DOI:
10.1039/C3RA44709J
(Paper)
RSC Adv., 2014,
4, 3299-3305
Composite of graphene quantum dots and Fe3O4 nanoparticles: peroxidase activity and application in phenolic compound removal†
Received
28th August 2013
, Accepted 23rd October 2013
First published on 24th October 2013
Abstract
Graphene quantum dots (GQDs) are graphene sheets with lateral sizes less than 100 nm, and have a higher electron conjugate state and a better dispersion ability in aqueous solution compared to micrometer-sized graphene oxide (GO) sheets. Therefore they can overcome the drawbacks of GO and are an ideal candidate for nano-composites. In this work, composites of GQDs and Fe3O4 nanoparticles (NPs) (GQDs/Fe3O4) were prepared via a one-step co-precipitation approach. The as-prepared GQDs/Fe3O4 composites showed superb peroxidase-like activities, which were much higher than composites of micrometer sized GO and Fe3O4 NPs (GO/Fe3O4), individual GQDs, and individual Fe3O4 NPs. The excellent peroxidase activities of the GQDs/Fe3O4 composites can be attributed to the unique properties of GQDs and the synergistic interactions between the GQDs and Fe3O4 NPs. The GQDs/Fe3O4 composites also exhibited a higher stability and reusability than natural peroxidases. The application of a GQDs/Fe3O4 composite as a catalyst for the removal of phenolic compounds from aqueous solutions was explored with nine phenolic compounds, and showed better or comparable removal efficiencies for some phenolic compounds compared to native horseradish peroxidase (HRP) under the same conditions. The extraordinary catalytic performance and physical properties of the as-prepared GQDs/Fe3O4 composite render it practically useful for industrial wastewater treatment.
Introduction
Natural peroxidases, such as horseradish peroxidase (HRP), vanadium haloperoxidase and glutathione peroxidase, are well known catalysts for many oxidation reactions in the presence of a peroxide (H2O2 in most cases).1–3 However, the utilization of natural peroxidases on a large scale remains challenging, mainly because of their high costs and low stabilities. Therefore, the exploration of peroxidase mimic systems with high stability and activity has attracted great attention during the last few decades.4–6 For instance, Fe3O4,7–9 Fe2O3,10–12 Au,13 Pt,14 Co3O4,15,16 V2O5,17,18 CuO19 and RuO220 nanoparticles (NPs) possess peroxidase-like catalytic activity toward the reduction of H2O2, and have been used in various fields such as H2O2 detection,21 immunoassays,7 glucose detection,22,23 nucleic acids detection24,25 and cytotoxicity assays.26 Due to the low cost, easy preparation, high thermal stability and reusability in comparison to natural peroxidases, NPs have also been used as catalysts for the oxidation degradation of organic pollutants in water.27–31 Unfortunately, the peroxidase-like activity of most bare NPs is relatively low.32 Additionally, without proper surface modification, bare NPs agglomerate quickly, and thus their effective surface area and peroxidase-like activity are suppressed.
Single-atomic layered graphene oxide (GO), due to its high electron conjugate state and abundant surface oxygen-containing groups, has showed peroxidase-like activity to catalyze the oxidation of 3,3,5,5-tetramethylbenzidine (TMB) with H2O2.33 More attractively, GO sheets can serve as matrices for templating or anchoring different nanoparticles on them, which can dramatically enhance the properties of the nanoparticles.34–40 For example, a Au NPs/GO hybrid exhibited a synergetic and switchable peroxidase-like activity in response to specific DNA molecules.35 The GO supported magnetic Fe3O4 NPs also showed a relatively stronger peroxidase-like catalytic activity for the reduction of H2O2 compared to the NPs alone.41–43 However, the lateral size of GO sheets generated from graphite oxidation and exfoliation can usually reach several micrometers or even larger, which is sometimes a drawback for using GO composites as catalysts. Graphene quantum dots (GQDs) are graphene sheets with lateral sizes less than 100 nm and have several unique properties, such as a better dispersing capability in aqueous solutions, compared to micrometer-sized GO sheets.44,45 Actually, we have demonstrated previously that GQDs, prepared through the photo-Fenton reaction of GO, with a defect-free 2D hexagonal lattice structure and enriched periphery carboxylic groups, have a much higher intrinsic peroxidase-like activity compared to micrometer sized GO, and show a greater performance and stability in H2O2 detection.46,47
Herein, we explore the peroxidase-like activity of composites of GQDs with Fe3O4 NPs. To this end, we have prepared and fully characterized the composites of GQDs and Fe3O4 NPs (GQDs/Fe3O4). We have illustrated that the as-obtained GQDs/Fe3O4 composites exhibited a much higher peroxidase-like activity than the composites of micrometer sized graphene oxide (GO) and Fe3O4 NPs (GO/Fe3O4), individual GQDs and Fe3O4 NPs. The GQDs/Fe3O4 composite with a GQDs to Fe3O4 ratio of 1
:
1 (in weight) showed the highest catalytic activity for the oxidation of 3,3′,5,5′-tetramethylbenzidine (TMB) with H2O2, which was about 22 fold higher than for individual Fe3O4 NPs, 25 fold higher than for GQDs, and 9 fold higher than for GO/Fe3O4 (1–1). The GQDs/Fe3O4 composites were stable over a wide range of temperatures and showed good reusability. In addition, we explored the potential application of GQDs/Fe3O4 composites for the removal of phenolic compounds. Although the removal efficiency was slightly lower than that with the same amount of HRP, the high thermal stability, easy-preparation and low cost of the GQDs/Fe3O4 composites still show its potential in the treatment of industrial wastewater.
Experimental methods
Materials
Horseradish peroxidase (HRP) was purchased from Majorbio Biotech Company, USA. 4-Aminoantipyrine (4-AAP) was bought from East China Normal University Chemical Factory. Other reagents were obtained from Sinopharm Chemical Reagent Co. Ltd., Shanghai, China. All reagents were used as received.
Fabrication of the GQDs/Fe3O4 and GO/Fe3O4 composites
GO and GQDs were prepared as described in our previous work.46,48,49 The GQDs/Fe3O4 composites were synthesized through a one-step co-precipitation procedure. In a typical experiment, 67.5 mg (0.25 mmol) of FeCl3·6H2O and 34.75 mg (0.125 mmol) of FeSO4·7H2O (with a molar ratio of 2
:
1) were dissolved in 2 mL of an acidic aqueous solution with pH = 5, which was adjusted by diluted HCl to decrease the hydrolysis of Fe2+. The GQDs (2.9 mg mL−1) were dispersed in water with pH = 12, which was adjusted with ammonium hydroxide. All solutions were maintained in a N2 atmosphere. Then, the solution of ferrous and ferric salts was added drop-wisely into the solution of GQDs with vigorous stirring at 25 °C in a nitrogen atmosphere. The ratios of GQDs to Fe3O4 (theoretically estimated based on the initial amount of GQDs and ferrous and ferric salts) were controlled to be 10/1, 5/1, 2/1, 1/1, 1/2, 1/5, and 1/10 in weight, respectively. After 90 min of reaction, the precipitates were collected by centrifugation and washed with water for 5 times. The solid products were then re-dispersed in distilled water and stored at 4 °C. The as-obtained composites were named as GQDs/Fe3O4 10–1, 5–1, 2–1, 1–1, 1–2, 1–5, and 1–10 accordingly. For comparison, the GO/Fe3O4 composites with GO to Fe3O4 ratios of 10/1, 5/1, 2/1, 1/1, 1/2, 1/5, and 1/10 were prepared through a similar procedure, and were named as GO/Fe3O4 10–1, 5–1, 2–1, 1–1, 1–2, 1–5, and 1–10 accordingly. Bare Fe3O4 NPs were also prepared.
Characterization
Atomic force microscope (AFM) images were taken on a MultiMode Nanoscope V scanning probe microscopy system (Veeco, USA). AFM cantilever tips with a force constant of ∼48 N m−1 and a resonance vibration frequency of ∼330 kHz were used and the scanning rate was set at 1 Hz. Transmission electron microscope (TEM) images were obtained using a JEM-2010 transmission electron microscope (JEOL, Japan). X-ray photoelectron spectroscopy (XPS) measurements were performed on an Axis Ultra DLD spectrometer (Kratos Analytical, UK) using a monochromated Al Kα source at 15 kV. FTIR spectra were acquired on a Nicolet 6700 FT-IR (Thermo Electron, USA) in the range of 4000–400 cm−1. The samples for the FTIR measurements were prepared by grinding the dried powder with KBr and then compressing them into thin pellets. The magnetic properties were measured using a vibrating sample magnetometer (VSM, Lakeshore 736, USA) at 300 K. Thermogravimetric (TG) analyses were carried out using a thermogravimetric analyzer (Shimadzu DSC-50, Japan) in a 30–1000 °C temperature range at a scan rate of 10 °C min−1 using stainless-steel pans under an air atmosphere. UV-visible absorption spectra were recorded using a Shimadzu UV-2550 (Shimadzu, Japan).
Peroxidase-like activity assay
The peroxidase-like activity of the GQDs/Fe3O4 composites was assayed as following: GQDs/Fe3O4 (10 μg) was first dispersed in 1 mL of NaAc buffer (0.1 M, pH 3.5) containing 0.9 mM TMB and 5 mM H2O2, as substrates, in a 1 mL quartz cuvette. The mixture was measured in time course mode by monitoring the absorbance change at 652 nm for 300 s on a UV-visible spectrophotometer at 30 °C. Similar procedures were also performed for GO/Fe3O4, GQDs and Fe3O4.
Phenolic compounds removal
The removal of phenolic compounds using GQDs/Fe3O4 1–1 as the catalyst in the presence of H2O2 was explored. In a typical experiment, 10 μg of GQDs/Fe3O4 1–1 was added in 1 mL of NaAc buffer (0.1 M, pH 3.5) containing 6 mM of phenol and 30 mM of H2O2, and then incubated at 30 °C for 3 h. To compare the peroxidase-like activity of GQDs/Fe3O4 1–1 with that of HRP, 10 μg of HRP was used to perform the same assay.
After 3 h of reaction, the removal efficiency was determined by measuring the residual phenolic compounds present in the supernatant with potassium ferricyanide and 4-aminoantipyrine (4-AAP).50 Typically, after centrifugation, 25 μL of the clear supernatant from the reaction was mixed in turn with 775 μL of phosphate buffer (0.1 M, pH 7.0), 100 μL of potassium ferricyanide (83.4 mM in 0.25 M sodium bicarbonate solution), and 100 μL of 4-AAP (20.8 mM in 0.25 M sodium bicarbonate solution). The total volume reached 1 mL. After the color of the mixture had developed completely over 5 minutes, the absorbance was measured at 490 nm against a blank (800 μL of phosphate buffer, 100 μL of potassium ferricyanide, and 100 μL of 4-AAP solution). For the phenolic compounds which cannot produce a colorimetric product under these conditions, such as 2-aminophenol, 1,4-benzenediol and catechol, the absorbances of their characteristic absorption peaks were measured directly.
Results and discussion
Characterization of the GQDs/Fe3O4 composites
The morphologies of the GQDs and GO sheets used in this work were characterized using AFM imaging (acquired under tapping mode) and the images are depicted in Fig. S1, ESI.† The average heights of both the GO sheets and the GQDs were ∼1 nm (Fig. S1 inset, ESI†), demonstrating their single atomic layer feature.48,49 Fig. 1a and b show the morphology of the as-prepared GQDs/Fe3O4 1–1 composite. Differing from the two dimensional layered structures of GQDs and GO, the Fe3O4 NPs and GQDs aggregated into three dimensional structures. Most probably, as schematically depicted in Fig. 1c, the Fe3O4 NPs are surrounded by GQDs to form composite particles. The sizes of the GQDs/Fe3O4 particles reached 30 nm (Fig. 1a). To get an insight into the hyperfine chemical structure, the FTIR spectra of GQDs and GQDs/Fe3O4 1–1 were measured and are shown in Fig. 2a. The appearance of the stretching vibrations peak at 597 cm−1 for Fe–O suggests the presence of iron oxide. Comparably, besides the vibration and deformation peaks of the O–H groups at 3409 cm−1 and 1384 cm−1, the C–O (epoxy) stretching vibration peak at 1227 cm−1, and the C–O (alkoxy) stretching peak at 1032 cm−1, after the formation of the composite, the C
O stretching vibration peak shifted from 1736 to 1624 cm−1, indicating that the periphery carboxylic groups of the GQDs had been converted into carboxylate groups. This result led us to believe that the GQDs bound to the Fe3O4 particles through, most probably, Fe–O chemical bonds. Some other new bands at 1387 and 1227 cm−1 may also be attributed to Fe–O bond formation between the carboxyl groups in GQDs and the iron atoms in the Fe3O4 NPs.
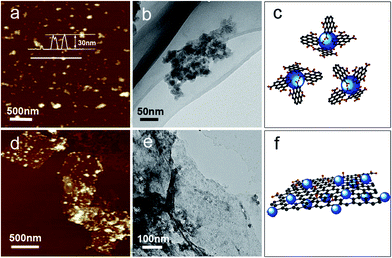 |
| Fig. 1 AFM images, TEM images, and schematic representations of the structures of the GQDs/Fe3O4 1–1 (a, b, c) and GO/Fe3O4 1–1 (d, e, f) composites, respectively. | |
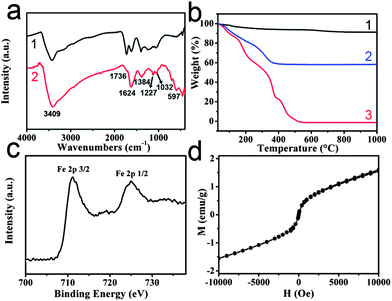 |
| Fig. 2 (a) FTIR spectra of the GQDs (1) and the GQDs/Fe3O4 1–1 composite (2); (b) TG curves of the Fe3O4 NPs (1), GQDs/Fe3O4 1–1 (2) and the GQDs (3); (c) XPS spectrum of Fe 2p in GQDs/Fe3O4 1–1; (d) hysteresis loop for GQDs/Fe3O4 1–1. | |
The content of GQDs in the composites is a key issue to understand their structures. Fig. 2b shows the TG curves for the GQDs/Fe3O4 1–1 composite. In an air atmosphere, the mass loss can be mainly attributed to the decomposition of the GQDs. At 1000 °C, the Fe3O4 NPs alone retains 91.3% of the initial weight, and the GQDs were totally gone. The GQDs/Fe3O4 1–1 composite retained about 58.2% of the initial weight, implying the actual ratio of GQDs to Fe3O4 in the GQDs/Fe3O4 1–1 composite was approximately 1
:
1 in weight, agreeing with the initial amounts of the GQDs and iron precursors. The formation of Fe3O4 was also confirmed using X-ray photoelectron spectroscopy (XPS) and magnetic property measurements. Fig. 2c shows two peaks at 711.2 and 725.1 eV, corresponding to 2p3/2 and 2p1/2 for Fe binding with oxygen.51 Additionally, the spectrum does not contain the charge transfer satellites for Fe 2p3/2 around 720 eV, reflecting the formation of mixed oxides of Fe(II) and Fe(III), such as Fe3O4.52 The magnetic properties of GQDs/Fe3O4 1–1 were also investigated with a vibrating sample magnetometer. Fig. 2d and S2† depict the magnetization curves of GQDs/Fe3O4 1–1 and bare Fe3O4 measured at 300 K. The saturation magnetization values were 1.6 emu g−1 for GQDs/Fe3O4 1–1 and 61.0 emu g−1 for Fe3O4. Both curves exhibited no magnetic hysteresis loops revealing their superparamagnetic characteristics. Due to the non-magnetic GQD portion, the saturation supermagnetization of GQDs/Fe3O4 1–1 was much lower than that of Fe3O4.
For comparison, we also prepared composites of GQDs/Fe3O4 and GO/Fe3O4 with different GQDs to Fe3O4 and GO to Fe3O4 ratios through similar procedures. As shown in Fig. 1d and e, in the GO/Fe3O4 composites, the GO sheets with micrometer lateral sizes were well decorated with a large amount of Fe3O4 NPs on both sides, which is different from the GQDs/Fe3O4 composites. Notably, the as-obtained GQDs/Fe3O4 composites exhibited a much better dispensability in water than bare Fe3O4 nanoparticles, since the Fe3O4 NPs were wrapped by GQDs which have abundant oxygen-containing groups (Fig. 1c). Additionally, as previously mentioned, GQDs and Fe3O4 both exhibit peroxidase-like activities.7,33 Therefore, we foresaw that the GQDs/Fe3O4 composites might have high peroxidase-like activities.
Peroxidase-like activities of the GQDs/Fe3O4 composites
The peroxidase-like activities of the as-prepared GQDs/Fe3O4 composites were evaluated through the catalytic oxidation of TMB in the presence of H2O2. The activities were also compared with those of bare GQDs, Fe3O4 and GO/Fe3O4. As depicted in Fig. S4, ESI,† the color of the reaction solution with the GQDs/Fe3O4 1–1 composite changed more rapidly than with GO/Fe3O4 1–1, GQDs, or Fe3O4 as observed by the naked-eye. These qualitative results indicate that the as-obtained GQDs/Fe3O4 composite had a much higher peroxidase activity than the others under the same reaction conditions.
The activities of the as-prepared composites were measured quantitatively by following the absorbance intensity of the reaction solutions at 652 nm. Fig. 3a compares the absorbance intensities of the reaction products using Fe3O4, GQDs/Fe3O4 and GO/Fe3O4, with GQDs or GO to Fe3O4 ratios of 0.1–10. Obviously, the GQDs/Fe3O4 composites were more active than the GO/Fe3O4 composites, GQDs, GO and Fe3O4. Among the GQDs/Fe3O4 composites, the GQDs/Fe3O4 1–1 composite exhibited the highest catalytic activity, which was about 9 fold higher than GO/Fe3O4 1–1, 22 fold higher than bare Fe3O4, and 25 fold higher than bare GQDs. The activities of the GQDs/Fe3O4 composites were not a simple addition of the activities of the GQDs and Fe3O4, there is a synergistic effect. To confirm this hypothesis, the GQDs and the Fe3O4 NPs (1
:
1 in weight) were mixed together as a control. As shown in Fig. 3b, the mixture of GQDs and Fe3O4 NPs exhibited a much weaker peroxidase-like activity than GQDs/Fe3O4 1–1. The superb peroxidase activity of GQDs/Fe3O4 1–1 suggests that it is possible that during the preparation of GQDs/Fe3O4, the oxygen atoms in the carboxylate groups in the GQDs coordinate to iron ions forming a GQDs/Fe3O4 conjugate, as concluded from the IR results. The formation of the conjugates makes the electron-transfer from the electron rich GQDs to Fe3O4 NPs more efficient. This is consistent with the literature that reports that Fe2+ may play a dominant role in the catalytic peroxidase-like activity of Fe3O4 NPs.7 This might be the partial reason that the as-prepared GQDs/Fe3O4 composites have higher peroxidase-like activities compared to the mixture of GQDs and Fe3O4 NPs, and the individual GQDs and Fe3O4 NPs. In the case of the GO/Fe3O4 composites, due to the large lateral size and the surface oxygen-containing groups, the electron conjugate state of GO is worse than that of GQDs, and thus the electron-transfer from GO to the Fe3O4 NPs that are anchored on its surface is less efficient (Fig. 1d). The GO/Fe3O4 structure also affects its dispensability in aqueous solutions, which may eventually influence its catalysis. Consequently, the GO/Fe3O4 composites show a much lower peroxidase-like catalytic activity than the GQDs/Fe3O4 composites.
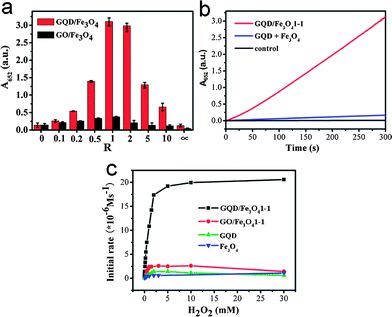 |
| Fig. 3 (a) Comparison of the reaction products of the GQDs/Fe3O4 or GO/Fe3O4 composites with different weight ratios. The reaction was performed with GQDs/Fe3O4 or GO/Fe3O4 composites (10 μg), TMB (0.9 mM) and H2O2 (5 mM) in 1 mL of NaAc (pH 3.5). The ratio of Fe3O4 alone equals 0, while the ratio of GQD or GO alone equals ∝. (b) Time-dependent absorbance changes at 652 nm for the as-prepared composite and its components. (c) The reaction rates of the different materials (10 μg) versus the concentration of H2O2 at 30 °C, with TMB (0.9 mM) in 1 mL of NaAc (pH 3.5). | |
To optimize the peroxidase-like activity of the GQDs/Fe3O4 composite, the initial reaction rate versus the H2O2 concentration was examined. The same experiments were done with GO/Fe3O4, GQDs, and Fe3O4 NPs for comparison. As presented in Fig. 3c, the reaction rate using GQDs/Fe3O4 1–1 increased very fast at low H2O2 concentrations and reached a plateau when the H2O2 concentration was 5 mM. In contrast, with the same H2O2 concentrations, all the other materials showed much slower reaction rates. The peroxidase-like activities of these materials were further characterized by the Michaelis–Menten constant (Km), the maximal reaction velocity (Vmax), and the turnover number (Kcat). The values were obtained according to the Lineweaver–Burk equation, and are summarized in Table 1. The Km value for GQDs/Fe3O4 1–1 is smaller than for the other materials, implying that GQDs/Fe3O4 1–1 has a higher affinity for H2O2. The higher Vmax value for GQDs/Fe3O4 1–1 indicates the synergistic effect on the peroxidase-like activity between GQDs and Fe3O4 NPs. However, the Kcat value for GQDs/Fe3O4 1–1 is lower than that for HRP using the same amount of HRP and GQDs/Fe3O4 1–1. In addition, the GQDs/Fe3O4 1–1 composite shows a better affinity, and a higher Vmax with the other substrate TMB (Fig. S5 and Table S1, ESI†).
Table 1 Comparison of the apparent kinetic parameters Michaelis–Menten constant (Km) and the maximum reaction rate (Vmax) of the different materials (H2O2 as the substrate). The turnover number (Kcat) was determined with the same amount of the material or HRP
Material |
Km (mM) |
Vmax (μM min−1) |
Kcat (mM min−1 g−1) |
GQDs/Fe3O4 1–1 |
0.46 |
14.08 |
1.4 × 106 |
GO/Fe3O4 1–1 |
1.10 |
4.42 |
4.4 × 105 |
GQDs |
0.62 |
1.42 |
1.4 × 105 |
Fe3O4 |
0.49 |
0.79 |
7.9 × 104 |
HRP |
1.13 |
10.74 |
3.6 × 109 |
Though the turnover number for the GQDs/Fe3O4 1–1 composite is lower than for native HRP, as an inorganic composite, GQDs/Fe3O4 should have a much better stability. To illustrate its thermal stability, GQDs/Fe3O4 1–1 was incubated for 2 h at different temperatures before the catalytic reaction. As shown in Fig. 4a, the activity of GQDs/Fe3O4 1–1 was found to be well maintained from 20 to 80 °C, and the optimal working temperature was around 50 °C. Fig. 4b shows that GQDs/Fe3O4 1–1 retained ∼60% of its initial activity after being reused more than 10 times. It was found that 64% of the initial activity of GQDs/Fe3O4 1–1 remained after more than 40 days storage at 4 °C (Fig. S6, ESI†). Comparably, more than 60% of the activity of HRP was lost under the same conditions. The high activity and stability, combined with easy preparation and low costs, renders the GQDs/Fe3O4 1–1 composite a potent peroxidase mimic for many applications under harsh conditions.
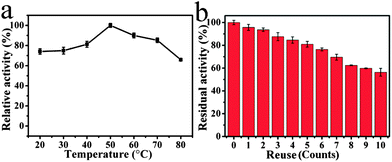 |
| Fig. 4 (a) Effect of temperature on the peroxidase-like activity of GQDs/Fe3O4 1–1; (b) reusability of the GQDs/Fe3O4 1–1 composite. | |
Application of GQDs/Fe3O4 1–1 in the removal of phenolic compounds
To explore the application of GQDs/Fe3O4 1–1 in the removal of phenolic compounds from water, nine compounds with different substituent groups (Table 2) were tested. In parallel, for comparison, the oxidation reactions of these phenolic compounds with the same amount of HRP as a catalyst were also conducted. As summarized in Table 2, for phenol, the removal efficiency with the GQDs/Fe3O4 1–1 composite was higher than with HRP. For some phenolic compounds, such as 3-aminophenol and 2-cholorolphenol, the removal efficiencies with GQDs/Fe3O4 1–1 were comparable to using HRP. For the other phenolic compounds tested, the GQDs/Fe3O4 1–1 composite showed lower removal efficiencies compared to HRP. The removal efficiency for phenol with GQDs/Fe3O4 1–1 was dependent on the concentration of H2O2 and temperature (Fig. 5). The GQDs/Fe3O4 1–1 composite showed a pronounced removal efficiency for phenol from 20 to 70 °C. The oxidation reaction of the phenolic compounds is determined by both steric effects and inductive effects imparted by the substituent on the phenyl ring. In the case of GQDs/Fe3O4 1–1, we believe steric effects possibly dominate the reaction, since the existence of a substituent decreased the reaction rate, and thus the removal efficiency of phenol was the best. Taking these results together, the easy-preparation, high reusability, improved stability, and low cost of the GQDs/Fe3O4 composite give it potential in the treatment of industrial wastewater.
Table 2 Removal efficiencies of phenolic compounds using the same amount of GQDs/Fe3O4 1–1 and HRP
Substrate |
Removal efficiency (%) |
GQDs/Fe3O4 1–1 |
HRP |
Phenol |
80.3% |
67.4% |
2-Methoxyphenol |
26.3% |
97.7% |
4-Methoxyphenol |
46.7% |
96.9% |
3-Aminophenol |
16.9% |
17.3% |
2-Aminophenol |
47.9% |
86.3% |
1,4-Benzenediol |
41.0% |
72.6% |
2-Cholorolphenol |
14.7% |
18.2% |
4-Cholorolphenol |
21.7% |
37.0% |
Catechol |
13.4% |
46.5% |
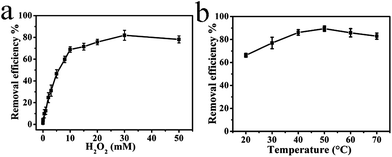 |
| Fig. 5 The phenol removal efficiency of GQDs/Fe3O4 1–1 versus the concentration of H2O2 (a) and temperature (b). | |
Conclusions
A facile approach for the synthesis of GQDs/Fe3O4 composites through co-precipitation was developed. The peroxidase-like activities of the as-obtained GQDs/Fe3O4 composites were explored and compared with those of GQDs, Fe3O4 nanoparticles, GO/Fe3O4 composites, and HRP. It was illustrated that the GQDs/Fe3O4 1–1 composite showed a much higher activity compared to the corresponding GO/Fe3O4 composite or its individual components. The high peroxidase-like activity of GQDs/Fe3O4 1–1 can be attributed to the unique properties of GQDs and the synergistic effect of GQDs and Fe3O4. Due to its high catalytic activity, good stability and reusability, the application of the GQDs/Fe3O4 1–1 composite for the removal of phenolic compounds from aqueous solutions was explored. We demonstrated that the as-obtained GQDs/Fe3O4 1–1 composite exhibited better or comparable catalytic efficiencies for some phenolic compounds compared to HRP. These results indicate that the GQDs/Fe3O4 1–1 composite is a potent peroxidase mimic that holds great promise for many catalytic applications.
Notes
The authors declare no competing financial interests.
Acknowledgements
We thank the National Science foundation of China (no. 91123011, 90923041, 31070742), the state key laboratory of bioreactor engineering (no. 2060204), 111 Project (no. B07023), the Science and Technology Commission of Shanghai Municipality (no. 11DZ2260600 and 12nm0503500), and the National “863” Program of China (no. 2012AA022603) for the financial support of this work.
References
- A. M. Klibanov, T. M. Tu and K. P. Scott, Science, 1983, 221, 259–261 CAS.
- M. Iwaoka and S. Tomoda, J. Am. Chem. Soc., 1994, 116, 2557–2561 CrossRef CAS.
- S. A. Borchardt, E. J. Allain, J. J. Michels, G. W. Stearns, R. F. Kelly and W. F. Mccoy, Appl. Environ. Microbiol., 2001, 67, 3174–3179 CrossRef CAS PubMed.
- H. Wei and E. Wang, Chem. Soc. Rev., 2013, 42, 6060–6093 RSC.
- X. Hu, J. Liu, S. Hou, T. Wen, W. Liu, K. Zhang, W. He, Y. Ji, H. Ren, Q. Wang and X. Wu, Sci. China: Phys., Mech. Astron., 2011, 54, 1749–1756 CrossRef PubMed.
- J. Xie, X. Zhang, H. Wang, H. Zheng and Y. Huang, TrAC, Trends Anal. Chem., 2012, 39, 114–129 CrossRef CAS PubMed.
- L. Gao, J. Zhuang, L. Nie, J. Zhang, Y. Zhang, N. Gu, T. Wang, J. Feng, D. Yang, S. Perrett and X. Yan, Nat. Nanotechnol., 2007, 2, 577–583 CrossRef CAS PubMed.
- H. Wei and E. Wang, Anal. Chem., 2008, 80, 2250–2254 CrossRef CAS PubMed.
- F. Yu, Y. Huang, A. J. Cole and V. C. Yang, Biomaterials, 2009, 30, 4716–4722 CrossRef CAS PubMed.
- X. Cao and N. Wang, Analyst, 2011, 136, 4241–4246 RSC.
- X. Zhang, S. Gong, Y. Zhang, T. Yang, C. Wang and N. Gu, J. Mater. Chem., 2010, 20, 5110–5116 RSC.
- K. N. Chaudhari, N. K. Chaudhari and J. Yu, Catal. Sci. Technol., 2012, 2, 119–124 CAS.
- S. Wang, W. Chen, A. Liu, L. Hong, H. Deng and X. Lin, ChemPhysChem, 2012, 13, 1199–1204 CrossRef CAS PubMed.
- M. Ma, Y. Zhang and N. Gu, Colloids Surf., A, 2011, 373, 6–10 CrossRef CAS PubMed.
- J. Mu, Y. Wang, M. Zhao and L. Zhang, Chem. Commun., 2012, 48, 2540–2542 RSC.
- J. Yin, H. Cao and Y. Lu, J. Mater. Chem., 2012, 22, 527–534 RSC.
- F. Natalio, R. André, A. F. Hartog, B. Stoll, K. P. Jochum, R. Wever and W. Tremel, Nat. Nanotechnol., 2012, 7, 530–535 CrossRef CAS PubMed.
- R. André, F. Natálio, M. Humanes, J. Leppin, K. Heinze, R. Wever, H.-C. Schröder, W. E. G. Müller and W. Tremel, Adv. Funct. Mater., 2011, 21, 501–509 CrossRef.
- W. Chen, J. Chen, Y. Feng, L. Hong, Q. Chen, L. Wu, X. Lina and X. Xia, Analyst, 2012, 137, 1706–1712 RSC.
- H. Deng, W. Shen, Y. Peng, X. Chen, G. Yi and Z. Gao, Chem.–Eur. J., 2012, 18, 8906–8911 CrossRef CAS PubMed.
- L. Zhang, Y. Zhai, N. G. D. Wen and S. Dong, Electrochem. Commun., 2008, 10, 1524–1526 CrossRef CAS PubMed.
- M. I. Kim, Y. Ye, B. Y. Won, S. Shin, J. Lee and H. G. Park, Adv. Funct. Mater., 2011, 21, 2868–2875 CrossRef CAS.
- C. Liu and W. Tseng, Anal. Chim. Acta, 2011, 703, 87–93 CrossRef CAS PubMed.
- K. S. Park, M. I. Kim, D.-Y. Cho and H. G. Park, Small, 2011, 7, 1521–1525 CrossRef CAS PubMed.
- A. Singh, S. Patra, J.-A. Lee, K. H. Park and H. Yang, Biosens. Bioelectron., 2011, 26, 4798–4803 CrossRef CAS PubMed.
- Z. Chen, J.-J. Yin, Y. Zhou, Y. Zhang, L. Song, M. Song, S. Hu and N. Gu, ACS Nano, 2012, 6, 4001–4012 CrossRef CAS PubMed.
- J. Zhang, J. Zhuang, L. Gao, Y. Zhang, N. Gu, J. Feng, D. Yang, J. Zhu and X. Yan, Chemosphere, 2008, 73, 1524–1528 CrossRef CAS PubMed.
- S. Zhang, X. Zhao, H. Niu, Y. Shi, Y. Cai and G. Jiang, J. Hazard. Mater., 2009, 167, 560–566 CrossRef CAS PubMed.
- N. Wang, L. Zhu, D. Wang, M. Wang, Z. Lin and H. Tang, Ultrason. Sonochem., 2010, 17, 526–533 CrossRef CAS PubMed.
- M. Zhu and G. Diao, J. Phys. Chem. C, 2011, 115, 18923–18934 CAS.
- H. Wang and Y. Huang, J. Hazard. Mater., 2011, 191, 163–169 CrossRef CAS PubMed.
- X. Sun, S. Guo, C.-S. Chung, W. Zhu and S. Sun, Adv. Mater., 2013, 25, 132–136 CrossRef CAS PubMed.
- Y. Song, K. Qu, C. Zhao, J. Ren and X. Qu, Adv. Mater., 2010, 22, 2206–2210 CrossRef CAS PubMed.
- C. Peng, B. Jiang, Q. Liu, Z. Guo, Z. Xu, Q. Huang, H. Xu, R. Tai and C. Fan, Energy Environ. Sci., 2011, 4, 2035–2040 CAS.
- M. Liu, H. Zhao, S. Chen, H. Yu and X. Quan, ACS Nano, 2012, 6, 3142–3151 CrossRef CAS PubMed.
- H. Chen, Y. Li, F. Zhang, G. Zhang and X. Fan, J. Mater. Chem., 2011, 21, 17658–17661 RSC.
- Y. Guo, L. Deng, J. Li, S. Guo, E. Wang and S. Dong, ACS Nano, 2011, 5, 1282–1290 CrossRef CAS PubMed.
- P. Yang, S. Jin, Q. Xu and S. Yu, Small, 2013, 9, 199–204 CrossRef CAS PubMed.
- Y. Tao, Y. Lin, Z. Huang, J. Ren and X. Qu, Adv. Mater., 2013, 25, 2594–2599 CrossRef CAS PubMed.
- J. Su, M. Cao, L. Ren and C. Hu, J. Phys. Chem. C, 2011, 115, 14469–14477 CAS.
- Y. Dong, H. Zhang, Z. U. Rahman, L. Su, X. Chen, J. Hu and X. Chen, Nanoscale, 2012, 4, 3969–3976 RSC.
- X. Liu, H. Zhu and X. Yang, Talanta, 2011, 87, 243–248 CrossRef CAS PubMed.
- Y. Ye, T. Kong, X. Yu, Y. Wu, K. Zhang and X. Wang, Talanta, 2012, 89, 417–421 CrossRef CAS PubMed.
- J. Shen, Y. Zhu, X. Yang and C. Li, Chem. Commun., 2012, 48, 3686–3699 RSC.
- K. A. Ritter and J. W. Lyding, Nat. Mater., 2009, 8, 235–242 CrossRef CAS PubMed.
- X. Zhou, Y. Zhang, C. Wang, X. Wu, Y. Yang, B. Zheng, H. Wu, S. Guo and J. Zhang, ACS Nano, 2012, 6, 6592–6599 CrossRef CAS PubMed.
- Y. Zhang, C. Wu, X. Zhou, X. Wu, Y. Yang, H. Wu, S. Guo and J. Zhang, Nanoscale, 2013, 5, 1816–1819 RSC.
- J. Zhang, H. Yang, G. Shen, P. Cheng, J. Zhang and S. Guo, Chem. Commun., 2010, 46, 1112–1114 RSC.
- X. Zhou, J. Zhang, H. Wu, H. Yang, J. Zhang and S. Guo, J. Phys. Chem. C, 2011, 115, 11957–11961 CAS.
- J. L. Gómez, A. Bódalo, E. Gómez, J. Bastida, A. M. Hidalgo and M. Gómez, Enzyme Microb. Technol., 2006, 39, 1016–1022 CrossRef PubMed.
- A.-L. Morel, S. I. Nikitenko, K. Gionnet, A. Wattiaux, J. Lai-Kee-Him, C. Labrugere, B. Chevalier, G. Deleris, C. Petibois, A. Brisson and M. Simonoff, ACS Nano, 2008, 2, 847–856 CrossRef CAS PubMed.
- X. Teng, D. Black, N. J. Watkins, Y. Gao and H. Yang, Nano Lett., 2003, 3, 261–264 CrossRef CAS.
Footnote |
† Electronic supplementary information (ESI) available: AFM images of graphene oxide (GO) and graphene quantum dots (GQDs); hysteresis loops of Fe3O4; TEM image of Fe3O4 NPs; photograph of the products formed after the oxidation of TMB; reaction rates for the different materials versus the concentration of TMB; comparison of the storage stability of the GQDs/Fe3O4 1–1 and HRP; comparison of the apparent kinetic parameters Michaelis constant (Km) and the maximum reaction rate (Vmax) for the different materials (TMB as a substrate). See DOI: 10.1039/c3ra44709j |
|
This journal is © The Royal Society of Chemistry 2014 |