DOI:
10.1039/C3RA42682C
(Paper)
RSC Adv., 2014,
4, 11367-11374
Stable nano-sized copper and its oxide particles using cobalt tetraamino phthalocyanine as a stabilizer; application to electrochemical activity†
Received
31st May 2013
, Accepted 13th January 2014
First published on 14th January 2014
Abstract
Uniform and monodisperse copper nanoparticles have been synthesized using a cobalt tetraamino phthalocyanine macrocyclic complex as a stabilizer in a one-step process in inert atmosphere. The resultant nanoparticles are characterized by electronic absorption spectra, infrared spectroscopy, transmission electron microscopy and X-ray diffraction. The nanoparticles possess a relatively narrow size distribution. Copper nanoparticles have an average diameter of 2 to 3 nm with a spherical shape. When the copper particles are exposed to air, nanorod like structures of copper oxide nanoparticles are formed from tiny copper nanoparticles through a process involving slow oxidation of copper nanoclusters by the Ostwald ripening process and orientation attachment phenomena. In order to compare the electrochemical activity and efficiency of phthalocyanine capped copper and copper oxide nanoparticles, electrocatalytic reduction of oxygen was carried out. The copper nanoparticles showed better electrocatalytic activity than their oxide counterparts probably due to the blocking behavior of aggregated copper oxide nanoparticles.
Introduction
Metal nanoparticles have been shown to have applications in the areas of catalysis,1 optoelectronics and electron microscopy markers,2,3 DNA detection4 etc. due to their size and shape dependent optical, electrical and electronic properties. The chemical and electrochemical synthesis of nanoparticles is generally carried out by reduction of the corresponding metal salt solution, sometimes in the presence of a capping agent. Though much interest has been given on the synthesis, characterization and application of gold and silver nanoparticles due to their unique surface plasmon resonance and high stability,5 little work has been done towards oxidisable metal nanoparticles. Further, the literature on the preparation of oxidisable metal nanoparticles is very meager due to their extreme sensitivity to oxygen which is much more enhanced in nanoscale structures. There are several problems related to the stability and oxidation resistance of these nanoparticles that need to be solved. Only a few studies have been carried out in this area6–17 and there are no clear guidelines for improvement of their air stability.
The ligand or capping agent plays a major role in stabilization of the nanoparticles and can also prevent oxidation. In this regard, we are interested in the preparation of macrocycle stabilized copper nanoparticles and their application towards electrocatalytic reduction of small molecules. As for the use of phthalocyanines as capping agents for metal nanoparticles, there are only limited reports available in the literature.14–21 Thiol functionalized phthalocyanines have been used as capping agents to prepare uniformly distributed gold and titanium dioxide nanoparticles and further used as photosensitizers and in photovoltaic applications.18–21 Stabilization of copper nanoparticles using phthalocyanines has not been reported in the literature. The difficulty in preparing monodisperse, stable copper nanoparticles using conventional capping agents such as alkane thiols has led to the search for other capping agents.
Copper nanoparticles can be used in the field of catalysis, inkjets, sensors and field emission emitters and their oxides in superconductors and in lithium batteries.6–8 Attempts have been made to synthesize copper nanoparticles and stabilize them by capping with various surfactants such as alkanethiols,9 reverse micelles as microreactors, electrocatalytic techniques and arc-discharge processes, laser irradiation, thermal decomposition, flame spray.10–13 Macromolecules such as dendrimers and polymers have also been used, but to a very limited extent.6–17 The oxidation state, size and stability of the copper nanoparticles vary greatly in these syntheses. The structure of phthalocyanine is highly versatile and can be tuned based on chemical modifications on the ring as well as in the central core. Phthalocyanines with different substituents at the peripheral benzene ring could then act as stabilizing agents for metal nanoparticles.14–21 The functionalized metal nanoparticles may have improved catalytic and optical properties.14–22 Synthesis of phthalocyanine with a cobalt central metal ion is easy and well-known.22 Phthalocyanine complexes of cobalt are good conductors and are electrocatalytically highly active compared to other metal ions.14–16,22,23
In the present study, cobalt tetraamino phthalocyanine has been used to stabilize copper nanoparticles. The four amino groups present in the periphery of the benzene ring of the phthalocyanine macrocycle are expected to stabilize the nanoparticle in a controlled fashion, thereby preventing aggregation. Since phthalocyanine is known to be a good catalyst for oxygen reduction, it is expected that the reduction will be catalyzed to a larger extent when the cobalt tetraamino phthalocyanine stabilized nanoparticle is used, wherein the capping agent as well as the nanosized particle can take part.
Results and discussion
The structure of the macrocycle CoPTA used in the present studies is shown in Fig. 1A. It contains four amine functional groups at the periphery of the benzene rings. This compound is green in color, planar, highly stable and the core structure is similar to biologically active molecules such as chlorophyll and haemoglobin. It is soluble in DMSO and concentrated sulphuric acid without undergoing any degradation.
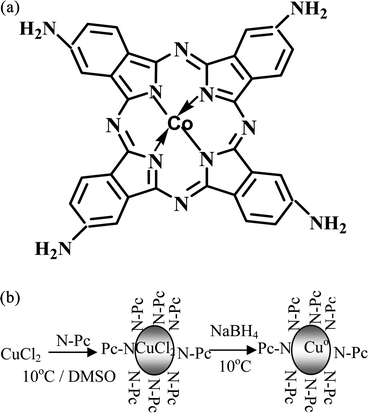 |
| Fig. 1 (A) Structure of cobalt tetraamino phthalocyanine (CoPTA). (B) Schematic showing the preparation of CoPTA capped copper nanoparticle where N-Pc = CoPTA. | |
The schematic for the preparation of CoPTA capped copper nanoparticles and the probable interactions are shown in Fig. 1B. The nitrogen atom of the amine groups on the periphery of the benzene rings of the phthalocyanine structure interact with copper nanoparticles, stabilizing them in a very efficient way. Covalent strong interactions between the amine and metal and steric effects due to macrocycle parts in the phthalocyanine were combined to efficiently stabilize the metallic nanoparticles.
Electronic absorption spectroscopy
The UV-visible absorption spectroscopy is very sensitive to the presence of copper nanoparticles due to the intense surface plasmon absorption peak exhibited by the particles.9–12,24 UV-visible absorption spectra were recorded for the nanoparticles prepared freshly using DMSO as a blank. The spectra were recorded in the region 300 to 900 nm at a scan rate of 240 nm min−1. Fig. 2A shows the UV-visible absorption spectra of freshly prepared copper nanoparticles in DMSO with and without the capping agent. The spectrum of pure CoPTA is also shown for comparison. The UV-visible spectra of pure CoPTA in DMSO showed absorbance peaks around 305 and 375 nm corresponding to the B band, a shoulder around 651 nm corresponding to the dimers and oligomers of the phthalocyanine and ∼738 nm for the Q band absorption. The stabilized nanoparticle solutions dampen the absorption of free phthalocyanine. A strong peak ∼576 nm is observed due to the excitation of surface plasmon vibrations in the copper nanoparticles. The plasmon absorption peaks observed for the copper nanoparticles coincide well with the reported values in the literature.24 The sharpness of the peak indicates formation of almost uniform and monodispersed nanoparticles. The spectra of the nanoparticle solutions remain unchanged with time in inert atmosphere for at least one week, indicating that the particle size distribution in the organic phase is stable. Copper nanoparticles prepared in a similar way (as mentioned above) without the capping agent showed a blue shift in the copper plasmon peak which resembles that of copper oxide,25 indicating that the uncapped copper nanoparticles are not stable even if they are prepared in inert atmosphere and undergo oxidation simultaneously. The broad band of these uncapped copper particles indicates that these are polydisperse, and aggregated as confirmed by TEM. The CoPTA stabilized copper nanoparticles are found to have a uniform size distribution when the temperature during the preparation process is maintained around 10 °C. Further, the size and shape of the particle also depends on the concentration ratio of the capping agent and metal salt. At low concentrations of the metal salt, the size can be controlled very well. The CoPTA protected metallic nanoparticles are stable and can be handled as powder without any aggregation. The particles can be re-dispersed in organic solvents such as dimethyl sulphoxide and dimethyl formamide without compromising with the particle size and distribution in inert atmosphere.
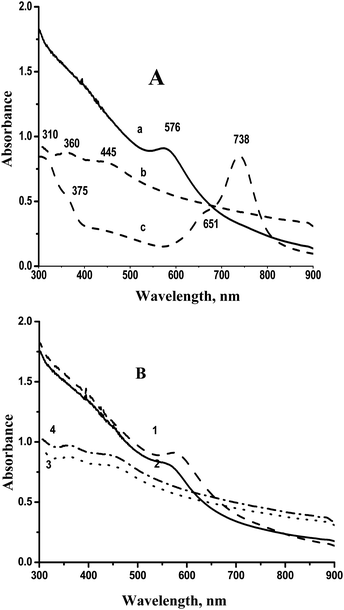 |
| Fig. 2 (A) UV-visible absorption spectra in DMSO of freshly prepared (a) CoPTA capped copper nanoparticles, (b) copper nanoparticles without capping agent and (c) 0.05 mM CoPTA. (B) Stability studies of CoPTA capped copper nanoparticles after exposing to air with time using UV-visible absorption spectra (1) freshly prepared, (2) 4 h, (3) 12 h and (4) 1 day. | |
Electronic absorption spectroscopy has also been used to study the stability of the copper nanoparticles in ambient conditions. Fig. 2B shows the UV-Vis absorption spectra of copper nanoparticles after exposing them to air at different time intervals. The intensity of the copper plasmon peak at ∼576 nm decreased slowly with time and there was a blue shift in the absorption peak. After one day the peak at 576 nm completely disappeared and a peak around 445 nm is observed. A change in the color of the colloidal solution to pale yellow from greenish was also noticed with the settling of particles. This may be due to the formation of copper oxide nanoparticles or aggregation of the particles. The effect of oxygen on the plasmon absorbance of copper nanoparticles has been observed before both in simulation and experiments.26 In the literature it has been reported that the oxidation of the copper nanoparticle to its oxide takes place within 30 min, whereas the copper nanoparticles prepared in this case are more stable and undergo slow oxidation, which may be due to the versatile nature of the phthalocyanine complex and the strong interaction between copper and nitrogen atoms of four amine groups. When phthalocyanine has a flat orientation23 around the copper nanoparticles, then all the nitrogen atoms from four amine groups interact with copper nanoparticles to stabilize them. The amine and cyano groups present in phthalocyanines form a supramolecular self assembly27,28 around the copper nanoparticles, which hinders the access of oxygen to copper. Further, phthalocyanine, which is conjugated in nature, interacts with oxygen like porphyrin and does not allow oxygen to interact with copper nanoparticles.29 Further theoretical studies are in progress to understand the nature of bonding between the copper and amine groups of phthalocyanine macrocycles.
FT-IR spectra
The infrared spectra for the pure CoPTA (Fig. 3a) and dry CoPTA capped copper nanoparticles (Fig. 3b) dispersed in KBr are shown in Fig. 3. The FT-IR spectrum was recorded in the region 400–1600 cm−1 for the copper nanoparticles stored in an inert atmosphere. The spectrum in the finger print region of 400–1600 cm−1 showed bands at 1120, 1082, 930, 880 and 760 cm−1 corresponding to the phthalocyanine skeletal vibrations of the stabilizer.15,16,22,23 Decrease in the intensity of absorption and shift in the characteristic peaks by 10–20 cm−1 was observed for CoPTA in the FT-IR spectrum of the nanoparticles, which may be due to the strong interaction between the macrocycle and copper nanoparticles in addition to the steric effects in stabilizing the nanoparticles. This indicates that the phthalocyanine capping is strong and is preserved in the solid state of the nanoparticles as well.
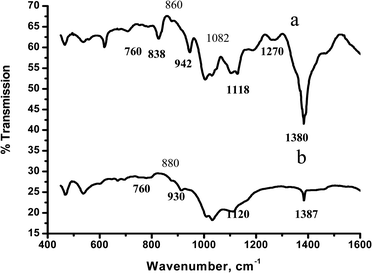 |
| Fig. 3 FT-IR spectra of (a) CoPTA and (b) CoPTA capped copper nanoparticles dispersed in KBr. | |
The BET surface area for freshly prepared CoPTA capped copper nanoparticles and control copper nanoparticles showed 72 and 54 m2 g−1 and after being exposed to air for 24 h were found to be 67 and 46 m2 g−1, respectively.
Transmission electron microscopy
The phthalocyanine stabilized copper nanoparticles were characterized using TEM. The colloidal nanoparticles dispersed in DMSO were spread on a Formvar/carbon-coated copper grid, dried and used for taking TEM images, diffraction pattern and small area diffraction. Colloidal particles dispersed on a nickel grid were used for EDAX (energy dispersive X-ray analysis) studies. Fig. 4a shows the TEM image of freshly prepared CoPTA stabilized copper nanoparticles. The particles are nearly spherical in shape and the size varies from 2 to 3 nm.
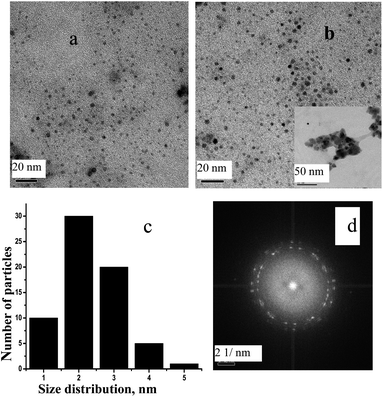 |
| Fig. 4 TEM images of stabilized copper nanoparticles (a) freshly prepared; (b) after one week in inert atmosphere, Inset: controlled copper particles without CoPTA; (c) histogram for size distribution and (d) FFT diffraction of the freshly prepared nanoparticles. | |
Fig. 4b shows the TEM image of CoPTA stabilized copper nanoparticles stored in inert atmosphere after one week. The micrograph showed that the particles were stable even after one week in inert atmosphere without undergoing any aggregation. The inset of Fig. 4b shows the TEM image of copper nanoparticles without the capping agent. These controlled copper nanoparticles are highly aggregated and polydisperse in nature with the size varying from 10 to 20 nm. Fig. 4c shows the particle size distribution histogram of the freshly stabilized copper nanoparticles, which indicates that the average size is around 2–3 nm. Fig. 4d shows the Fast Fourier transform (FFT) diffraction pattern of the stabilized copper particles. The image showed more bright spots in the diffraction pattern indicating that the colloidal copper particles have well-defined crystalline lattices, which were consistent with those of metallic copper.
Fig. 5a shows the TEM image of copper nanoparticles after exposing to air for one day. The TEM image showed aggregation of particles and formation of rod like structures having a length of 30–50 nm and a width of 5–10 nm. TEM images were also taken at different time intervals and are given in the supporting information (Fig. S1†). The extent of formation of rod-like structures increased with time with a decrease in the number of spherical monodispersed particles. The particles were also analyzed for EDAX which showed the presence of copper and oxygen in addition to C, N and Co (quantitative data for elemental analysis are given in Table SI in ESI†) Furthermore, based on the elemental analysis of the sample (Cu = 9.21%, O = 0.78%), the weight Cu/O ratio is 11.8 to the actual value of 8 for Cu2O. This indicates the formation of some copper oxide nanoparticles after exposure to air. Fig. 5b shows the FFT diffraction image of the copper particle. The image showed diffusive ring patterns, which are most probably attributed to the polycrystalline nature of the particle cores. This behavior in the diffraction pattern indicates the transformation of highly crystalline copper nanoparticles into amorphous metal oxide nanoparticles. The Small Area Electron Diffraction (SAED) pattern for different time intervals are given in the supporting information (Fig. S2†). The increase in the nanoparticle size can be attributed to the two phenomena: (1) the expansion of the crystal lattice when metallic copper is oxidized into copper oxide, (2) sacrifice of smaller particles in the formation of jumbo irregularly shaped particles, due to solution-phase Ostwald ripening9 as well as oriented attachment of smaller molecules to form bigger molecules in some specific direction and orientation as shown in Fig. 5. The molecular mechanism is not clear for the formation of these jumbo nanorods. Additional contributions to the formation of these morphologically well-defined nanorods might be from the particle aggregates.
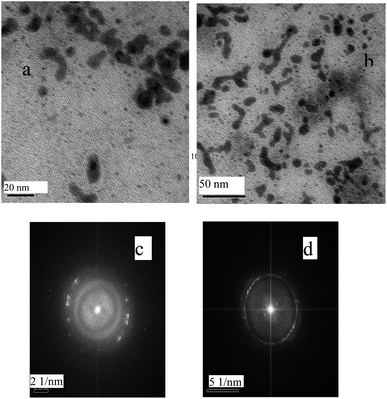 |
| Fig. 5 TEM images of copper nanoparticles after exposing to air for 1 day: (a) 20 nm, (b) 50 nm scale, (c and d) respective FFT diffraction images. | |
X-ray diffraction
The formation of copper nanoparticles is confirmed by a powder X-ray diffractogram. Fig. 6 displays the XRD profile of freshly prepared copper nanoparticles (Fig. 6a) and after exposing the same to air for one day (Fig. 6b). The peak positions in Fig. 6a are consistent with metallic copper (ASTM card no. 4-0836, X-ray diffraction files for inorganic materials). All peaks of copper are observed in the experimental region, indicating the crystalline nature of copper nanoparticles. The X-ray reflections are indexed based on the fcc structure of copper and the diffraction peaks were observed at 2θ values 43.1° and 50.2°, which matches with the literature values.30 The peak that appears at 2θ value 43.1° corresponds to the (111) crystallographic plane and 50.2° to (200) according to JCPDS cards. The average crystalline size of the copper nanoparticles is calculated using the Debye–Scherrer equation given below:
t = 0.9λ/Bcos θ |
where t corresponds to the particle size in Å, λ is the X-ray wavelength, θ is the Bragg angle and B corresponds to the full-width at half-maximum of the peak under consideration. The particle size of the copper nanoparticles determined from the XRD pattern indicates that the particles have a size of 3–5 nm, which correlates with the size obtained from TEM measurements.
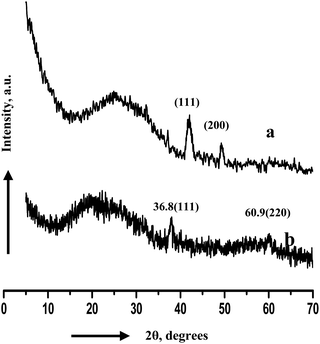 |
| Fig. 6 XRD patterns for copper nanoparticles. (a) Freshly prepared in deaerated atmosphere and (b) after exposing to air for one day. | |
The XRD peaks were also recorded at time intervals of 6, 12 and 24 h after exposing to air. With the exposure of these nanoparticles to air, as expected the intensity of the diffraction peaks due to the copper nanoparticles started decreasing and that of oxide nanoparticles increased with time. The XRD profile after 6 h showed peaks due to copper and also due to its oxidized product, i.e., copper oxide. The XRD pattern taken after 12 h showed peaks of weaker intensity for copper, whereas broader and intense peaks were observed for copper oxide nanoparticles. The XRD profile after 24 h (Fig. 6b) showed broader peaks due to copper oxide nanoparticles at 2θ values 36.8°, 60.9° and very weak peaks were observed corresponding to copper nanoparticles. The 2θ values of copper oxide correspond to (111) and (220) crystallographic planes respectively.11–13 This confirmed the electronic absorption and TEM results of oxidation to copper oxide nanoparticles.
The oxidation of Cu to Cu2O may undergo first, followed by very slow oxidation to CuO.31 The oxidation of copper nanoparticles is incomplete at room temperature even after one day as we can see from EDAX (Table S1 in ESI†) and XRD results. This may be due to the oxide layer, which may protect the metal core from complete oxidation. It is known that the electron affinity of the adsorbate also plays an important role.31 Low electron affinities do not favour the promotion of the ionic surface.
Electrocatalytic reduction of dissolved oxygen
Copper and copper oxide nanoparticles are also compared and characterized for their activity towards electrochemical reduction of oxygen by cyclic voltammetry. Nanomaterials and electrochemistry have a long shared history (e.g., catalysis and fuel cell electrodes).15,16,32–34 Phthalocyanine macrocycles are promising as good electrocatalysts since they are chemically and electrochemically stable, and have been shown to exhibit substantial catalytic activity in a wide variety of redox processes.34,35 Cobalt phthalocyanines have been known to be good catalysts for the reduction of small molecules like oxygen.34,35 The main aim of the oxygen reduction studies is to increase the rate of the electroreduction of oxygen in order to improve the efficiency of the oxygen (air) electrode of the fuel system. Fig. 7 shows the electrocatalytic reduction of oxygen using bare glassy carbon (GC), modified with copper and copper oxide nanoparticles. Currents have been normalized per electroactive catalyst area by deducting the electroactive surface area of the Co complex estimated by the Co redox couple surface electrochemistry in deaerated solutions. The bare GC electrode showed small increasing currents in the negative direction corresponding to oxygen reduction (Fig. 7a). 10 μL of 0.1 mg mL−1 CoPTA modified electrode showed an increase in peak current for O2 reduction (Fig. 7b). The efficiency of oxygen reduction for GC modified with freshly prepared phthalocyanine capped copper nanoparticles was excellent (Fig. 7d). The reduction takes place at lower over potentials (−0.62 V) than that observed on GC modified with CoPTA alone and even almost ten times increase in reduction current is observed. It has been reported that copper nanoparticles show a peak at −0.66 V for ORR36 and have a BET surface area of 50–55 m2 g−1 (ref. 37), which is similar to the BET surface area observed for control copper nanoparticles prepared. The better catalytic activity observed for the CoPTA capped copper nanoparticles is the result of a combined effect of the macrocycle as well as metallic nanoparticles.
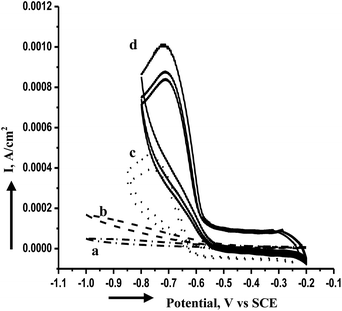 |
| Fig. 7 CVs showing the electrocatalytic reduction of dioxygen with (a) bare GC; GC modified with (b) CoPTA; (c) metallic oxide nanoparticles after exposing the particles to air for 1 day and (d) CoPTA capped copper nanoparticles. The electrolyte is phosphate buffer, pH 7. Scan rate is 50 mV s−1. Currents normalized per electroactive catalyst area. | |
However, the GC modified with copper oxide nanoparticles stored in air for one day also showed an increase in currents (Fig. 7c) compared to bare GC and CoPTA modified GC electrodes, but less than that of copper and at higher overpotentials (−0.75 V). This may be due to the fact that the copper oxide nanoparticles are comparatively less conducting than copper nanoparticles and act as a comparatively lower electron conduction system and a mass transfer blocking layer.38 In the literature, in order to increase the electrocatalytic activity of copper oxide nanoparticles, Nafion® has been used to modify copper oxide nanoparticles on a platinum electrode for sensing hydrogen peroxide, to enhance the ionic and electronic conduction for electron transfer.38 The ability of the modified electrodes to block the electron transfer between the metal surface and soluble redox mediator is a direct measure of the defectiveness associated with the blocking film.39,40 Most active copper nanoparticles probably interact much more strongly with oxygen than their oxide counterparts exhibiting lower activity. In addition, copper nanoparticles offer higher catalytic efficiency due to their large surface-to-volume ratios compared to their oxide counterparts.41 In the case of Cu nanoparticles, the excellent electrocatalytic activity may be due to the high surface area effect as seen by BET surface analysis compared to the copper oxide nanoparticles. The stability and reproducibility of the modified electrodes were examined by potential recycling in the phosphate buffer, Fig. 8a. Copper nanoparticles and their oxide counterparts modified electrode showed 15% and 13% rapid current decrease respectively, after the first scan and the current decrease was comparatively less for the subsequent three cycles (2–6%) and thereafter the current remained almost constant for the next six cycles, exhibiting high resistance to passivation. The plot of peak current versus scan rate is shown in Fig. 8b. A linear relationship between the peak current (Ip) and square root of the scan rate was obtained for both copper and copper oxide modified electrodes indicating diffusion-controlled electroreduction of oxygen.42
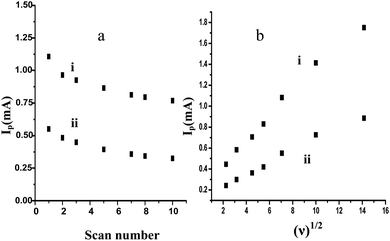 |
| Fig. 8 (a) Plot of variation of peak current (Ip) with scan number for the electrocatalytic reduction of oxygen with (i) copper nanoparticles and (ii) copper oxide nanoparticles. Scan rate 50 mV s−1. (b) Plot of variation of the peak current (Ip) with square root of scan rate (ν) for the electrocatalytic reduction of oxygen with (i) copper nanoparticles and (ii) copper oxide nanoparticles. | |
Experimental details
Materials
Copper chloride (CuCl2), dimethyl sulfoxide (DMSO, 98%), sodium borohydride (NaBH4), sodium sulphide nonahydrate (Na2S·9H2O) were analytical grade reagents purchased from Ranbaxy Chemicals, India. Cobalt tetraamino phthalocyanine (CoPTA) was prepared using a reported procedure.22 Briefly, a slurry of cobalt tetranitro phthalocyanine in water was reduced using sodium sulphide nonahydrate at 50 °C with stirring for 5 h. The bluish green residue is purified and characterized using elemental analysis, UV-visible spectrum and FT-IR spectroscopy before using it for the preparation of copper nanoparticles as a capping agent.
Preparation of nanoparticles
Copper nanoparticles were prepared by modifying the Brust method in a single step process.15,16,43 To a constantly stirred 5 mL 0.05 mmol L−1 solution of CoPTA in DMSO at 10 °C in argon inert atmosphere, 5 mL 0.1 mmol L−1 CuCl2 in DMSO is added. Subsequently, a freshly prepared 5 mL 1 mmol L−1 sodium borohydride (NaBH4) in water is added through a syringe. The color of the solution changed immediately to brownish black from purple. The colloidal solution was stirred for another 30 minutes at the same temperature and atmosphere. Later on, the color of the solution turned green and is found to be stable in inert atmosphere for more than a week. The nanoparticles were subsequently extracted by repeated washing, centrifuging and evaporation. The samples used for characterization were dispersed in dimethyl sulphoxide and ultrasonicated for 10 min before the UV-Vis, TEM and electrochemical experiments.
Characterization
The UV-visible spectra were recorded using a Perkin-Elmer model lambda 35 spectrophotometer. The samples were prepared by dissolving 1 mg of the dry nanoparticles in 5 mL DMSO. A Perkin-Elmer (Spectrum one) FT-IR spectrometer was used to get the IR spectra. FT-IR measurements were made as KBr pellets. Surface area determination was performed by Brunauer–Emmett–Teller (BET) methods using an ASAP 2000 surface area analyzer (Micrometrics Instrument Corp.) During BET surface area analysis, the sample was preconditioned under vacuum (10−3 Torr) for 24 h at 150 °C. Transmission electron micrographs (TEM) were obtained using a TECNAI electron microscope at an operating voltage of 20 kV. X-ray diffraction spectra were recorded at a temperature of 298 K using a Philips PW 1050/37 model diffractometer, operating at 40 kV and 30 mA. Cu Kα radiation with a wavelength of 1.54178 Å and a step size 0.020 in the 2θ range, 5–70 degrees were used. For XRD measurements, the nanoparticles were powdered and spread uniformly on slides. Care was taken to avoid the unnecessary exposure of copper nanoparticles to air. The cyclic voltammetry experiments were carried out using an electrochemical analyzer (CH Instruments, USA), using a three electrode cell system with saturated calomel electrode as a reference, glassy carbon electrode modified with the nanoparticles as working and platinum foil as counter electrode. The glassy carbon electrode (GC) was modified by drop coating 10 μL 0.1 mg mL−1 of the stabilized nanoparticles. The modified electrode is dried in argon atmosphere and used as a working electrode. The electrocatalysis of oxygen reduction has been carried out using the stabilized nanoparticles in phosphate buffer, pH 7.0 at a scan rate of 50 mV s−1.
Conclusion
This paper demonstrates the use of phthalocyanine macrocycles containing amine functional groups as a capping agent to stabilize copper nanoparticles for the first time in a one-phase system. This yields stable powders of nanoparticles that may be readily re-dispersed in different solvents and are highly stable in inert atmosphere. The particles have well-defined edge-to-edge distances of 2–3 nm with spherical shape. These nanoparticles when they are exposed to air slowly undergo oxidation to metallic oxide nanoparticles with the formation of rod-like structures. The slower oxidation may be due to the versatile structure of the phthalocyanine macrocyclic ligand and stronger interaction between the copper and nitrogen atoms of the amine groups on phthalocyanine. Copper nanoparticles in inert atmosphere act as good electrocatalysts for dioxygen reduction compared to their metallic oxide nanoparticles due to their large surface-to-volume ratios in addition to the differences in physico-chemical properties like conductivity, defectiveness of the film, blocking behavior, etc.
Acknowledgements
K. S. Lokesh thanks the CSIR, DST, New Delhi and VGST, Karnataka State Govt. for the funding under young scientist programme.
References
- A. J. Hoffman, G. Mills, H. Yee and M. R. Hoffman, J. Phys. Chem., 1992, 96, 5546 CrossRef CAS.
- V. L. Colvin, M. C. Schlamp and A. P. Alivisatos, Nature, 1994, 370, 354 CrossRef CAS.
- K. S. Lokesh, S. Chardon, F. Lafolet, Y. Traoré, C. Gondran, P. Guionneau, L. Guérente, P. Labbé, A. Deronzier and J.-F. Létard, Langmuir, 2012, 28(32), 11779 CrossRef CAS PubMed.
- R. Elghanian, J. J. Storhoff, R. C. Mucic, R. L. Letsinger and C. A. Mirkin, Science, 1997, 277, 1078 CrossRef CAS.
- B. L. Cushing, V. L. Kolesnichenko and C. J. O'Connor, Chem. Rev., 2004, 104, 3893 CrossRef CAS PubMed.
- E. R. Savinova, E. N. Savinov and V. N. Parmon, J. Mol. Catal., 1988, 48, 231 CrossRef CAS.
- J. B. Reitz and E. I. Solomon, J. Am. Chem. Soc., 1998, 120, 11467 CrossRef CAS.
- J. M. Tarascon and M. Arand, Nature, 2001, 414, 359 CrossRef CAS PubMed.
- S. Chen and J. M. Sommers, J. Phys. Chem. B, 2001, 105, 8816 CrossRef CAS.
- K. Akamatsu, S. Ikeda, H. Nawafune and H. Yanagimoto, J. Am. Chem. Soc., 2004, 126, 10822 CrossRef CAS PubMed.
- W.-T. Yao, S.-H. Yu, Y. Zhou, J. Jiang, Q.-S. Wu, L. Zhang and J. Jiang, J. Phys. Chem. B, 2005, 109, 14011 CrossRef CAS PubMed.
- Y. H. Kim, D. K. Lee, B. G. Jo, J. H. Jeong and Y. S. Kang, Colloids Surf., A, 2006, 285, 364 CrossRef PubMed.
- E. K. Athanassiou, R. N. Grass and W. J. Stark, Nanotechnology, 2006, 17, 1668 CrossRef CAS.
- R. M. Crooks, M. Zhao, L. Sun, V. Chechik and L. K. Yeung, Acc. Chem. Res., 2001, 34, 181 CrossRef CAS PubMed.
- K. S. Lokesh, N. S. Venkatanarayanan and S. Sampath, Microchim. Acta, 2009, 167(1–2), 97 CrossRef CAS.
- K. S. Lokesh, Y. Shivaraj, B. P. Dayananda and S. Chandra, Bioelectrochemistry, 2009, 75, 104 CrossRef CAS PubMed.
- C. Sudeshna, K. S. Lokesh, A. Nicolai and H. Lang, Anal. Chim. Acta, 2009, 632(1), 63 CrossRef PubMed.
- D. C. Hone, P. I. Walker, R. E. Gowing, S. FitzGerald, A. Beeby, I. Chambrier, M. J. Cook and D. A. Russell, Langmuir, 2002, 18, 2985 CrossRef CAS.
- X. Zhang, Y. Wang, Y. Ma, Y. Ye, Y. Wang and K. Wu, Langmuir, 2006, 22, 344 CrossRef CAS PubMed.
- C. Nitschke, S. M. O'Flaherty, M. Kroll and W. J. Blau, J. Phys. Chem. B, 2004, 108, 1287 CrossRef CAS.
- S. Karan and B. Mallik, J. Phys. Chem. C, 2007, 111(20), 7352 CAS.
- B. N. Achar and K. S. Lokesh, J. Organomet. Chem., 2004, 689, 3357 CrossRef CAS PubMed.
- K. S. Lokesh, Bioelectrochemistry, 2013, 91, 21 CrossRef PubMed.
- J. A. Creighton and D. G. Eadon, J. Chem. Soc., Faraday Trans., 1991, 87, 3881 RSC.
- J. Zhu, D. Li, H. Chen, X. Yang, L. Lu and X. Wang, Mater. Lett., 2004, 58, 3324 CrossRef CAS PubMed.
- M. P. Pileni, New J. Chem., 1998, 22, 693 RSC.
- K. S. Lokesh, K. D. Wael and A. Adriaens, Langmuir, 2010, 26, 17665 CrossRef CAS PubMed.
- T. Yokoyama, S. Yokoy, T. Kamikado, Y. Okuno and S. Mashiko, Nature, 2001, 619, 413 Search PubMed.
- J. H. Zagal, F. Bedioui and J. P. Dodelet, N4-macrocyclic metal complexes, Springer, NY, 2006 Search PubMed.
- P. Kanninen, C. Johans, J. Merta and K. J. Kontturi, J. Colloid Interface Sci., 2008, 318, 88 CrossRef CAS PubMed.
- D. B. Penderson, S. Wang and S. H. Liang, J. Phys. Chem. C, 2008, 112, 8819 Search PubMed.
- E. Hosono, S. Fujihara, I. honma and H. Zhou, Electrochem. Commun., 2006, 8, 284 CrossRef CAS PubMed.
- C. M. Welch and R. G. Compton, Anal. Bioanal. Chem., 2006, 384(3), 601 CrossRef CAS PubMed.
- A. B. P. Lever, M. R. Hempstead, C. C. Leznoff, W. Liu, M. Melnik, W. A. Nevin and P. Seymour, Pure Appl. Chem., 1986, 58, 1467 CrossRef CAS.
- K. S. Lokesh, M. De Keersmaecker and A. Adriaens, Molecules, 2012, 17, 7824 CrossRef CAS PubMed.
- T. Selvaraju and R. Ramaraj, Pramana, 2005, 65, 713 CrossRef CAS.
- http://www.americanelements.com/cunp.html.
- X.-M. Miao, R. Yuan, Y.-Q. Chai, Y.-T. Shi and Y.-Y. Yuan, J. Electroanal. Chem., 2008, 612, 157 CrossRef CAS PubMed.
- C. E. D. Chidsey and D. N. Loiacono, Langmuir, 1990, 6, 682 CrossRef CAS.
- S. E. Creager and K. G. Olsen, Anal. Chim. Acta, 1995, 307, 277 CrossRef CAS.
- R. Narayan and M. A. El-Sayed, J. Phys. Chem. B, 2003, 107, 12416 CrossRef.
- A. J. Bard and L. R. Faulkner, Electrochemical Methods: Fundamentals and Applications, John Wiley, New York, 2nd edn, 2001, p. 231 Search PubMed.
- M. Brust, M. Walker, D. Bethell, D. J. Schiffrin and R. Whyman, J. Chem. Soc., Chem. Commun., 1994, 7, 801 RSC.
Footnote |
† Electronic supplementary information (ESI) available. See DOI: 10.1039/c3ra42682c |
|
This journal is © The Royal Society of Chemistry 2014 |