DOI:
10.1039/C3RA43364A
(Paper)
RSC Adv., 2014,
4, 3502-3511
Degradation of aniline with zero-valent iron as an activator of persulfate in aqueous solution†
Received
2nd July 2013
, Accepted 4th November 2013
First published on 14th November 2013
Abstract
Zero valent iron (ZVI) can activate persulfate to generate sulfate free radicals which are a strong oxidant to degrade organic pollutants. The oxidative degradation of aniline in aqueous solution by persulfate activated with zero valent iron was studied under laboratory conditions. Batch experiments were conducted to investigate the effects of different parameters such as pH, ZVI concentration, aniline concentration, persulfate concentration and reaction temperature on aniline degradation. The results showed that aniline degradation increased with increasing temperature. The optimum dosage of ZVI was 0.4 g L−1 and 85% aniline degradation was observed. Maximum aniline degradation was observed at pH 4.0, whereas at pH above or below 4.0, aniline degradation efficiency was decreased. In the persulfate-ZVI system, the apparent energy of activation for aniline degradation was 14.85 kJ mol−1. The existence of persulfate radicals and hydroxyl radicals produced during the degradation of aniline were identified with scavenger ethanol and tert-butyl alcohol. The reaction intermediates nitrobenzene, nitroso-benzene and p-benzoquinone were detected by gas chromatography-mass spectrometry and based on these intermediates obtained a probable pathway for aniline degradation has been proposed.
1. Introduction
Aromatic amines such as aniline are some of the most toxic pollutants present in the effluent of many industries. Aniline has been widely used as a raw material in many industries such as plastic, paint, dye manufacturing, pesticides, rubber chemicals, antioxidants and pharmaceuticals.1–3 Aniline has been classified as a persistent pollutant by China and USA because of its potent toxicity to humans and wildlife.4,5 It has been recognized as a high priority contaminant. Therefore, efficient treatment methods for aniline have been explored which are: ozonation, electro-Fenton and fluidized-bed Fenton processes, chemical oxidation and electro-catalytic oxidation.6–10
Advanced oxidation processes (AOPs) are being considered as an alternative to various treatment technologies for the treatment of contaminants. AOPs are based on the utilization of highly reactive oxidizing radicals to oxidize organic pollutants. Hydrogen peroxide, ozone, potassium permanganate were used as common oxidants in AOPs.11–13 These oxidants have some limitations, like H2O2 and O3 have short life spans due to their high reactivity; O3 has low water solubility and KMnO4 only reacts with unsaturated compounds.11–13
Recently, persulfate have received much attention as common oxidant for the treatment of organic contaminants.14–18 Persulfate is strong and non-selective oxidant with high redox potential of 2.01 V. Persulfate ions are able to oxidize many organic substances because persulfate anion is the most powerful oxidant of the peroxygen family and one of the strongest oxidants used in remediation. In aqueous solution the sulfate radical is a strong oxidant with redox potential of 2.6 V similar to that of hydroxyl radical (2.8 V). The persulfate anion activated by initiator, including UV light, heat, bases, soil mineral or transition metals such as ferrous iron, produces sulfate free radical (SO4˙−), which is a stronger oxidant (E0 = 2.6 V) than persulfate anion.19–21
|
S2O82− + 2e− → 2SO42−
| (1) |
|
S2O82− + heat/UV → 2SO4˙−
| (2) |
|
S2O82− + 2Fe2+ → 2Fe3+ + 2SO4˙−
| (3) |
|
S2O82− + Fe2+ → Fe3+ + SO42− + SO4˙−
| (4) |
|
SO4˙− + Fe2+ → Fe3+ + SO42−
| (5) |
At neutral pH, sulfate radicals have higher redox potential, furthermore; sulfate radicals are more selective for oxidation at acidic pH when compared to hydroxyl radicals. Sulfate radicals are more effective for the degradation of recalcitrant organic compound due to higher stability in water.22 Sulfate radical oxidation has numerous advantages over other oxidizing systems such as high solubility, relatively stable at room temperature, wide operating pH range and strong oxidizing potential.23–25 As a result, sulfate radical-based advanced oxidation processes have been applied successfully for the remediation of different contaminants.26,27 Sulfate radicals are also produced by the activation of persulfate by visible light, microwave, natural organic compounds, quinones and chelated metal complex.28–32 Persulfate activation by ferrous iron has been used for waste water treatment including pollutants such as aniline and diuron.2,3 However, excess of ferrous iron in solution reacts with sulfate radical which results in the consumption of sulfate radical and iron hydroxide produced decreasing the oxidation efficiency.33–35 In the persulfate activation, it is important to maintain suitable quantities of Fe2+, preventing the rapid conversion of Fe2+ to Fe3+ and scavenging of sulfate radicals. Liang et al.36 used chelating agents such as EDTA and citric acid which maintain the concentration of Fe2+ in solution.
Zero valent iron was used as an alternative source of Fe2+ through the oxidation of ZVI to activate persulfate37 as shown in the following equations.
|
Fe0 + S2O82− → Fe2+ + 2SO42−
| (7) |
ZVI is readily oxidized to Fe2+ ion in the presence of oxidants. In the aqueous systems this phenomenon leads to the dissolution of solid which is the primary cause of metal corrosion.38 In persulfate-ZVI system, persulfate also directly reacts with ZVI to release Fe2+ (eqn (7)). The generation of ferrous iron and recycling of ferric iron at the ZVI surface can prevent the accumulation of excess ferrous iron and reduce the precipitation of iron hydroxides during the reaction (eqn (8)).
Oh et al.19 observed the activation of persulfate by ZVI without involving aqueous Fe2+. Heterogeneous activation of persulfate may be an alternative mechanism in which there is direct electron transfer from ZVI or surface bound Fe2+ to persulfate.39–41
|
Fe(s)0 + 2S2O82−(aq) → Fe2+ + 2SO4˙−(aq) + 2SO42−(aq)
| (9) |
|
Fe(surf)2+ + S2O82−(aq) → Fe(surf)3+ + 2SO4˙−(aq) + SO42−(aq)
| (10) |
In Fenton's oxidation process, ZVI was used as iron source. As a result, hydroxyl radicals were produced which degrade environmental pollutants. The degradation of contaminants by sulfate radical in persulfate-ZVI system is promising. Oh et al.19 investigated that the complete oxidation of polyvinyl alcohol was obtained by ZVI-activated persulfate in 2 h due to production of sulfate radicals. Furthermore, 91% removal of 2,4-dinitrotoluene in 300 min was observed which shows fast and distinct degradation of 2,4-dinitrotoluene in persulfate-ZVI system.39 Zhao et al. also reported 88% degradation of 4-chlorophenol in persulfate-ZVI system.34 In the persulfate-ZVI system, 98.5% decolorization of anthraquinone dye Reactive Blue 19 was observed in 90 min due to generation of sulfate radicals.42 These results clearly show that, in persulfate-ZVI system, mostly aniline was degraded through oxidation of persulfate rather than reduction of ZVI. The results also indicated that ZVI can activate persulfate to start organic oxidation, consistent with our previous result with PCA.17 Furthermore, the degradation of contaminants by sulfate radicals in persulfate-ZVI system also requires further active research work.43,44
The objectives of these studies were to determine the persulfate activation efficiency with ZVI for aniline degradation and to observe the effects of different reactions parameters on aniline degradation by persulfate and ZVI.
2. Materials and methods
2.1 Chemicals
All chemicals used in this study were reagent grade and ultrapure water produced by a Millipore milli-Q system. Sodium persulfate (>99.0%), sodium thiosulfate pentahydrate (>99.0%), disodium hydrogen phosphate dodecahydrate (>99.0%), sodium phosphate monobasic dehydrate (>99.0%), concentrated sulfuric acid (>98.0%), sodium hydroxide (>96.0%), and aniline (>98.0%) were purchased from Tianjin Kermel Chemical Reagent Co. Ltd (Tianjin China). Methanol (>99.9%) were obtained from Sigma-Aldrich USA. The ZVI (>99.0% pure, particle size 0.21 mm and surface area 0.14 m2 g−1) used in this study was purchased from Sigma-Aldrich USA.17
2.2 Experimental procedures
Stock solutions of aniline (10 mM) and persulfate (50 mM) were prepared in deionized water prior to each batch experiment. The buffer solution used was prepared by mixing 1.85 g L−1 of dibasic sodium phosphate (Na2HPO4) and 1.52 g L−1 of monobasic sodium phosphate (NaH2PO4). The initial pH of the solution was 7.0. The pH values of all solutions were adjusted with 1 M sodium hydroxide (NaOH) or sulfuric acid (H2SO4). All of the reactions were carried out in 250 mL Erlenmeyer flasks operated as completely mixed batch reactor systems. Reaction mixtures were obtained by mixing an appropriate amount of aniline (0.5 mL, 0.05 mM) from stock solution and phosphate-buffer solution (94.5 mL) on a rotary shaker at 125 rpm and 25 °C. After 10 min, 0.5 mL solution was collected from the reactor to determine the initial aniline concentration and then predetermined amount of ZVI particles were added followed by the addition of sodium persulfate (5 mL, 2.5 mM). At regular time intervals, the sample aliquots were taken from flasks and filtered through 0.45 μm membrane. After filtration all samples were quenched immediately with sodium thiosulfate, to stop the chemical oxidation reaction.
Several sets of the experiments were conducted to determine the effects of various parameters on aniline degradation. To determine the effect of pH on the aniline degradation, five pH regimes of aqueous solutions at 2.0, 4.0, 7.0, 9.0, and 11.0 were studied, respectively. The effect of ZVI dosages on the aniline degradation was studied at 0.1, 0.2, 0.3, 0.4 and 0.5 g L−1 ZVI, respectively. To investigate the effect of temperature on the oxidation of aniline in the persulfate-ZVI system, the temperature was maintained at 20, 40, 60 and 80 °C. The effects of aniline and persulfate concentration on the degradation of aniline were also studied. For all experiments, duplicate reactor systems were run simultaneously.
2.3 Analytical methods
The concentration of aniline in the aqueous phase was measured by HPLC (Shimadzu LC-20) equipped with UV detector at 244 nm. The HPLC column used was a reversed-phase C18 column (250 mm × 4.6 mm) and the temperature of the column was maintained at 40 °C. The mobile phase used was methanol–water (70
:
30, v/v) with a flow-rate at 0.7 mL min−1. The concentration of Fe2+ was determined colorimetrically using a UV-vis spectrophotometer at 510 nm after adding 1,10-phenanthroline to form a colored complex of Fe2+–phenanthroline.45 Persulfate ion was determined by iodometric titration with sodium thiosulfate.46 The intermediates were identified on a VARIAN 4000 gas chromatography tandem mass spectrometer (GC-MS) with a weak polarity chromatographic column (0.15 μm × 0.25 mm × 60 m). The temperature program used was: 0 min at 40 °C, raised to 100 °C at 5 °C min−1, then increased to 300 °C at 15 °C min−1. The injector temperature was set at 250 °C. The surface morphology and chemical composition of ZVI was analyzed using EVO LS10 (Car Zeiss) scanning electron microscope (SEM) being operated at an acceleration voltage of 10 kV and equipped with an Oxford Energy 400 X-ray energy dispersive spectrometer (EDS, INCA Energy). X-ray powder diffraction (XRD) pattern was obtained on a diffractometer with Cu KR radiation (D8 ADVANCE, Bruker).
3. Results and discussion
3.1 Comparison experiments
Batch experiments were conducted to investigate the degradation of aniline by persulfate, ZVI and the combination of persulfate and ZVI, respectively. The results showed that lower aniline degradation was observed by persulfate or ZVI alone when compared to persulfate-ZVI system. As shown Fig. 1, only 18.22% removal of aniline was observed in the ZVI system after 5 h of reaction. The degradation of aniline occurred due to ZVI surface adsorption and reduction. In the persulfate alone degradation experiment, 38.50% aniline removal was observed. In addition, oxidation of persulfate was also very slow. While in the persulfate-ZVI system, 72% aniline degradation was achieved due to the generation of sulfate radicals. The aniline decomposition efficiency in persulfate-ZVI system was much higher when compared to ZVI and persulfate only.
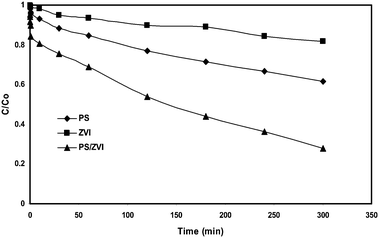 |
| Fig. 1 Effect of ZVI, persulfate (PS) and persulfate-ZVI on the degradation of aniline. [Aniline]0 = 0.05 mM; temp. = 25 °C; [PS]0 = 2.5 mM; [ZVI] = 0.3 g L−1; pH = 7.0. | |
3.2 Effects of ZVI dosage on aniline degradation
The effect of ZVI concentration on aniline degradation efficiencies of persulfate-ZVI system was evaluated by conducting experiments using different ZVI concentrations (0.1–0.5 g L−1) at fixed oxidant concentration (2.5 mM). The removal of aniline was significant as shown in Fig. 2. The aniline degradation efficiency increased with increasing ZVI doses. Higher amount of ZVI provides more sites for sulfate radical generation thereby increasing the rate of reaction. When the ZVI concentration was varied from 0.1 to 0.4 g L−1, the aniline degradation efficiency was 34%, 48%, 72% and 85%, respectively. The higher aniline degradation at higher ZVI concentration might be due to higher sulfate radical production. However, the degradation of aniline decreased with the increase in ZVI concentration from 0.4 to 0.5 g L−1. The aniline degradation efficiency in the persulfate-ZVI system followed this order 0.4 g L−1 > 0.5 g L−1 > 0.3 g L−1 > 0.2 g L−1 > 0.1 g L−1. As mentioned earlier, the activation of persulfate might be through the electron transfer at the ZVI surface or by Fe2+ in aqueous solution. As a result, persulfate activation and degradation of aniline were increased. Another set of experiment was conducted to compare the degradation of aniline in persulfate-Fe2+ system. The degradation efficiency of aniline was lower in persulfate-Fe2+ system (data shown in ESI†). The reaction of aniline degradation was rapidly terminated in persulfate-Fe2+ system because all the persulfate activated with excess Fe2+ quenched the sulfate radicals produced. Based on the results and reaction rate constants, it can be concluded that higher Fe2+ concentration can also act as a sulfate radical scavenger as mentioned by eqn (5) and caused the decrease in sulfate radical concentrations.41 Fe2+ was rapidly consumed and its concentration decreased sharply because of it short lifespan. The previous studies also indicated that destruction of sulfate radical might occurred in the presence of excess Fe2+ due to the rapid conversion of Fe2+ to Fe3+, which reduced the ultimate oxidizing capability.47–49 In persulfate-ZVI system, a continuous supply of Fe2+ could be provided to the persulfate system during the oxidation of ZVI by persulfate. The activation of persulfate to generate sulfate radicals is more effective when Fe2+ is supplied continuously when compared to its one-time dose. The results showed that the constant activity of ZVI in treatment or remediation processes can control oxidation process in a better way.
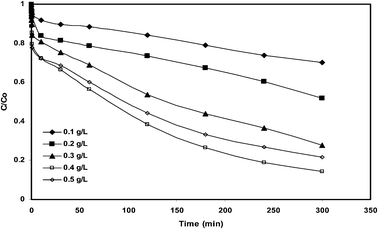 |
| Fig. 2 Effect of ZVI dosages on aniline degradation in persulfate-ZVI system. [Aniline]0 = 0.05 mM; temp. = 25 °C; [PS]0 = 2.5 mM; pH = 7.0. | |
3.3 Effect of persulfate and aniline concentration on aniline degradation
Persulfate ions are able to oxidize many organic substances because persulfate anion is the most powerful oxidant of the peroxygen family and one of the strongest oxidants used in remediation. In aqueous solution the sulfate radical is a strong oxidant with redox potential of 2.6 V similar to that of hydroxyl radical (2.8 V). In this study, the effect of persulfate concentrations (1–5 mM) on the degradation of aniline was studied at fixed concentration of ZVI (0.3 g L−1) (Fig. 3a). The higher persulfate concentration resulted in higher production of sulfate free radicals as well as higher degradation of contaminant. The results also showed that aniline degradation increased with increasing amount of persulfate (1–2.5 mM). Furthermore, 72% aniline degradation was achieved within 5 h of reaction with 2.5 mM persulfate concentration. However, further increase in persulfate concentration above certain limits (critical concentration), decreased the degradation rate of aniline because persulfate acts as a sulfate radical scavenger instead of a free-radical generator.42 |
SO4˙− + S2O82− → SO42− + S2O8˙−
| (11) |
|
SO4˙− + SO4˙− → S2O82−
| (12) |
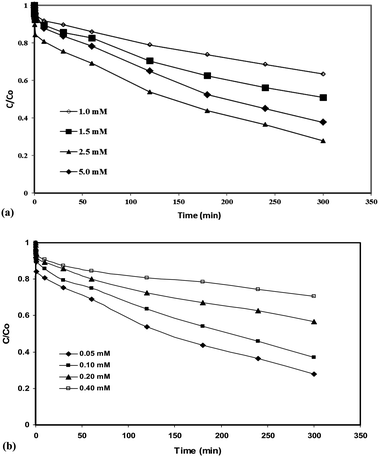 |
| Fig. 3 (a) Effect of persulfate concentration on the degradation of aniline; (b) effect of aniline concentration on the degradation of aniline. Temp. = 25 °C; [ZVI] = 0.3 g L−1; pH = 7.0. | |
The equation (11) showed that the addition of excess persulfate in persulfate-ZVI system, persulfate can compete with aniline for sulfate free radicals which can decrease the sulfate free radicals in the solution and then decreasing the aniline removal efficiency. The optimum persulfate concentration for aniline degradation was 2.5 mM (Fig. 3a).
The degradation efficiency also depends on the initial concentration of the pollutant. The effect of initial concentration of aniline on the rate of degradation in persulfate-ZVI system was investigated in the range of 0.05–0.40 mmol L−1 while maintaining the other parameters constant. It was observed that initial concentration of aniline had a significant effect on the degradation of aniline. It was found that increase in aniline concentration decreased the rate constant of aniline degradation. The rate constant of aniline degradation in persulfate-ZVI system at different aniline concentration 0.05, 0.10, 0.20, 0.40 mM were 0.0039, 0.003, 0.018 and 0.011 min−1 respectively. Increase in the concentration of aniline from 0.05–0.40 mmol L−1 decreased the degradation of aniline from 72% to 29% after 300 min (Fig. 3b). The lower degradation of aniline was due to excess concentration of aniline that might cover the iron surface and active sites for persulfate, retarding the degradation reaction.
3.4 Effect of pH on aniline degradation
The solution pH is an important parameter affecting the degradation of organic pollutants. Therefore the effect of pH on the aniline degradation efficiency was studied. The experiment was carried out by changing in the initial pH of persulfate-ZVI system from 2–11. The results showed that the degradation of aniline was significantly influenced by solution pH. The degradation of aniline decreased with the increase in the initial pH value (Fig. 4). As shown in Fig. 4, rapid degradation of aniline was observed at pH 4.0. At pH 4.0, aniline was completely removed after 10 min. These results are similar to our recent study in which complete p-chloroaniline (PCA) degradation was observed at pH 4.0.17 The degradation of aniline observed at pH 2.0, was 71% after 1 h and 76% after 5 h. The rate of aniline degradation at pH 7.0, 9.0 and 11 were 72%, 56.49% and 43.97%, respectively. The results obtained in this study were also in agreement with the previous findings which showed the rate of PCBs, propachlor, diphenylamine and azo dye orange G degradation by persulfate decreased with the increase in the pH value.50–53 In case of pH 2.0, however, degradation of aniline decreased when compared to pH 4.0, most likely because of the scavenging reactions of SO4˙−. Under strong acidic conditions (i.e., pH 2.0) the generation of SO4˙− increased due to acid-catalyzation via eqn (13) and (14), and then higher SO4˙− radical concentrations, which favored scavenging of SO4˙− by itself, or SO4˙−/S2O82− reaction in accordance with eqn (11) and (12).22 |
S2O82− + H+ → HS2O−8
| (13) |
|
HS2O−8 → SO4˙− + SO42− + H+
| (14) |
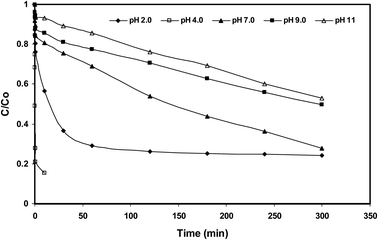 |
| Fig. 4 Effect of pH on the degradation of aniline in persulfate-ZVI system. [Aniline]0 = 0.05 mM; [PS]0 = 2.5 mM; temp. = 25 °C; [ZVI] = 0.3 g L−1. | |
Nevertheless, the aniline degradation efficiency at pH 2.0 was as high as 76.0% comparable to that at near neutral and basic pH. An optimum pH, 4.0 is appropriate and either decreasing or increasing the pH would result in the decrease of the degradation process. The precipitation of Fe3+ ions occurred whenever the pH was higher than 4.0. The oxyhydroxides of Fe3+ had low sulfate radical production efficiency by activating persulfate. The following equations indicate the formation of Fe3+ oxyhydroxides:
|
Fe3+ + H2O → FeOH2+ + H+ (k = 2.3 × 107 s−1)
| (15) |
|
Fe3+ + 2H2O → Fe(OH)2+ + 2H+ (k = 4.7 × 103 s−1)
| (16) |
|
2Fe3+ + 2H2O → Fe2(OH)24+ + 2H+ (k = 1.1 × 107 s−1)
| (17) |
The degradation of aniline at high pH values may be due to base activation of persulfate for the generation of sulfate free radicals in persulfate-ZVI system. The base activation mechanism of persulfate was shown by the following equation.20,51
|
2S2O82− + 2H2O → SO4˙− + 3SO42− + O2−˙ + 4H+
| (18) |
Moreover, under strong basic conditions sulfate radicals can react with hydroxyl anion to generate ˙OH and the reactivity of ˙OH is inhibited due to the presence of various anions (SO42−).47,51 The degradation efficacy of aniline decreased due to recombination of SO4˙− and ˙OH as shown in eqn (19).
|
SO4˙− + ˙OH → HSO−4 + 0.5O2
| (19) |
3.5 Effect of temperature on aniline degradation
In order to elucidate the effect of temperature on the degradation of aniline in persulfate-ZVI system a series of experiment were conducted at different temperature from 20 to 80 °C. As the temperature of the system increased, the degradation of aniline was faster. The results show (Fig. 5a) that aniline degradation efficiency increases with increase in temperature of persulfate-ZVI system. Complete degradation of aniline was observed for reaction carried out at 80 °C and 60 °C over 1 h and 2 h, respectively. However, the aniline degradation efficiencies were about 69 and 84% at 20, 40 °C, respectively. The results revealed that more generation of sulfate radicals occurred at higher temperature. This is because higher temperature increased the reaction rate between persulfate and ferrous iron. The rate constant of aniline degradation in persulfate-ZVI system at 20, 40, 60, 80 °C were 0.0037, 0.006, 0.0147, and 0.0319 min−1 respectively. Oh et al.19 observed that temperature is an important parameter to promote the oxidation of polyvinyl alcohol in persulfate-ZVI system. A set of experiments without ZVI were conducted to investigate the effect of temperature on aniline degradation because persulfate can also be activated by heating to generate sulfate radicals.8,14,19 Aniline degradation increased with increasing temperature from 20 to 80 °C without ZVI (Fig. 5b), but aniline degradation efficiency is less when compared to persulfate-ZVI system. The activation energy of the reaction was obtained by using Arrhenius equation.
ln k = ln A − Ea/RT |
where A is the frequency factor, R is the universal gas constant, Ea is the activation energy and T is the temperature in Kelvin. As shown inset Fig. 5, a good linear relationship was observed between ln
k vs. 1/T. The activation energy for the degradation of aniline in persulfate-ZVI system was 14.85 kJ mol−1. This lower value of activation energy indicates that aniline degradation requires low energy in persulfate-ZVI system when compared to thermal reaction energy of 60–250 kJ mol−1.53 The activation energy of catalytic degradation of aniline by immobilized iron oxide catalysts was reported to be 13.65–15.65 kJ mol−1.54 Shahrezaei et al.55 observed the activation energy of 20.337 kJ mol−1 for the degradation of aniline by photocatalytic using TiO2 nanoparticles. As compared to above mentioned activation energy, the relative lower value of aniline degradation in persulfate-ZVI system implies that such reaction can be easily attained.
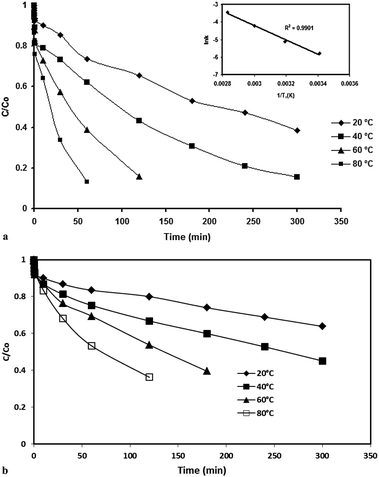 |
| Fig. 5 (a) Effect of temperature on the degradation of aniline in persulfate-ZVI system. [Aniline]0 = 0.05 mM; [PS]0 = 2.5 mM; [ZVI] = 0.3 g L−1; pH = 7.0. (b) Effect of temperature on the degradation of aniline with persulfate. [Aniline]0 = 0.05 mM; [PS]0 = 2.5 mM; [ZVI] = 0.3 g L−1; pH = 7.0. | |
3.6 Identification of predominant radicals in persulfate-ZVI system
To identify the dominating radical species in persulfate-ZVI system, alcohols were used to quench the hydroxyl and sulfate radicals. Therefore, ethanol (alcohol with alpha hydrogen) was used as hydroxyl and sulfate radical scavenger and tert-butyl alcohol (TBA) with no alpha hydrogen were effective quenching agents for hydroxyl radicals. In this study, alcohol (EtOH, TBA) concentration used was 1 M. The results clearly showed that aniline degradation was significantly decreased by the addition of alcohols. As shown in Fig. 6, the degradation of aniline in persulfate-ZVI system was 85% without any radical scavenger. However, in the presence of ethanol (EtOH), only 16% aniline degradation was observed. While in the presence of TBA, the degradation of aniline was 44%. The results revealed that the reaction was completely quenched by the addition of EtOH as ˙OH radical and SO4−˙ radical scavengers, while with the addition of TBA, the degradation rate of aniline was moderately influenced. According to previous literature, the second order rate constants of the EtOH and TBA with hydroxyl radicals and sulfate radicals are following.48,56–58
t-BuOH + HO˙ ∼ 3.8–7.6 × 108 M−1 s−1 |
t-BuOH + SO4−˙ ∼ 4.0–9.1 × 105 M−1 s−1 |
EtOH + HO˙ ∼ 1.2–2.8 × 109 M−1 s−1 |
EtOH + SO4−˙ ∼ 1.6–7.7 × 107 M−1 s−1 |
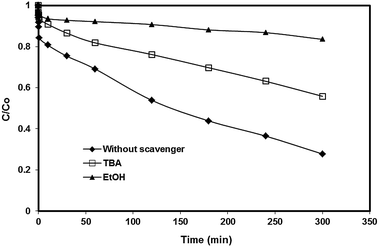 |
| Fig. 6 Effect of EtOH and TBA as radical scavengers on the degradation of aniline in persulfate-ZVI system. [Aniline]0 = 0.05 mM; [PS]0 = 2.5 mM; temp. = 25 °C; [ZVI] = 0.3 g L−1; pH = 7.0, EtOH = 1 M, TBA = 1 M. | |
On the basis of the above mentioned rate of constants for TBA and EtOH, it can be inferred that in TBA system the degradation of aniline was due to the formation of sulfate radicals as TBA quenched hydroxyl radicals only. While in the presence of EtOH, the efficiency of aniline degradation was much lower (16%) because both radicals were scavenged. Sulfate radicals are more selective for electron transfer reaction while hydroxyl radicals rapidly undergo by hydrogen addition or abstraction. Therefore, we observed that sulfate radicals are dominant active species for the degradation of aniline.
3.7 Removal of TOC in persulfate-ZVI system
The TOC removal efficiency indicates the effective degradation of organic pollutants. The experiment was conducted to investigate the removal of TOC. Experiment was carried out at aniline initial concentration 0.05 mM L−1, persulfate 2.5 mM L−1, dosage of ZVI 0.3 g L−1 and pH 7.0. Aniline mineralization by persulfate-ZVI process was quantified by measuring TOC contents. As shown in Fig. 7, aniline mineralization was significant, where 25% TOC removal efficiency was achieved within 1 h. TOC removal efficiency increased with increasing time, 61.5% TOC was removed after 5 h reaction time. The results showed that aniline was not directly oxidized to CO2 and H2O in one step. Therefore, intermediates were produced and then open-ringed and organic acids were produced. Sulfate radicals were considered as major oxidant for the degradation of aniline and its intermediates. At pH 7.0, aniline degradation was higher when compared to TOC removal efficiency. The degradation of aniline was 72% and TOC removal was 61.5% in ZVI-activated persulfate system. TOC removal efficiency of aniline was more when compared to p-chloroaniline.17 In other study only 24% TOC removal was observed during degradation of aniline.59 The results showed that persulfate-ZVI combined system was not only effective for the degradation of aniline, but also for the mineralization of aniline.
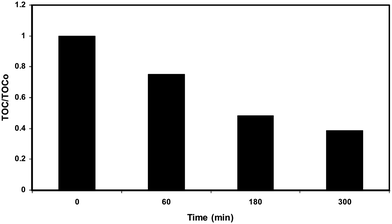 |
| Fig. 7 Removal of TOC in persulfate-ZVI system. [Aniline]0 = 0.05 mM; [PS]0 = 2.5 mM; [ZVI] = 0.3 g L−1; pH = 7.0. | |
3.8 Mechanism of aniline degradation
To explore the formation of intermediates during the degradation of aniline in persulfate-ZVI was identified by gas chromatography-mass spectrometry (GC-MS). The decomposition of persulfate in aqueous solution produced hydroxyl and sulfate radicals which can oxidize many organic pollutants into carbon dioxide.13 The results revealed that during the degradation process of aniline, the color of aniline solution varied from colorless to yellow and brown respectively and then ultimately became colorless. During the process of degradation, some complex intermediate products were developed causing a change in the color of aniline solution. The possible intermediate products were p-benzoquinone, nitroso-benzene and nitrobenzene. Based on the intermediates detected in this study and previous literature,6,10 a pathway might be proposed for the degradation of aniline in persulfate-ZVI system (Fig. 8). The degradation of aniline process initiated by the attack of sulfate and hydroxyl radicals and yield either benzoquinonimine or nitrobenzene. The oxidation of benzoquinonimine produced p-benzoquinone. Further degradation of nitrobenzene, as well as p-benzoquinone produced maleic acid, and further maleic acid transformed into oxalic acid. The maleic acid directly or via oxalic acid mineralized to CO2 and H2O. The generation of hydroxyl and persulfate radicals is essentially important in organic oxidation because these are powerful oxidizing agents. Sulfate and hydroxyl radicals are electrophilic radicals whom attack on benzene ring result into mineralization of aniline and its intermediates to CO2 and H2O.
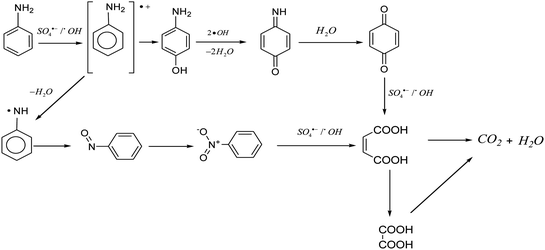 |
| Fig. 8 Proposed reaction pathway for the degradation of aniline degradation in persulfate-ZVI. | |
4. Observation of ZVI surface
The changes in the iron surface were observed by conducting SEM with corresponding EDS and XRD. The result showed that different surface texture was seen due to corrosion of iron by persulfate oxidation on the surface of ZVI. An amorphous plate-like porous structure (Fig. 9b) was formed in ZVI/PS system (in the absence of aniline) while coarse and grooving aggregate formation was seen in ZVI/PS/aniline system (Fig. 9c, in the presence of aniline).37 As shown in Fig. 10, SEM-EDX analysis showed the changes occurred in the elemental composition of ZVI. The ZVI surface exhibited the presence of oxygen after oxidation with persulfate. Atomic weight-based quantitative SEM-EDX peak area analysis showed original ZVI having Fe
:
C = 99.00
:
1.00% (Fig. 10a). The elemental composition changed to Fe
:
O
:
C = 58.77
:
40.23
:
1.00 after reaction with persulfate (Fig. 10b). This % atomic ratio further changed to Fe
:
O
:
C = 19.31
:
55.24
:
25.45 in ZVI/PS/aniline system (Fig. 10c). The XRD diffractogram of original ZVI is shown in Fig. 11a. The Fig. 11a indicates that iron is mainly in its Fe0 state, characterized by the basic reflection appearing at a 2-theta value of 44.9°. Signal of the iron oxides (magnetite, Fe3O4) were observed at a 2-theta value of 35.4 in the XRD patterns of persulfate-ZVI system. It indicated that the reflections of magnetite in the spectrum were very weak, but magnetite reflection was not observed in the XRD spectrum in ZVI/PS/aniline system (Fig. 11c).
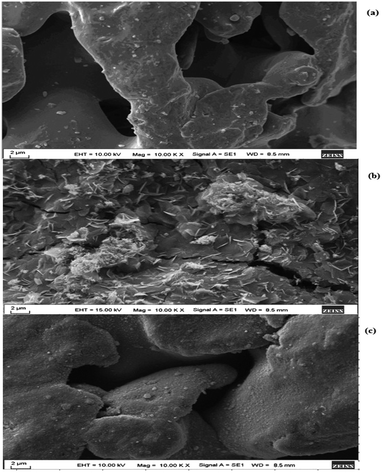 |
| Fig. 9 SEM images of the ZVI surface from the systems of (a) original ZVI, (b) ZVI/PS, and (c) aniline/ZVI/PS. | |
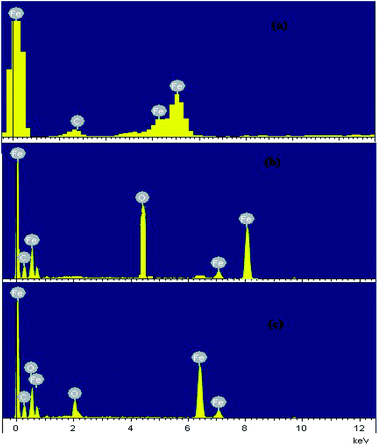 |
| Fig. 10 Results of EDS analysis for residual elements on the ZVI surface (a) original ZVI, (b) ZVI/PS, and (c) aniline/ZVI/PS. | |
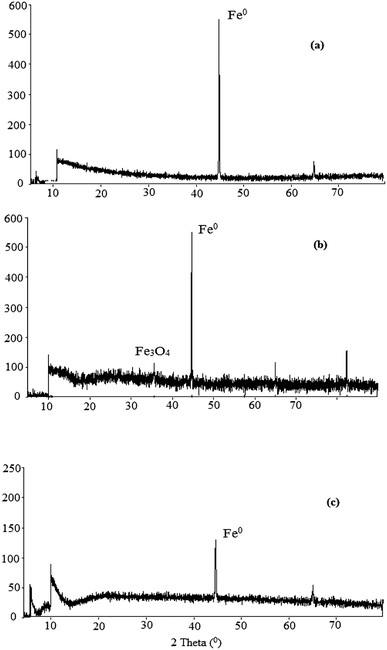 |
| Fig. 11 XRD spectrum of ZVI particles for (a) original ZVI, (b) ZVI/PS, and (c) aniline/ZVI/PS. | |
5. Conclusions
The oxidation system consisting of persulfate-ZVI has been demonstrated to be an effective oxidant reagent to degrade aniline in aqueous solution. In the persulfate-ZVI system the decomposition of persulfate occurred more rapidly due to the release of Fe2+ from ZVI. Increased ZVI dosages resulted in enhanced aniline degradation due to increased activation of persulfate by ZVI. Aniline was successfully degraded in the persulfate-ZVI system within 1 h at a temperature of 80 °C. The oxidation reaction showed excellent Arrhenius behavior with an activation energy of about 14.84 kJ mol−1. The results showed that high temperature and acidic pH values e.g. 4.0 were more favorable than neutral and basic pH for complete degradation of aniline. The optimum pH for degradation of aniline was 4.0 at which 100% degradation efficiency was achieved after 10 min. Radical scavengers such as EtOH and TBA demonstrated the responsibility of sulfate radicals as well as hydroxyl radicals in the degradation of aniline. Nitrobenzene, p-benzoquinone and nitroso-benzene are indentified as intermediates during the degradation of aniline in the persulfate-ZVI system. This study indicates that the activation of persulfate by ZVI is an effective tool for remediation of water contaminated with aniline.
Acknowledgements
This study was financially supported by the Foundation of Science and Technology Planning Project of Guangdong Province (2010B050200007), the Fundamental Research Funds for the Central Universities (2011ZM0054) and the Research Fund Program of Guangdong Provincial Key Laboratory of Environmental Pollution Control and Remediation Technology (2011k0013) and Research Funds of Guangdong Provincial Key Laboratory of Atmospheric environment and Pollution Control.
References
- E. Brillas and J. Casado, Chemosphere, 2002, 47, 241 CrossRef CAS.
- L. Y. Zhu, B. Ma, L. Zhang and L. Zhang, Chemosphere, 2007, 69, 1579 CrossRef CAS PubMed.
- J. Anotai, N. Masomboon, C.-L. Chuang and M.-C. Lu, Water Sci. Technol., 2011, 63, 1434 CAS.
- M. F. Khan, X. Wu, B. S. Kaphalia, P. J. Boor and G. A. Ansari, Toxicology, 2003, 194, 95 CrossRef CAS PubMed.
- L. Wang, C. B. Zhang, F. Wu and N. S. Deng, J. Photochem. Photobiol., B, 2007, 87, 49 CrossRef CAS PubMed.
- R. Sauleda and E. Brillas, Appl. Catal., B, 2001, 29, 135 CrossRef CAS.
- J. Anotai, C. C. Su, Y. C. Tsai and M. C. Lu, J. Hazard. Mater., 2010, 183, 888 CrossRef CAS PubMed.
- Y. Zhang, W. Huang and E. F. Donna, Chin. Chem. Lett., 2010, 21, 911 CrossRef CAS PubMed.
- N. Fu, X. Y. Tu and Y. Pan, Environ. Chem., 2008, 27, 578 CAS.
- X. Xie, Y. Zhang, W. Huang and S. Huang, J. Environ. Sci., 2012, 24, 821 CrossRef CAS.
- R. J. Watts and A. L. Teel, J. Environ. Eng., 2005, 131, 612 CrossRef CAS.
- F. J. Rivas, J. Hazard. Mater., 2006, 138, 234 CrossRef CAS PubMed.
- R. H. Waldemer and P. G. Trantnyek, Environ. Sci. Technol., 2006, 40, 1055 CrossRef CAS.
- C. J. Liang, C. J. Bruell, M. C. Marley and K. L. Sperry, Chemosphere, 2004, 55, 1225 CrossRef CAS PubMed.
- G. P. Anipsitakis and D. D. Dionysiou, Appl. Catal., B, 2004, 54, 155 CrossRef CAS PubMed.
- A. Rastogi, S. R. Al-Abed and D. D. Dionysiou, Water Res., 2009, 43, 684 CrossRef CAS PubMed.
- H. Liang, Y. Zhang, S. Huang and I. Hussain, Chem. Eng. J., 2013, 218, 384 CrossRef CAS PubMed.
- I. Hussain, Y. Zhang, S. Huang and X. Du, Chem. Eng. J., 2012, 203, 269 CrossRef CAS PubMed.
- S.-Y. Oh, H. W. Kim, J.-M. Park, H.-S. Park and C. Yoon, J. Hazard. Mater., 2009, 168, 346 CrossRef CAS PubMed.
- O. S. Furman, A. L. Teel and R. J. Watts, Environ. Sci. Technol., 2010, 44, 6423 CrossRef CAS PubMed.
- J. Saien, Z. Ojaghloo, A. R. Soleymani and M. H. Rasoulifard, Chem. Eng. J., 2011, 167, 172 CrossRef CAS PubMed.
- A. Romero, A. Santosa, F. Vicentea and C. Gonzálezb, Chem. Eng. J., 2010, 162, 257 CrossRef CAS PubMed.
- K.-C. Huang, Z. Zhao, G. E. Hoag, A. Dahmani and P. A. Block, Chemosphere, 2005, 61, 551 CrossRef CAS PubMed.
- J. C. Yan, M. Lei, L. H. Zhu, M. N. Anjum, J. Zou and H. Q. Tang, J. Hazard. Mater., 2011, 186, 1398 CrossRef CAS PubMed.
- Y. R. Wang and W. Chu, Water Res., 2011, 45, 3883 CrossRef CAS PubMed.
- C. J. Liang, Z. S. Wang and C. J. Bruell, Chemosphere, 2007, 66, 106 CrossRef CAS PubMed.
- C. H. Yen, K. F. Chen, C. M. Kao, S. H. Liang and T. Y. Chen, J. Hazard. Mater., 2011, 186, 2097 CrossRef CAS PubMed.
- G. Subramanian, P. Parakh and H. Prakash, Photochem. Photobiol. Sci., 2013, 12, 456 CAS.
- N. B. Oncu and I. A. Balcioglu, Bioresour. Technol., 2013, 146, 126 CrossRef PubMed.
- M. Ahmad, A. L. Teel and R. J. Watts, Environ. Sci. Technol., 2013, 47, 5864 CrossRef CAS PubMed.
- G. Fang, J. Gao, D. D. Dionysiou, C. Liu and D. Zhou, Environ. Sci. Technol., 2013, 47, 4605 CrossRef CAS PubMed.
- G. Subramanian, P. Parakh and H. Prakash, J. Hazard. Mater., 2012, 213–214, 19 Search PubMed.
- C. Liang and Y.-Y. Guo, Environ. Sci. Technol., 2010, 44, 8203 CrossRef CAS PubMed.
- J. Zhao, Y. Zhang, X. Quan and S. Chen, Sep. Purif. Technol., 2010, 71, 302 CrossRef CAS PubMed.
- F. Vicente, A. Santos, A. Romero and S. Rodriguez, Chem. Eng. J., 2011, 70, 127 CrossRef PubMed.
- C. J. Liang, C. J. Bruell, M. C. Marley and K. L. Sperry, Chemosphere, 2004, 55, 1213 CrossRef CAS PubMed.
- C. Liang and M.-C. Lai, Environ. Eng. Sci., 2008, 25, 1071 CrossRef CAS.
- R. Venkatpathy, D. G. Bessingpas, S. Canonica and J. A. Perlinger, Appl. Catal., B, 2002, 37, 139 CrossRef.
- S.-Y. Oh, S.-G. Kang and P. C. Chiu, Sci. Total Environ., 2010, 408, 3464 CrossRef CAS PubMed.
- T. Kohn, K. J. T. Livi, A. L. Roberts and P. J. Vikesland, Environ. Sci. Technol., 2005, 39, 2867 CrossRef CAS.
- Y. H. Huang and T. C. Zhang, Water Res., 2006, 40, 3075 CrossRef CAS PubMed.
- C. Le, J.-H. Wu, P. Li, X. Wang, N.-W. Zhu, P.-X. Wu and B. Yang, Water Sci. Technol., 2011, 64, 754 CrossRef CAS.
- L. G. Devi, S. G. Kumar, K. M. Reddy and C. Munikrishnappa, J. Hazard. Mater., 2009, 164, 459 CrossRef PubMed.
- D. H. Bremmer, A. E. Burgess, D. Houllemare and K.-C. NamKung, Appl. Catal., B, 2006, 63, 15 CrossRef PubMed.
- APHA, AWWA, WEF, Washington, DC, 1998.
- I. M. Kolthoff and V. A. Stenger, Volumetric Analysis, Titration Methods: Acid Base, Precipitation, and Complex Reactions, second revised ed., Interscience Publishers Inc., New York, vol. 2, 1947 Search PubMed.
- I. M. Kolthoff and I. K. Miller, J. Am. Chem. Soc., 1951, 73, 3055 CrossRef CAS.
- G. P. Anipsitakis and D. D. Dionysiou, Environ. Sci. Technol., 2004, 38, 3705 CrossRef CAS.
- S. S. Gupta and Y. K. Gupta, Inorg. Chem., 1981, 20, 454 CrossRef CAS.
- A. Rastogi, S. R. Al-Abed and D. D. Dionysiou, Appl. Catal., B, 2009, 85, 171 CrossRef CAS PubMed.
- C. S. Liu, K. Shih, C. X. Sun and F. Wang, Sci. Total Environ., 2012, 416, 507 CrossRef CAS PubMed.
- S. X. Li, D. Wei, N. K. Mak, Z. W. Cai, X. R. Xu and H. B. Li, J. Hazard. Mater., 2009, 164, 26 CrossRef CAS PubMed.
- X.-R. Xu and X.-Y. Li, Sep. Purif. Technol., 2010, 72, 105 CrossRef CAS PubMed.
- Y.-H. Huang, C.-C. Su, Y.-P. Yang and M.-C. Lu, Environ.
Eng. Sci., 2011, 28, 891 CrossRef CAS.
- F. Shahrezaei, Y. Mansouri, A. A. L. Zinatizadeh and A. Akhbari, Int. J. Photoenergy, 2012 DOI:10.1155/2012/430638.
- A. Ghauch and A. Tuqan, Chem. Eng. J., 2012, 183, 162 CrossRef CAS PubMed.
- A. Ghauch, A. Tuqan and N. Kibbi, Chem. Eng. J., 2012, 197, 483 CrossRef CAS PubMed.
- Y. F. Huang and Y. H. Huang, J. Hazard. Mater., 2009, 162, 1211 CrossRef CAS PubMed.
- J. Anotai, M.-C. Lub and P. Chewpreecha, Water Res., 2006, 40, 1841 CrossRef CAS PubMed.
Footnote |
† Electronic supplementary information (ESI) available. See DOI: 10.1039/c3ra43364a |
|
This journal is © The Royal Society of Chemistry 2014 |