DOI:
10.1039/C3RA43803A
(Paper)
RSC Adv., 2014,
4, 3643-3652
Dendrimer-based multilayer nanocarrier for potential synergistic paclitaxel–doxorubicin combination drug delivery†
Received
20th July 2013
, Accepted 24th October 2013
First published on 28th October 2013
Abstract
A unimolecular micelle-like nanocarrier (PPDP) based on poly(amidoamine) (PAMAM) dendrimers was synthesized to develop combined drug delivery systems for intensifying the therapeutic effect on tumors. The carrier was designed as a dual drug container with oligo-poly(ε-caprolactone) (PCL) and PEGylated amidoamine dendrons as the hydrophobic and hydrophilic domains for each molecule. PPDP was characterized with a size of 10–20 nm, indicating that it mostly remained in the single molecular state rather than in aggregates. Based on PPDP, two drug delivery systems of PPDP-enc-DOX + PTX and PPDP-coj-DOX-enc-PTX were constructed. PPDP-enc-DOX + PTX was prepared by physically encapsulating doxorubicin (DOX) and paclitaxel (PTX) in the different layers of the PPDP, while PPDP-coj-DOX-enc-PTX was fabricated by conjugating DOX through the pH-sensitive linkage on the exterior and embedding PTX in the interior of the PPDP. The drug loading or conjugating and the drug release behaviors were tested. The cytotoxicity, the cell uptake and the intracellular distribution of PPDP-enc-DOX + PTX and PPDP-coj-DOX-enc-PTX were measured in vitro. For both of the systems, the release of PTX was achieved in a sustained manner during 48 h in vitro, while for the PPDP-coj-DOX-enc-PTX system, the liberation of DOX could be controlled. PPDP-enc-DOX + PTX and PPDP-coj-DOX-enc-PTX both showed the ability to simultaneously deliver two drugs into the same tumor cells and showed higher cytotoxicity toward MCF-7/ADR and MCF-7 cells than free DOX or PTX alone due to the synergistic effect of the drugs. Notably, PPDP-enc-DOX + PTX showed a better inhibition effect on MCF-7/ADR cells as it could deliver DOX into the nucleus, while PPDP-coj-DOX-enc-PTX had the stronger cytotoxicity to MCF-7 cells due to the steady sustained release manner of the two drugs.
Introduction
Currently, chemotherapy for cancer remission remains a tremendous challenge because of multiple drug resistance (MDR), which mainly originates from overexpressed drug efflux transporters such as p-glycoprotein in cancer cells, enhanced drug metabolism, the self-repairing ability of cancer cells and inevitable drug-induced side effects.1,2 One approach to address such issues is to develop drug delivery systems with either multi-drug loading or conjugating for combinational chemotherapy, which offers the advantages of enhancing therapeutic efficacy, decreasing side effects, precisely exposing drugs to the target and retarding the development of cancer chemoresistance due to the synergistic or combined effect of different drugs. Several examples have been reported to co-encapsulate or conjugate different chemotherapeutic agents or siRNAs together with an anticancer drug in a single delivery system, including polymeric nanoparticles,3 mesoporous silica nanoparticles,4 liposomes and plasmid DNA hydrogels,5 to improve the drug sensitivity and achieve a synergistic therapeutic outcome. For example, Wang et al. developed a triblock polymer-based micelleplex system with PCL as the hydrophobic core, polyethylene glycol (PEG) as the hydrophilic corona and poly(2-aminoethyl ethylene phosphate) (PPEEA) as the cationic shell. Such micellar nanoparticles were proven to have the ability to co-deliver siRNA and PTX into the same tumor cells both in vitro and in vivo and showed a synergistic tumor suppression effect in the MDA-MB-435s xenograft murine mode.6 Nguyen and coworkers reported a cisplatin-conjugated DOX-encapsulated nanoparticle based on the lipid-templated polymercaged nanobin platform.7 This system, with a high loading capacity of DOX inside, could deliver both drugs to the cancer cells, leading to highly synergistic potency of the drugs and enhanced therapeutic efficacy. Zhang et al. incorporated DOX and camptothecin (CPT) with proportional control over drug loading into polymeric nanoparticles. These dual-drug loaded nanoparticles displayed higher cytotoxicity to MDA-MB-435 breast cancer cells in vitro.2 Despite these progresses, multi-drug delivery by a single vehicle still remains less explored and clarification of the mechanism of the synergistic effect is limited. Therefore, there is urgent demand to develop combined drug delivery systems for intensifying the cytotoxicity to tumor cells, minimizing the toxicity to the normal tissues and reducing MDR of the chemotherapeutic agents.8
Dendritic architectures, as the most pervasive topologies in biological systems with various dimensional scales,9 are more optimal molecular level nanostructures than linear polymers to create multidrug delivery carriers. The multi-exterior groups and interior cavities of dendritic molecules afford the carriers with the abilities of encapsulating/conjugating different drug molecules and targeting/solubilizing moieties on the same frame. The “dendritic effect”, which originates from the unique topology structures, may lead to their interesting biological functions, for example, the nanoglobular contrast agents from poly(L-lysine) dendrimers conjugated with a large number of Gd(III) chelates show size-dependent pharmacokinetics,10,11 and the well-defined molecular structures of such molecules endow the carriers with reproducible properties, either in preparations or in biological applications.
DOX and PTX, with different solubility features, are commonly used anticancer agents in clinical settings nowadays, especially for treating various types of aggressive metastatic breast cancers.12 However, a fatal side effect of DOX derives from its systemic toxicity, especially cardiotoxicity when taking higher doses,13 while the limitations of PTX come from its nonspecific toxic effects, its formulation with the emulsifier known as Cremophor EL, the need for special solvents and the development of resistance by cancer cells.14 It was reported that excellent antitumor activity of DOX was observed and tumor inhibition was intensified when using DOX and PTX as co-therapeutic agents.15,16 Hence, taking the advantages of these two drugs, co-delivery by one system is a worthy and meaningful subject for maximizing the efficacy of the individual therapeutic agents.
Herein, we report a new kind of multilayered nanocarrier (PPDP) based on poly(amidoamine) (PAMAM) dendrimers. The carrier was designed as a dual drug container with layered hydrophobic and hydrophilic structures for each molecule, which is distinct from the reported co-delivery vehicles fabricated by the assembly of copolymers,17 modification of hollow mesoporous silica18 and decoration of polymer-caged liposomes.19 Based on PPDP, two delivery systems were constructed either by encapsulating DOX and PTX in the different layers of the carrier, or by conjugating DOX through a pH-sensitive linkage on the exterior and embedding PTX in the interior of the carrier. The properties of PPDP and the obtained drug delivery systems, including two drug loading and release, the capability of co-delivering two different chemotherapeutics to the same cancer cells, the effect on overcoming drug resistance, the cytotoxicity to the tumor cells, the cell uptake and the intracellular distribution were detected by using MCF-7 and MCF-7/ADR cells in vitro.
Results and discussion
An ideal polymeric drug delivery system or biomedical vector needs to have a structure in the nanometer scale and be uniform in size to possess precise, nanoscale-container and/or scaffold properties with high drug loading ability, and to be provided with feasible surface modifiable functionality and reproducible preparation capability.20 Dendrimer-based drug carriers are considered as the best candidates for creating drug delivery systems to meet the requirement for a non-virus vehicle. These systems have the advantages of precise and stable nanostructures, with easily functionalized and multi-peripheral groups. Particularly, they can act as “unimolecular micelles” to mimic the hydrophobic and hydrophilic core shell topology of a regular micelle.21 Such micelles are independent of concentration and temperature, which differs from the conventional micelles of amphiphilic polymers, which have a dynamic nature, and liposomes, which are energetically metastable.9
In the present study, we used fourth generation PAMAM dendrimers as a core, and oligo-PCL and peripherally PEGylated amidoamine dendrons as the hydrophobic and hydrophilic domains to construct a unimolecular micelle-like nanocarrier (Scheme 1). Importantly, the molecular weight of PAMAM–oligo-PCL–OH was controlled carefully in order to afford the carrier with optimum hydrophobic and hydrophilic properties. According to the comparison experiments, the PAMAM–oligo-PCL–OH would mainly show the hydrophilic feature of the PAMAM dendrimers if the molecular weight was less than 40 kDa (each oligo-PCL chain with less than 4 repeating units in theory), leading to difficult separation from PAMAM–OH, while it would exhibit the hydrophobic nature of PCL when the product had a molecular weight of more than 100 kDa (each oligo-PCL chain with over 12 monomer units in theory). As a result, the molecular weight of PAMAM–oligo-PCL–OH was adjusted to 70–80 kDa (each oligo-PCL chain with 8–10 units in theory), which not only provided the desired hydrophobicity but also the hydroxyl functional groups for the next click reaction. Elemental analysis, GPC and 1H NMR measurements were used to characterize the resulting products with the results shown in Fig. S1–S3.†
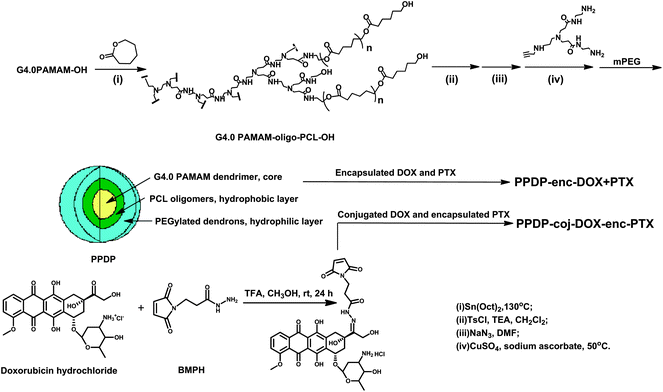 |
| Scheme 1 The synthetic route of PAMAM dendrimer-based multilayer nanocarrier and drug delivery systems. | |
The click reaction was applied to build the outer hydrophilic domain by reacting PAMAM–oligo-PCL–N3, which was converted from PAMAM–oligo-PCL–OH with the amidoamine dendrons having an alkynyl moiety at the focal point. It was found that the commonly used CuBr–PMDETA catalyst for the click reaction was inoperative in our case, mainly due to the hydrophilic feature of the dendritic branches that caused the focal alkyne group to chelate well with Cu2+ ions in organic solvent. In our system, CuSO4–sodium ascorbate was an effective catalyst for the reaction when the solvent ratio of N,N-dimethylformamide (DMF) and H2O was 5
:
1. FT-IR measurement showed that the band of the azide group at 2096 cm−1 disappeared and the bands of amide group at 1650 and 1540 cm−1 greatly intensified (Fig. S5†), indicating a high yield reaction in 24 h.
The final PEGylation for the multi-layered carrier PPDP was accomplished by linking the NHS end group of PEG1000 with the amine moieties of the dendrons. 1H NMR measurement indicated that about 50% of the peripheral amine groups of the dendrons were covered with PEG chains (Fig. S4†).
The TEM image showed that the size of the PPDP was in the range of 10–20 nm (Fig. 1), suggesting that it mostly remained in the molecular state rather than forming unexpected aggregates. This was further evidenced by dynamic light scattering (DLS) measurements, which demonstrated that the particles were monodispersed (Fig. S6†).
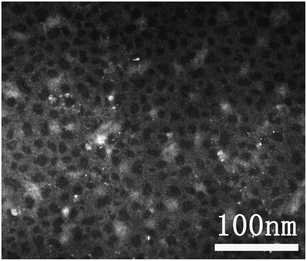 |
| Fig. 1 TEM image of PPDP. | |
For the drug delivery system of PPDP-enc-DOX + PTX, PTX was entrapped in the hydrophobic oligo-PCL layer with an encapsulating efficiency higher than 90%, and DOX was embedded mainly in the outer hydrophilic area with a loading efficiency of more than 70% according to HPLC and UV-vis measurements (Fig. S7†). This might be due to the steric hindrance of the oligo-PCL layer; the interaction of DOX with the amide groups of the amidoamine dendrons via hydrogen bonding22 and the entrapment effect of the PEG chains blocked the entrance of DOX into the central PAMAM core. Such observation is consistent with the report by Kenji Kono et al., who synthesized PEG 550 and 2000-attached PAMAM dendrimers and encapsulated DOX and PTX in the interior. They found that the inner space of the dendrimers might become larger due to the attachment of long PEG chains, and DOX molecules might possibly be solubilized in the surface of the PEG layer.23
The in vitro drug release of PPDP-enc-DOX + PTX was studied in buffer solution at different pHs at room temperature (Fig. 2a and b). PTX displayed palliative and sustained-release properties over 50 h at pH 5.0 and pH 7.4 with a cumulative release amount of 40%, except for an initial burst in the release pattern that appeared up to 2 h with about 10% being released. However, the cumulative release amounts of DOX were 65% at pH 7.4, and nearly 75% at pH 5.0 over an approximately 3 h period, respectively. This may be attributed to the combined effects of (1) the unstable localization of DOX in the outer region due to the weak interactions between them; (2) particularly, the amino groups in the dendritic structures are partly protonated with the decrease of pH,24 leading to the dissociation of DOX because of the structure extension of the carrier; and (3) the lower release amount of DOX at pH 7.4 was derived from the poor solubility of doxorubicin hydrochloride under natural conditions. The same result was reported by Fréchet and coworkers.25 However, the sustained-release property of PTX suggested that the drug molecules were well embedded in the hydrophobic domain surrounded by the outer hydrophilic layer, and the release was accelerated at pH 5.0 due to the partial protonation of the PAMAM dendrimers and dendrons.
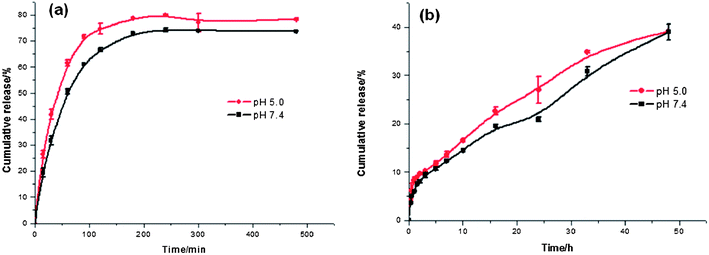 |
| Fig. 2 The drug release profiles at pH 5.0 ( ) and pH 7.4 ( ) of (a) DOX and (b) PTX from PPDP-enc-DOX + PTX in vitro. The data are presented as the mean ± standard deviation (n = 3). | |
In order to better control the release of DOX, PPDP-coj-DOX-enc-PTX was prepared by covalently conjugating DOX on the periphery of the amidoamine dendrons through acyl hydrazone bonds, with a grafting amount of 1.5% (w/w) calculated by UV-vis measurement. Such a low grafting rate may be due to the large coverage of PEG chains that block the reaction of –NH2 on the dendrimer surface with the succinimide group modified in the DOX molecule as an active linker.
We monitored the in vitro drug release of PPDP-coj-DOX-enc-PTX in buffer at different pHs at room temperature. The release of DOX was different from that with PPDP-enc-DOX + PTX (Fig. 3). It showed a well controlled sustained manner and pH-sensitivity, with a release amount of 23% at pH 7.4 and 40% at pH 5.0 over 48 h, indicating the facility of fracturing the hydrazone bonds in an acidic environment. For PTX liberation, it maintained the same palliative and sustained-release behavior, with a release amount of 40% at pH 5.0 over 48 h. These results demonstrate that different drugs can be co-implanted in a PAMAM dendrimer molecule by a combined method of conjugation and encapsulation, and the controlled release of DOX is achieved by breaking the acyl hydrazone bonds in an acidic environment. In such a way, the release of DOX and PTX reaches a balance rate of roughly 1
:
1.
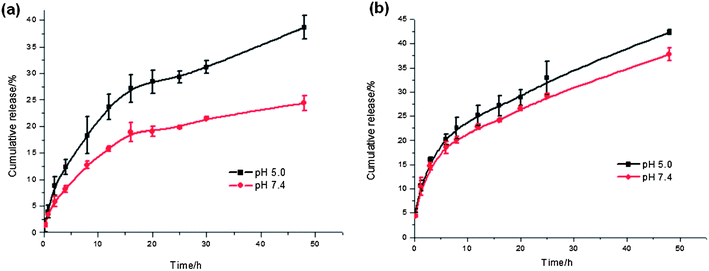 |
| Fig. 3 The drug release profiles of (a) DOX and (b) PTX from PPDP-coj-DOX-enc-PTX in vitro at pH 5.0 ( ) and pH 7.4 ( ). The data are presented as the mean ± standard deviation (n = 3). | |
It should be noticed that for the PPDP-enc-DOX + PTX system, the profiles of DOX release showed a burst manner, which is not ideal for controlled release, while for the system of PPDP-coj-DOX-enc-PTX, the release profile of DOX displayed a controlled sustained manner over 48 h.
The human breast cancer cell line MCF-7 and the drug resistant breast cancer cell line MCF-7/ADR were chosen for the cytotoxicity assays (Fig. 4). For the PPDP-enc-DOX + PTX system, the cell viability of MCF-7 cells treated with PPDP-enc-DOX + PTX was 55%, lower than with free PTX (67%) and free DOX (69%) with the concentration of drugs at 20 μM (DOX + PTX). Notably, the MCF-7/ADR cells maintain low viability throughout with the order being 58% (PPDP-enc-DOX + PTX) < 82% (DOX) < 90% (PTX) when the cells were incubated with PPDP-enc-DOX + PTX, and DOX and PTX individually at the same concentration of 20 μM. For the PPDP-coj-DOX-enc-PTX system (Fig. 5), the cell viability of the MCF-7 cells decreased to 45% as compared to with the PPDP-enc-DOX + PTX which gave a cell viability of 55% under the same conditions.
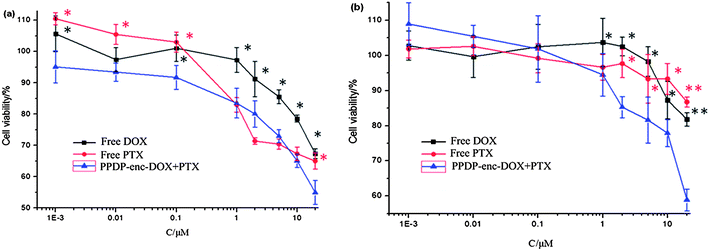 |
| Fig. 4 Cytotoxicity of free DOX ( ), free PTX ( ) and PPDP-enc-DOX + PTX ( ) to (a) MCF-7 cells and (b) MCF-7/ADR cells over 48 h. The data are presented as the mean ± standard deviation (n = 3). *p < 0.05; **p < 0.01, significance represents free DOX/PTX vs. PPDP-enc-DOX + PTX. | |
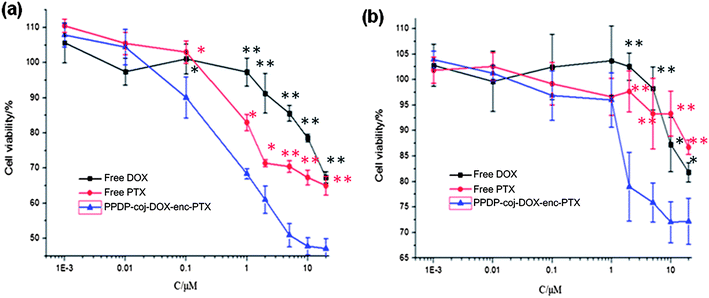 |
| Fig. 5 Cytotoxicity of free DOX ( ), free PTX ( ) and PPDP-coj-DOX-enc-PTX ( ) to (a) MCF-7 cells and (b) MCF-7/ADR cells over 48 h. The data are presented as the mean ± standard deviation (n = 3). *p < 0.05; **p < 0.01, significance represents free DOX/PTX vs. PPDP-coj-DOX-enc-PTX. | |
From the above results, it could be seen that both systems displayed higher cytotoxicity to the MCF-7 and MCF-7/ADR cells than DOX and PTX alone, which corresponds to the results reported by Shuai and coworkers.26 Such results were also reported by Dennis E. Discher et al. They co-delivered DOX and PTX using biodegradable polymersomes and found that the dual drug combination showed a higher maximum tolerated dose and shrunk the tumors more effectively than the free drug cocktail.15 Comparing the two systems, it is noteworthy that (1) PPDP-enc-DOX + PTX with two encapsulated drugs displays remarkable inhibition of the drug resistant MCF-7/ADR cells, indicating that it has great superiority in overcoming MDR by simultaneously co-delivering DOX and PTX to the same tumor cells; and (2) PPDP-coj-DOX-enc-PTX showed an even better inhibition effect on MCF-7 cells than PPDP-enc-DOX + PTX. This might be due to the palliative and sustained-release manner of the two drugs, thus leading to the enhanced cytotoxicity.27
To understand the synergistic effect, we further analyzed the cellular uptake and the intracellular distribution of the drugs in MCF-7 and MCF-7/ADR cells incubated with PPDP-enc-DOX + PTX, PPDP-coj-DOX-enc-PTX, free DOX and free PTX for 2 h or 4 h, respectively (Fig. 6 and S8†). DOX and PPDP-enc-DOX + PTX can be evaluated from the characteristic fluorescence of DOX (excitation/emission = 488 nm/580 nm). To visualize PTX, we attached a fluorescent probe, fluorescein isothiocyanate (FITC) (excitation/emission = 488 nm/530 nm), to the molecule. It was observed that PPDP-enc-DOX + PTX could deliver DOX into the nucleolus and allow PTX to be mainly distributed in the cytoplasm after 2 h of incubation (Fig. S8†). Remarkably, for the MCF-7/ADR cells, PTX was located in the cytoplasm, but free DOX was not found in the nucleolus. However, the majority of DOX encapsulated in PPDP-enc-DOX + PTX was accumulated in the nucleolus. The same phenomenon was also found after 4 h of incubation for both of the cells (Fig. 6).
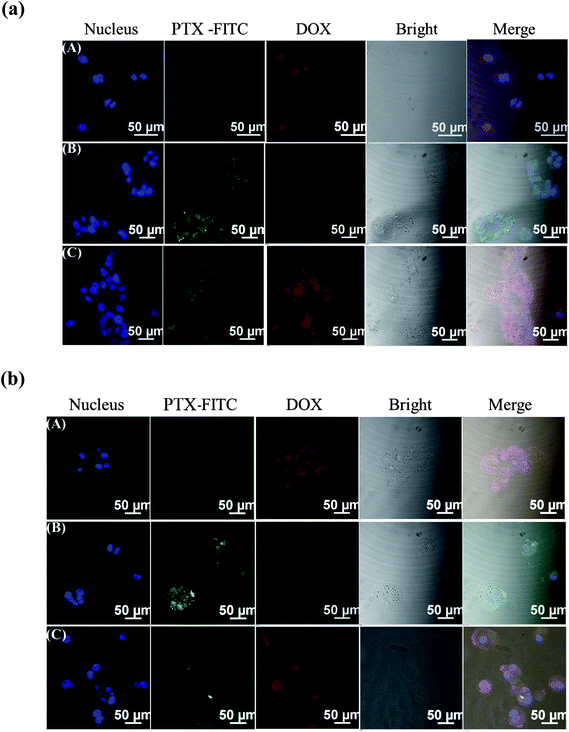 |
| Fig. 6 Confocal images of the MCF-7/ADR cells incubated with 20 mM (A) free DOX, (B) free PTX and (C) PPDP-enc-DOX + PTX, respectively, for (a) 2 h and (b) 4 h. For each panel, the images from left to right show the cells with nuclear staining by Hoechst 33258, PTX–FITC, DOX fluorescence, bright field and overlays of the images (scale bar, 50 μm). | |
For the PPDP-coj-DOX-enc-PTX system, it was found that little DOX could be observed in the nucleolus of the MCF-7 cells when the cells were incubated with PPDP-coj-DOX-enc-PTX for 2 h at pH 5.0 and most of the DOX was present in the cytoplasm (Fig. 7a) because of the ineffective cleavage of the acyl hydrazone bonds. However, DOX was found in the nucleolus of MCF-7 cells after 4 h of incubation, indicating the release of DOX due to the fracture of chemical bonds under acidic condition (Fig. 7b).
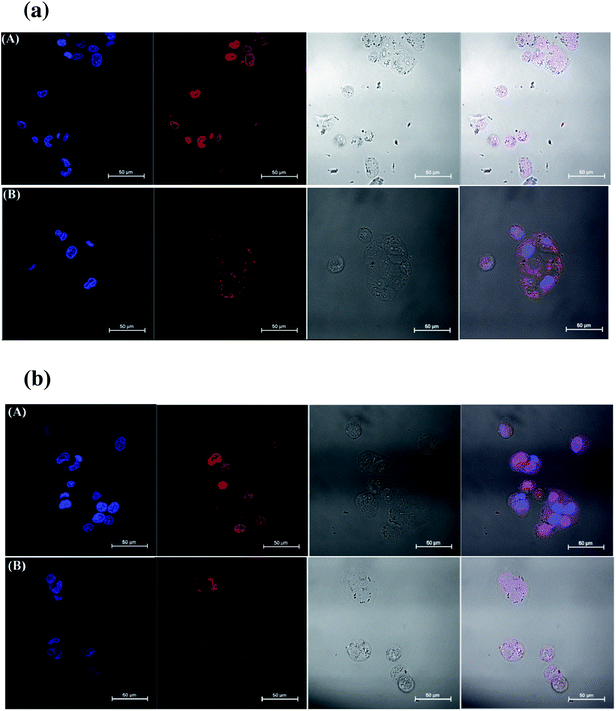 |
| Fig. 7 Confocal images of the MCF-7 cells incubated with 20 mM (A) free DOX and (B) PPDP-coj-DOX-enc-PTX, respectively, for (a) 2 h and (b) 4 h. For each panel, the images from left to right show the cells with nuclear staining by Hoechst 33258, DOX fluorescence, bright field and overlays of the images (scale bar, 50 μm). | |
It is widely known that DOX is a kind of antitumor antibiotic that restrains the synthesis of nucleic acids such as DNA and RNA in the nucleoli of cancer cells,28 while PTX binds to the N-terminal 31 amino acids of the beta-tubulin subunits of microtubules in the cytoplasm.29 Gustafson et al. studied the mechanism of the synergistic effect of DOX and TAX in vivo.16 They found that the combined treatment of DOX and TAX altered the pharmacokinetics of both agents. The interesting part was that variation of the plasma pharmacokinetics of DOX was not found, while the drug level in other tissues changed dramatically. In our case, the detailed mechanism of two drugs co-delivered by a dendrimer-based carrier is not clear now, but such unimolecular micelle drug delivery systems will undoubtedly provide a potential approach for future breast cancer therapy.
Conclusion
Two PAMAM dendrimer-based drug delivery systems with multilayered structures were constructed either by physically encapsulating the two drugs DOX and PTX or by chemically bonding a drug in the outer structure. Both PPDP-enc-DOX + PTX and PPDP-coj-DOX-enc-PTX showed higher cytotoxicity to MCF-7/ADR and MCF-7 cells than free DOX and PTX alone, due to the synergistic paclitaxel–doxorubicin combination drug delivery. For the PPDP-enc-DOX + PTX system, notably, it displayed an inhibition effect on MDR because the DOX could enter into the nucleus of the MCF-7/ADR cells. As for the PPDP-coj-DOX-enc-PTX system, especially, it showed stronger cytotoxicity to MCF-7 cells due to the steady, sustained release manner of the two drugs.
Experiment section
Materials and characterization
DOX and PTX were purchased from Duodian chemical cooperation (Nanjing, China). ε-Caprolactone, sodium ascorbate, copper bromide, N,N,N′,N′,N′′-pentamethyldiethylene-triamine (PMDETA), fluorescein isothiocyanate (FITC) and sulforhodamine B (SRB) were purchased from Sigma-Aldrich corporation (St. Louis, MO, USA). Methoxy PEG succinimidyl carbonate ester (mPEG-NHS, Mw = 1000) was purchased from Biomatrik Inc. (Jiaxing, China). Other reagents were purchased from Beijing Chemical Reagents (Beijing, China). ε-Caprolactone was distilled after being stirred with calcium hydride for 6 h and stored at room temperature. P-toluensulfonyl chloride was recrystallized in a mixed solvent of acetic ether–petroleum ether before use. Copper bromide was washed with glacial acetic acid, methane and acetone stepwise and dried before use. Other reagents were used as received, and the solvents were purified according to the general procedures before use. 1H NMR spectra were recorded on a ARX 400 MHz (Bruker) or a Mercury Plus 300 MHz (Varian) spectrometer. Low-resolution mass spectra were measured on an APEX IV Fourier transform electrospray mass spectrometry (Bruker). FT-IR spectra were measured on a VECTOR22 Fourier transform infrared spectrometer (Bruker). UV-vis spectra were measured on a CARY 1E absorption spectrophotometer (Varian). Fluorescence spectra were measured on a Hitachi F-4500 spectrometer. TEM images were taken on an H-9000NAR, operating at an acceleration voltage of 100 kV. Dynamic light scattering (DLS) experiments were conducted on a commercialized spectrometer (Brookhaven Inc., Holtsville, NY) equipped with a BI-200SM goniometer and a BI-TurboCorr digital correlator. HPLC was performed on a HP1100 chromatogram (Agilent) with an Eclipse XDB-C18 column (4.6 × 250 mm). Gel permeation chromatography (GPC) was performed on a Waters chromatogram with tetrahydrofuran as the solvent and polystyrene as the standard. Laser scanning confocal images were taken on a Nikon A1R-si microscope.
Synthesis of hydroxyl-terminated PAMAM dendrimers and amino-amine dendrons
Hydroxyl-terminated PAMAM dendrimers (PAMAM–OH, G4.0) were synthesized according to a previous report.30 The products were dialyzed for 4 days and freeze-dried before used. 1H NMR (D2O, 300 MHz, ppm): δ2.25–2.40 (m, 2H), δ2.45–2.55 (m, 2H), δ2.68–2.74 (m, 2H), δ3.10–3.20 (t, 2H), δ3.45–3.55 (t, 2H).
The first generation amino-amine dendrons (D1.0) with alkyne as the core were synthesized as described before.31 D1.0: 1H NMR (CDCl3, 300 MHz, ppm): δ1.46 (s, 4H), δ2.24 (t, 1H), δ2.38 (t, 4H), δ2.83 (q, 8H), δ3.29 (q, 4H), δ3.43 (d, 2H), δ7.30 (s, 2H). FTMS (m/z: 284.1).
Synthesis of PAMAM–oligo-PCL
PAMAM–oligo-PCL was synthesized by ring opening polymerization. PAMAM–OH G4.0 (1.04 g, 73 μmol) and ε-caprolactone (6.38 g, 56 mmol) were put into a round glass flask followed by exhausting for 20 minutes. Then the exhausting–refilling process was repeated three times and the solution was heated up to 140 °C to make the PAMAM–OH completely dissolve in ε-caprolactone. The solution was cooled to 110 °C and one drop of Sn(Oct)2 was added immediately. The exhausting–refilling process was repeated three times and the solution was stirred at 120 °C for 24 h. The reaction mixture was then cooled to room temperature and CHCl3 was added to dissolve the products. Linear and star polymers were isolated by fractionation in CHCl3 and petroleum. The solution was separated into two layers. The lower layer was then precipitated into diethyl ether and a light yellow polymer was obtained. GPC and elemental analysis were used to determine the molecular weight of the products. Yield: 30%. 1H NMR (CDCl3, 300 MHz, ppm): δ1.30–1.44 (m, CH2), δ1.50–1.72 (m, CH2), δ2.17–2.23 (t, CH), δ2.25–2.40 (m, CH2), δ2.45–2.55 (m, –CH2CH2N<), δ2.68–2.74 (m, –NCH2CH2CO–), δ3.10–3.20 (t, –CONHCH2CH2–), δ3.60–3.70 (t, CH2OH), δ4.00–4.15 (m, CH2).
Synthesis of tosyl-terminated PAMAM–oligo-PCL
The terminal group of PAMAM–PCL–OH was converted into a tosyl group. Briefly, PAMAM–PCL–OH (4.00 g, 50 μmol) was put into a 100 mL round glass flask and dissolved in 50 mL CHCl2. After cooling, TsCl (8.45 g, 44.3 mmol) was added into the solution followed by adding 3 mL triethylamine. The exhausting–refilling process was repeated three times and the reaction solution was heated up to room temperature for further stirring for 24 h. The reaction solution was washed with water three times and then precipitated into diethyl ether to remove unreacted TsCl. Yield: 70%, 1H NMR (CDCl3, 300 MHz, ppm): δ1.30–1.44 (m, CH2), δ1.50–1.72 (m, CH2), δ2.17–2.23 (t, CH), δ2.25–2.40 (m,CH2), δ2.44 (s, CH3), δ4.00–4.15 (m, CH2), δ7.35 (d, CH), δ7.81 (d, CH).
Synthesis of N3-terminated PAMAM–oligo-PCL
PAMAM–oligo-PCL–OTs (4.04 g, 44.9 μmol) was dissolved in 20 mL DMF. Then at room temperature, NaN3 (4.84 g, 74.4 mmol) was added into the solution and the solution was stirred for 24 h. The reaction solvent was then evaporated and the residue was re-dissolved into CH2Cl2. After filtration to remove white solid, the solution was washed with water three times to remove unreacted NaN3. Yield: 65%. 1H NMR (CDCl3, 300 MHz, ppm): δ1.30–1.44 (m, CH2), δ1.50–1.72 (m, CH2), δ2.17–2.23 (t, CH), δ2.25–2.40 (m, CH2), δ3.30 (t, –CH2–N3), δ4.00–4.15 (m, CH2). FT–IR (KBr, cm−1): 3440 (νN–H), 2946 (νC–H), 2096 (νN
N), 1730 (νC
O), 1650 (νCO–NH).
Synthesis of PAMAM–oligo-PCL–D1.0
The alkyne-cored dendrons were conjugated with N3-terminated PAMAM–oligo-PCL by the click reaction. Take D1.0 as an example. PAMAM–PCL–N3 (0.20 g, 2.5 μmol) and D1.0 (104.5 mg, 0.37 mmol) were dissolved in 10 mL DMF. 2 mL aqueous solution containing sodium ascorbate (81.27 mg, 0.41 mmol) and CuSO4·5H2O (40 mg, 0.16 mmol) was added into the DMF solution slowly and a repeated exhausting–refilling process was performed three times. The reaction system was allowed to react for 24 h at 50 °C in a sealed environment. The crude product was transferred to a MWCO 2000 dialysis bag and dialyzed against water for two days followed by lyophilizing. Yield: 50%. 1H NMR (D2O, 400 MHz, ppm): δ1.30–1.44 (m, CH2), δ1.50–1.72 (m, CH2), δ2.17–2.23 (t, CH), δ2.25–2.40 (m,CH2), δ2.45–2.55 (m, –CH2CH2N<), δ2.68–2.74 (m, –NCH2CH2CO–), δ3.10–3.20 (t, –CONHCH2CH2–), δ4.00–4.15 (m, CH2). FT-IR (KBr, cm−1): 3440 (νN–H), 2946 (νC–H), 1730 (νC
O), 1650 (νCO–NH).
Synthesis of PAMAM–oligo-PCL–D1.0–PEG (PPDP)
The final step was linking PEG chains to the periphery of the dendrons. PAMAM–oligo-PCL–D1.0 (30 mg) was dissolved in 6 mL water, and then 1 mL mPEG–NHS (41 mg) aqueous solution was added dropwise and stirred overnight. The crude product was transferred to a MWCO 2000 dialysis bag and dialyzed against water for two days followed by lyophilization. Yield: 90%. 1H NMR (CDCl3, 300 MHz, ppm): δ1.30–1.44 (m, CH2), δ1.50–1.72 (m, CH2), δ2.17–2.23 (t, CH), δ2.25–2.40 (m, CH2), δ3.36–3.38 (s, CH3), δ3.50–3.74 (s, –OCH2CH2O–), δ4.00–4.15 (m, CH2), δ4.20 (m, –NHCOOCH2CH2–).
Synthesis of PTX–FITC
FITC was chemically conjugated to PTX for taking confocal images. Briefly, PTX (9.85 mg, 11.5 μmol) and FITC (6.83 mg, 17.6 μmol) were dissolved in 6 mL DMSO and stirred at 90 °C for 2 h. The crude product was transferred to a MWCO 2000 dialysis bag and dialyzed against water until the dialysis fluid no longer turned yellow. The suspension in the dialysis bag was then centrifuged and a bright yellow solid was obtained.
Synthesis of PPDP-enc-DOX + PTX – PTX and DOX loading
69.52 mg PPDP and 6.96 mg PTX were dissolved in 10 mL CHCl3. Then the solvent was evaporated and dried out at 37 °C overnight. 6.94 mg of DOX hydrochloride was first dissolved in 70 mL deionized water and then added into PPDP–PTX residue and subjected to ultrasound for 5 minutes. Then the solution was centrifuged. The undissolved PTX was used to determine the encapsulating proportion of PTX by HPLC. The solution was transferred to a dialysis bag (MWCO 8000) and dialyzed twice to remove unencapsulated DOX. The encapsulation percentage of DOX was determined by a UV-vis scanning spectrophotometer at 480 nm in deionized water.
Synthesis of PPDP-coj-DOX-enc-PTX
DOX was activated using BMPH using the same procedures as reported by Chilkoti and coworkers.32 Briefly, DOX–HCl (50.4 mg, 86.9 μmol) and BMPH (19.0 mg, 86.5 μmol) were dissolved in 60 mL anhydrous methanol. Then trifluoroacetic acid (TFA) was added to the solution and the solution was stirred for 24 h in the dark at room temperature. The product was obtained after removal of the solvent. 1H NMR (d6-DMSO, 300 MHz, ppm) (Fig. S1†): δ7.96 (br, DOX, protons of the phenyl ring), δ7.70 (br, DOX, protons of the phenyl group), δ7.08 (s, BMPH, protons of the double bond), δ5.32 (s, –OCHO– of DOX), δ4.60 (s, BMPH, the methylene next to the maleimide group), δ4.05 (m, DOX, the methine next to the hydroquinone), δ3.65 (s, DOX, –OCH3), δ3.23 [m, DOX, combination of the methylene next to the hydrazone linker, –CH(OH)– and –OCH(CH3)CH(OH)– of the tetrahydropyran ring], δ2.73 (d, DOX, the methylene next to the hydroquinone), δ2.55 [br, BMPH, –CH2C(O)NH–], δ2.37 [d, –CH(NH2)–], δ2.06 (d, DOX, one proton of the methylene of the tetrahydropyran ring), δ1.80 (m, the other proton of the methylene of the tetrahydropyran ring), δ1.09 (m, –CH3 in the tetrahydropyran ring). FT-MS (m/z: 709.2).
The activated DOX was dissolved in 20 mL dry methanol and mixed with PPDP (60.84 mg) for 2 days in the dark. The solution was concentrated by rotary evaporation and dialyzed against deionized water for 2 days to remove the unreacted DOX. Lyophilization yielded PPDP–DOX as a dark red powder.
Transmission electron micrograph morphology
A drop of PPDP solution (concentration: 1.0 mg mL−1) was placed on a carbon-coated formvar copper grid. After 2 minutes, the grid was tapped with filter paper to remove the aqueous solution on the surface and air-dried. Negative staining was performed by addition of a drop of 1 wt% solution of uranyl acetate to the copper grid with the sample.
Light scattering measurement
For DLS measurements, the aqueous sample (1 mg mL−1) was passed through a 0.20 μm syringe filter (Sartorius Stedim Biotech, Goettingen, Germany) to remove dust.
PTX and DOX release
Drug release was performed in PBS solution as described before.22,33 Briefly, 3.5 mg PPDP–PTX–DOX was dissolved in 10 mL of pH 5.0 or pH 7.4 PBS solution, respectively. Then the solution was transferred into a MWCO 2000 dialysis bag and dialyzed against PBS solution (containing 0.2% Tween-80) at 37 °C. 1 mL dialysis fluid outside the bag was taken and the same volume of fresh medium was supplied immediately to keep the same volume. The PTX release curve was determined by HPLC and the DOX release curve was determined by a F-4500 spectrometer at 480 nm in deionized water.
Cell culture
In this study, the human breast cancer cell line MCF-7 and the drug resistant human breast cancer cell line MCF-7/ADR were routinely grown in RPMI 1640 medium supplemented by 10% heat-inactivated fetal bovine serum (FBS), 100 U mL−1 penicillin and 100 mg mL−1 streptomycin. The cells were maintained at 37 °C with 5% CO2.
In vitro cytotoxicity assay
Both MCF-7 and MCF-7/ADR cells were used in the cytotoxicity test. MCF-7 or MCF-7/ADR cells were seeded into 96-well culture plates at a density of 5 × 103 cells per well and grown for 24 h. Then free DOX, free PTX and PPDP–DOX–PTX were added into the 96-well culture plates, respectively. The concentration of the drugs was 20 mM. After 48 h, the cell viability was measured using a microplate reader at 540 nm after the SRB staining assay. The following formula was used: Survival% = (A540nm for the treated cells/A540nm for the control cells) × 100%, where the A540nm was the absorbance value. Each assay was repeated 3 times and then dose–effect curves were plotted.
In vitro cellular uptake
Before the experiment, MCF-7 or MCF-7/ADR cells were seeded into chambered coverslips at a density of 4 × 104 cells per well and incubated for 24 h. After adding free DOX, free PTX–FITC, PPDP–DOX, PPDP–PTX–FITC and PPDP–DOX–PTX–FITC to the cells at a total drug concentration of 20 mM, respectively, the cells were incubated for another 2 or 4 h. Afterwards, the cells were washed with cold PBS three times, fixed with 4% (v/v) paraformaldehyde and finally stained with Hoechst 33258. The confocal images were then taken using a Nikon A1R-si microscope.
Statistics analysis
Data are presented as mean ± standard deviation. One-way analysis of variance (ANOVA) was used to determine the significance among groups following the Bonferroni's post-test.
Acknowledgements
This work was supported by the National Natural Science Foundation of China (NSFC 21174005 and 21274004) to Xin-Ru Jia. We thank Dr Ronghua Sun for the help on the cell culture.
References
- H. A. Meng, M. Liong, T. A. Xia, Z. X. Li, Z. X. Ji, J. I. Zink and A. E. Nel, ACS Nano, 2010, 4, 4539–4550 CrossRef CAS PubMed.
- S. Aryal, C. M. J. Hu and L. F. Zhang, Mol. Pharmacol., 2011, 8, 1401–1407 CrossRef CAS PubMed.
- H. T. T. Duong, C. P. Marquis, M. Whittaker, T. P. Davis and C. Boyer, Macromolecules, 2011, 44, 8008–8019 CrossRef CAS.
- Y. F. Zhu, W. J. Meng, H. Gao and N. Hanagata, J. Phys. Chem. C, 2011, 115, 13630–13636 CAS.
- D. Costa, A. J. M. Valente, M. G. Miguel and J. Queiroz, Langmuir, 2011, 27, 13780–13789 CrossRef CAS PubMed.
- T. M. Sun, J. Z. Du, Y. D. Yao, C. Q. Mao, S. Dou, S. Y. Huang, P. Z. Zhang, K. W. Leong, E. W. Song and J. Wang, ACS Nano, 2011, 5, 1483–1494 CrossRef CAS PubMed.
- S. M. Lee, T. V. O'Halloran and S. T. Nguyen, J. Am. Chem. Soc., 2010, 132, 17130–17138 CrossRef CAS PubMed.
- S. Hoffmeyer, O. Burk, O. von Richter, H. P. Arnold, J. Brockmoller, A. Johne, I. Cascorbi, T. Gerloff, I. Roots, M. Eichelbaum and U. Brinkmann, Proc. Natl. Acad. Sci. U. S. A., 2000, 97, 3473–3478 CrossRef CAS.
- S. Svenson and D. A. Tomalia, Adv. Drug Delivery Rev., 2005, 57, 2106–2129 CrossRef CAS PubMed.
- T. L. Kaneshiro and Z.-R. Lu, Biomaterials, 2009, 30, 5660–5666 CrossRef CAS PubMed.
- I. J. Majoros, A. Myc, T. Thomas, C. B. Mehta and J. R. Baker, Biomacromolecules, 2006, 7, 572–579 CrossRef CAS PubMed.
- C. F. Higgins, Nature, 2007, 446, 749–757 CrossRef CAS PubMed.
- X. L. Li, X. W. Lu, H. E. Xu, Z. S. Zhu, H. T. Yin, X. P. Qian, R. T. Li, X. Q. Jiang and B. R. Liu, Mol. Pharmacol., 2012, 9, 222–229 CrossRef CAS PubMed.
- M. Mokni, S. Hamlaoui-Guesmi, M. Amri, L. Marzouki, F. Limam and E. Aouani, Cardiovasc. Toxicol., 2012, 12, 158–165 CrossRef PubMed.
- F. Ahmed, R. I. Pakunlu, A. Brannan, F. Bates, T. Minko and D. E. Discher, J. Controlled Release, 2006, 116, 150–158 CrossRef CAS PubMed.
- D. L. Gustafson, A. L. Merz and M. E. Long, Cancer Lett., 2005, 220, 161–169 CrossRef CAS PubMed.
- W. Chen, F. Meng, R. Cheng and Z. Zhong, J. Controlled Release, 2010, 142, 40–46 CrossRef CAS PubMed.
- Y. Zhao, L.-N. Lin, Y. Lu, S.-F. Chen, L. Dong and S.-H. Yu, Adv. Mater., 2010, 22, 5255–5259 CrossRef CAS PubMed.
- X. B. Xiong and A. Lavasanifar, ACS Nano, 2011, 5, 5202–5213 CrossRef CAS PubMed.
- R. Esfand and D. A. Tomalia, Drug Discovery Today, 2001, 6, 427–436 CrossRef CAS.
- D. M. Watkins, Y. SayedSweet, J. W. Klimash, N. J. Turro and D. A. Tomalia, Langmuir, 1997, 13, 3136–3141 CrossRef CAS.
- H. He, Y. Li, X.-R. Jia, J. Du, X. Ying, W.-L. Lu, J.-N. Lou and Y. Wei, Biomaterials, 2011, 32, 478–487 CrossRef CAS PubMed.
- C. Kojima, K. Kono, K. Maruyama and T. Takagishi, Bioconjugate Chem., 2000, 11, 910–917 CrossRef CAS PubMed.
- P. K. Maiti, T. Cagin, S. T. Lin and W. A. Goddard, Macromolecules, 2005, 38, 979–991 CrossRef CAS.
- C. C. Lee, A. T. Cramer, F. C. Szoka Jr and J. M. J. Fréchet, Bioconjugate Chem., 2006, 17, 1364–1368 CrossRef CAS PubMed.
- W. Wang, D. Cheng, F. Gong, X. Miao and X. Shuai, Adv. Mater., 2012, 24, 115–120 CrossRef CAS PubMed.
- X. Xu, X. Chen, Z. Wang and X. Jing, Eur. J. Pharm. Biopharm., 2009, 72, 18–25 CrossRef CAS PubMed.
- M. Bigioni, C. Salvatore, A. Bullo, D. Bellarosa, E. Iafrate, F. Animati, G. Capranico, C. Goso, C. A. Maggi, G. Pratesi, F. Zunino and S. Manzini, Biochem. Pharmacol., 2001, 62, 63–70 CrossRef CAS.
- E. Briasoulis, V. Karavasilis, E. Tzamakou, D. Rammou, K. Soulti, C. Piperidou and N. Pavlidis, Cancer Chemother. Pharmacol., 2004, 53, 452–457 CrossRef CAS PubMed.
- C. Hua, S. M. Peng and C. M. Dong, Macromolecules, 2008, 41, 6686–6695 CrossRef CAS.
- Y. Yang, C. Hua and C. M. Dong, Biomacromolecules, 2009, 10, 2310–2318 CrossRef CAS PubMed.
- J. A. MacKay, M. N. Chen, J. R. McDaniel, W. G. Liu, A. J. Simnick and A. Chilkoti, Nat. Mater., 2009, 8, 993–999 CrossRef PubMed.
- Y. Li, H. He, X. Jia, W.-L. Lu, J. Lou and Y. Wei, Biomaterials, 2012, 33, 3899–3908 CrossRef CAS PubMed.
Footnote |
† Electronic supplementary information (ESI) available. See DOI: 10.1039/c3ra43803a |
|
This journal is © The Royal Society of Chemistry 2014 |