cytochrome oxidase intensively, leading to the body hypoxia and further poisoning or death.17 Moreover, CN− is widely used in industry, such as mining and industrial organic chemistry. Therefore, developing efficient sensing systems for CN− is of significance for environmental monitor and food safety. Various methods have been developed to detect and quantify CN− in water sample over the past twenty years. Fluorescent methods usually show many advantages, such as rapid response, high sensitivity, good selectivity, excellent reproducibility, low cost and easy-operation.18–22 Many fluorescent probes have been applied in sensing CN−, including organic fluorophores,23,24 gold nanoclusters,25 polymer microspheres26 and Cd-based semiconductor QDs.27 However, these fluorescent probes usually have some disadvantages. For example, complex organic synthesis is often harmful for human bodies and Cd-containing QDs are toxic to the ecological environment. Therefore, the establishment of low-toxic, selective and sensitive BPEI-GQDs/Cu2+-based fluorescent “turn-on” sensing system for CN− is of significantly important.
Experimental
Chemicals
Citric acid was purchased from Alfa Aesar (USA). Branched poly (ethylenimine) (M = 1800) was obtained from Aladdin Chemical Co., Ltd, (Shanghai, China). All other reagents were of analytical grade and used as received. Doubly distilled water was used throughout the experiments. Phosphate-buffered saline (PBS) solutions of different pH values were prepared by titrating 0.01 M phosphoric acid solution with a concentrated sodium hydroxide solution (1 M) to the required pH values.
Synthesis of BPEI-CQDs
The BPEI-capped CQDs were synthesized by pyrolyzing the mixture of CA and BPEI according a method that has been described elsewhere.12,13
Analysis of real sample
The water sample was collected from Min River (Fujian, China). The sample was filtered through a 0.22 μm membrane (Millipore) prior to the detection. Aliquots (500 μL) of this river water were spiked with standard CN− solutions (final concentration, 0–200 μM). The spiked samples were then diluted to 1000 μL with PBS (10 mM, pH 7.0) containing BPEI-CQDs (final concentration 16.8 μg mL−1) and Cu2+ (final concentration 15 μM), then analyzed using the developed sensing technique.
Instrumentation
UV-visible spectra were obtained by a LAMBDA 750 (PerkinElmer, USA) FL spectra were obtained using a Caryeclipse (Varian, USA) fluorescence spectrophotometer.
Results and discussion
FL recovery of BPEI-CQDs/Cu2+ system by CN−
As reported in our previous work,12 the amino groups at the surfaces of BPEI-CQDs can selectively and sensitively capture Cu2+ to form absorbent cupric amine, which quenches the FL signal of BPEI-CQDs by the inner filter effect. Furthermore, the quenched FL signal can be recovered by a strong metal ion chelator such as ethylenediaminetetraacetate (EDTA). Besides the EDTA, CN− is another well-known chelator that can combine Cu2+ strongly to stable [Cu(CN)x]n− species, based on which many FL turn-on sensing system have been developed for detecting CN−.27,28 Therefore, it was believed that CN− can also recover the FL signal of BPEI-CQDs which is quenched by Cu2+. However, the experimental results came out much differently. When the FL of 0.168 mg mL−1 BPEI-CQDs was quenched by 150 μM Cu2+, CN− could not recover the quenched FL signal even at a rather high concentration (5 mM). It is because that the abundant amino groups on the surfaces of PEI-capped CQDs will react with CN−,29 hindering the formation of [Cu(CN)x]n− species. As a result, CN− can't remove Cu2+ from the surfaces the BPEI-CQDs, and accordingly can't recover the FL signal of the BPEI-CQDs/Cu2+ system. In a control experiment, CN− was added firstly into the Cu2+ solution, and the BPEI-CQDs were then added into the solution. As shown in Fig. 1, the bright FL of 0.168 mg mL−1 BPEI-CQDs is quenched dramatically by 150 μM Cu2+, but is quenched very slightly by the mixture of 150 μM Cu2+ and 2 mM CN−. That is to say, CN− can inhibit the quenching effect of Cu2+ on the FL signal of BPEI-CQDs, producing a “recovered” FL signal. The corresponding UV-vis absorption spectra indicate that CN− can inhibit the formation of absorbent cupric amine between the amino groups and Cu2+. Herein, the mechanism of the FL “recovery” of BPEI-CQDs/Cu2+ system by CN− can be proposed in Fig. 2. CN− reacts with Cu2+ to produce stable [Cu(CN)x]n− species, keeping Cu2+ from being captured by the amino groups of BPEI-CQDs. As a result, the FL quenching effect of Cu2+ on the FL of BPEI-CQDs is inhibited. Accordingly, a sensing system of CN− can be developed based on the “recovered” FL signal of the BPEI-CQDs/Cu2+ system.
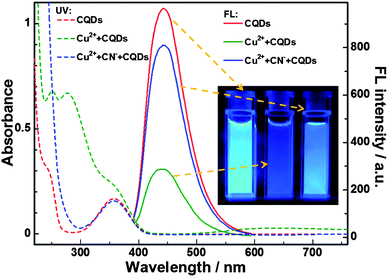 |
| Fig. 1 UV-vis absorption and FL spectrum of 0.168 mg mL−1 BPEI-CQDs (red lines), 150 μM Cu2+ + 0.168 mg mL−1 BPEI-CQDs (green lines), and 2 mM CN− + 150 μM Cu2+ + 0.168 mg mL−1 BPEI-CQDs (blue lines) in pH 7 PBS. The inset shows the corresponding photos of the three solutions illuminated by an UV beam of 365 nm. | |
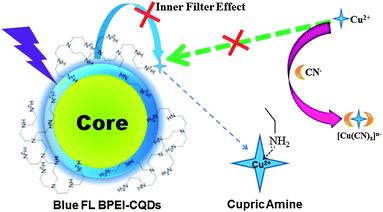 |
| Fig. 2 Diagram for the “recovery” effect of cyanide on the fluorescence of BPEI-CQDs/Cu2+ system. | |
Establishment of the FL sensing method for CN−
Since the CN− sensing system is based on the “recovered” FL signal of BPEI-CQDs quenched by Cu2+, both the concentration effects of BPEI-CQDs and Cu2+ on the FL inhibition and recovery should be estimated. On one hand, according to our previous results, 16.8 μg mL−1 BPEI-CQD solution had the most sensitive response to the change of Cu2+ concentration, and was thus used in the sensing system. On the other hand, as mentioned above, CN− “recover” the FL signal of the BPEI-CQDs/Cu2+ system by combining Cu2+ into stable [Cu(CN)x]n− species. In other words, increasing the concentration of CN− is decreasing the concentration of free Cu2+ in the solution. When the concentration of Cu2+ is lower than 15 μM, the FL intensity of BPEI-CQDs decreases sensitively as the concentration of Cu2+ is increased (Fig. S1†). However, when the concentration of Cu2+ is higher than 15 μM, the FL intensity of BPEI-CQDs is relatively insensitive to the concentration's change of Cu2+. Accordingly, 15 μM Cu2+ was used in the sensing system to obtain a high sensitivity and wide response range.
Effect of pH value was also investigated since pH of solution may affect not only the FL intensity of the BPEI-CQDs/Cu2+ and the quenching effect of Cu2+, but also the FL “recovery” efficiency by CN−. Generally speaking, acidic condition is unsuitable for the detection of CN− due to the formation of toxic hydrogen cyanide (HCN). Therefore, the pH effect was investigated only in the range of pH 7–12. Experimental results indicate that the neutral solution (pH = 7) is the optimal condition for the sensing system (Fig. 3). First of all, BPEI-CQDs show the best FL activity in neutral solution, and the FL intensity decreases dramatically with the increase of pH value of the solution. Secondly, Cu2+ shows the best quenching efficiency to the FL of BPEI-CQDs in the neutral solution ((F0 − F1)/F0, where F0 and F1 are respectively the FL intensities of BPEI-CQDs in the absence and presence of Cu2+), the quenching efficiency decreases obviously with the increase of pH value. Finally, CN− exhibits the highest “recovery” efficiency in the neutral solution ((F2 − F1)/(F0 − F1), where F2 is the FL intensity of BPEI-CQDs in the presence of Cu2+ and CN− mixture) for the FL of BPEI-CQDs quenched by Cu2+. Therefore, the neutral medium was chosen for this sensing system.
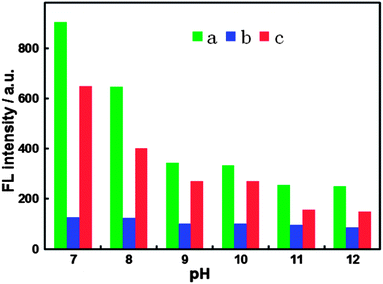 |
| Fig. 3 FL responses of 16.8 μg mL−1 BPEI-CQDs (a), 15 μM Cu2+ + 16.8 μg mL−1 BPEI-CQDs (b), and 150 μM CN− + 15 μM Cu2+ + 16.8 μg mL−1 BPEI-CQDs (c) at different pH values. | |
Time-dependent FL change of the “recovery” system was investigated. The “recovery” effect of CN− on the FL of BPEI-CQDs/Cu2+ system is not very stable against time. As shown in Fig. 4, the “recovery” efficiency decreases gradually from 67.6 to 44.2% in 1 hour's observation. The corresponding UV-vis spectra show that the absorption bands produced by the cupric amine (an absorption band centered at 275 nm and the other broad band found in the range from 500 to 800 nm) increases with time increasing (Fig. S2†). It is supposed that Cu2+ can be released gradually from the [Cu(CN)x]n−. In other words, the [Cu(CN)x]n− is not stable enough in the presence of abundant amino groups. In order to detect CN− sensitively and accurately, the FL signal was measured after BPEI-CQDs were added into the solution for 1 min.
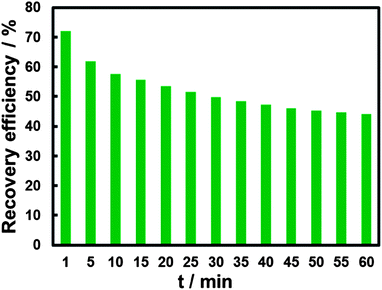 |
| Fig. 4 Time-dependent FL response of the 16.8 μg mL−1 BPEI-CQDs in pH 7 PBS upon the addition of 15 μM Cu2+ and 150 μM CN−. | |
The linear response range and detection limit of this sensing system was subsequently investigated under the optimized conditions discussed above. As shown in Fig. 5, the FL intensity of BPEI-CQDs/Cu2+ system is sensitive to CN− and “recovered” gradually with the concentration of CN−. There is a good linear relationship between “recovered” FL signal and the concentration of CN− in the range from 2 to 200 μM (see inset of Fig. 5 and eqn (1)).
|
F2 − F1 = 2.509 × 106C + 13.67 (R2 = 0.991)
| (1) |
where
C is the concentration of CN
−. The theoretical detection limit was calculated to be 6.5 × 10
−7 M (at a
S/
N of 3), which is lower than the maximum allowable concentration for drinking water containing CN
− ordained by international standards (MCL: 2.7 × 10
−6 M). This result implies the practical significance of the sensing system.
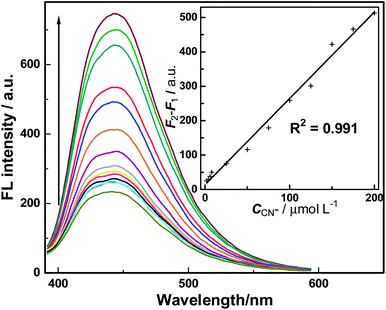 |
| Fig. 5 FL response of BPEI-CQDs/Cu2+ system (BPEI-CQDs: 16.8 μg mL−1, Cu2+ 15 μM) upon addition of various concentrations of CN− ions in a pH 7 PBS solution (from bottom: 0, 2, 4, 6, 8, 10, 25, 50, 75, 100, 125, 150, 175, 200 μM). Inset: linear range of the plot of “recovered” FL intensity against the concentration of CN− (2–200 μM). | |
The selectivity of this sensing system for CN− was evaluated before being applied in real sample analysis. Besides CN−, 15 kinds of common anions including SCN−, NO3−, AC−, PO43−, CO32−, SO32−, citrate, NO2−, SO42−, I−, IO3−, Br−, Cl−, C2O42− and S2− were investigated. As shown in Fig. 6, most anions have no obvious effects on the FL response of the BPEI-CQDs/Cu2+ system, except for C2O42− further quenched slightly the FL of BPEI-CQDs/Cu2+ system. This result indicates that this sensing system is highly selective towards cyanide over other anions.
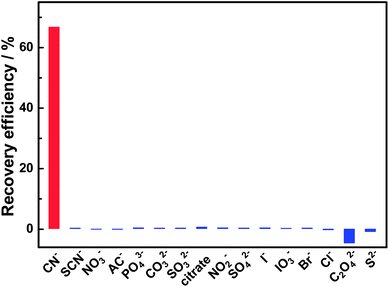 |
| Fig. 6 Selectivity of the BPEI-CQDs/Cu2+ system sensor for CN− over other ions in pH 7 PBS: concentrations of BPEI-CQDs and Cu2+ were 16.8 μg mL−1 and 15 μM respectively; concentrations of all anions were 150 μM. | |
Applications
Base on the results mentioned above, the application of the sensing system in real water sample (collected from Min River, the largest river in Fujian Province, China) was investigated. Since no cyanide was detected in the river water sample, 50 μM CN− was spiked before the sample was detected using a standard addition method. The recovery of the measurement was 96.53%, with a relative standard deviation of 6.32 (n = 10). This result demonstrates a high potential of the sensing system for the analysis of trace levels of CN− in real sample.
Conclusions
We have demonstrated a FL “turn on” sensing method for the detection of CN− in aqueous solution based on the BPEI-CQDs/Cu2+ system. CN− combines Cu2+ strongly to produce stable [Cu(CN)x]n− species, hindering the formation of absorbent cupric amine. As a result, CN− produces a “recovered” FL signal of the BPEI-CQDs/Cu2+ system through inhibiting the quenching effect of Cu2+ on the FL of BPEI-CQDs. This sensing system shows many advantages, such as good sensitivity, selectivity, wide linear response range, and low cost. Furthermore, this research further promotes the analytical application of CDs.
Acknowledgements
This study was financially supported by National Natural Science Foundation of China (21075018, 21305017), Program for New Century Excellent Talents in Chinese University (NCET-10-0019), and Program for Changjiang Scholars and Innovative Research Team in University (no. IRT1116).
Notes and references
- Y. P. Sun, B. Zhou, Y. Lin, W. Wang, K. S. Fernando, P. Pathak, M. J. Meziani, B. A. Harruff, X. Wang, H. Wang, P. G. Luo, H. Yang, M. E. Kose, B. Chen, L. M. Veca and S. Y. Xie, J. Am. Chem. Soc., 2006, 128, 7756 CrossRef CAS PubMed
. - L. A. Ponomarenko, F. Schedin, M. I. Katsnelson, R. Yang, E. W. Hill, K. S. Novoselov and A. K. Geim, Science, 2008, 320, 356 CrossRef CAS PubMed
. - S. N. Baker and G. A. Baker, Angew. Chem., Int. Ed., 2010, 49, 6726 CrossRef CAS PubMed
. - J. Shen, Y. Zhu, X. Yang and C. Li, Chem. Commun., 2012, 48, 3686 RSC
. - Z. Zhang, J. Zhang, N. Chen and L. Qu, Energy Environ. Sci., 2012, 5, 8869 CAS
. - X. Yan, X. Cui, B. Li and L. S. Li, Nano Lett., 2010, 10, 1869 CrossRef CAS PubMed
. - Y. Li, Y. Hu, Y. Zhao, G. Shi, L. Deng, Y. Hou and L. Qu, Adv. Mater., 2011, 23, 776 CrossRef CAS PubMed
. - J. Peng, W. Gao, B. K. Gupta, Z. Liu, R. Romero-Aburto, L. Ge, L. Song, L. B. Alemany, X. Zhan, G. Gao, S. A. Vithayathil, B. A. Kaipparettu, A. A. Marti, T. Hayashi, J. J. Zhu and P. M. Ajayan, Nano Lett., 2012, 12, 844 CrossRef CAS PubMed
. - X. Sun, Z. Liu, K. Welsher, J. T. Robinson, A. Goodwin, S. Zaric and H. Dai, Nano Res., 2008, 1, 203 CrossRef CAS PubMed
. - Z. Liu, J. T. Robinson, X. Sun and H. Dai, J. Am. Chem. Soc., 2008, 130, 10876 CrossRef CAS PubMed
. - Y. Dong, G. Li, N. Zhou, R. Wang, Y. Chi and G. Chen, Anal. Chem., 2012, 84, 8378 CrossRef CAS PubMed
. - Y. Dong, R. Wang, G. Li, C. Chen, Y. Chi and G. Chen, Anal. Chem., 2012, 84, 6220 CrossRef CAS PubMed
. - Y. Dong, R. Wang, H. Li, J. Shao, Y. Chi, X. Lin and G. Chen, Carbon, 2012, 50, 2810 CrossRef CAS PubMed
. - K. Kurnia, D. E. Giles, P. M. May, P. Singh and G. T. Hefter, Talanta, 1996, 43, 2045 CrossRef CAS
. - Z. Xu, X. Chen, H. N. Kim and J. Yoon, Chem. Soc. Rev., 2010, 39, 127 RSC
. - X. Lou, L. Qiang, J. Qin and Z. Li, ACS Appl. Mater. Interfaces, 2009, 11, 2529 Search PubMed
. - M. Panda and N. C. Robinson, Biochemistry, 1995, 34, 10009 CrossRef CAS
. - X. Zhang, Z. Yang, Z. Chi, M. Chen, B. Xu, C. Wang, S. Liu, Y. Zhang and J. Xu, J. Mater. Chem., 2010, 20, 292 RSC
. - X. Zhang, Z. Chi, Y. Zhang, S. Liu and J. Xu, J. Mater. Chem. C, 2013, 1, 3376 RSC
. - M. Peng, Y. Guo, X. Yang, L. Wang and J. An, Dyes Pigm., 2013, 98, 327 CrossRef CAS PubMed
. - M. Zhu, Z. Gu, R. Zhang, J. Xiang and S. Nie, Talanta, 2010, 81, 678 CrossRef CAS PubMed
. - Y. Oztekin, Z. Yazicigil, A. O. Solak, Z. Ustundag and A. Okumus, Sens. Actuators, B, 2012, 166–167, 117 CrossRef CAS PubMed
. - S. Saha, A. Ghosh, P. Mahato, S. Mishra, S. K. Mishra, E. Suresh, S. Das and A. Das, Org. Lett., 2010, 12, 3406 CrossRef CAS PubMed
. - X. Wu, B. Xu, H. Tong and L. Wang, Macromolecules, 2011, 44, 4241 CrossRef CAS
. - Y. Liu, K. Ai, X. Cheng, L. Huo and L. Lu, Adv. Funct. Mater., 2010, 20, 951 CrossRef CAS
. - A. Álvarez-Diaz, A. Salinas-Castillo, M. Camprubí-Robles, J. M. Costa-Fernández, R. Pereiro, R. Mallavia and A. Sanz-Medel, Anal. Chem., 2011, 83, 2712 CrossRef PubMed
. - A. Touceda-Varela, E. I. Stevenson, J. A. Galve-Gasión, D. T. Dryden and J. C. Mareque-Rivas, Chem. Commun., 2008, 1998 RSC
. - L. Shang, L. Zhang and S. Dong, Analyst, 2009, 134, 107 RSC
. - J. G. Erickjson, J. Org. Chem., 1955, 20, 1569 CrossRef
.
Footnote |
† Electronic supplementary information (ESI) available: Fig. S1 and S2. See DOI: 10.1039/c3ra45893h |
|
This journal is © The Royal Society of Chemistry 2014 |