Productivity of aquatic primary producers under global climate change
Received
3rd December 2013
, Accepted 25th July 2014
First published on 8th August 2014
Abstract
The productivity of aquatic primary producers depends on a number of biotic and abiotic factors, such as pH, CO2 concentration, temperature, nutrient availability, solar UV and PAR irradiances, mixing frequency as well as herbivore pressure and the presence of viruses, among others. The effects of these factors, within a climate change context, may be additive, synergistic or antagonistic. Since some of them, e.g. solar radiation and temperature, vary along a latitudinal gradient, this perspective about the effects of global climate change on primary producers will consider ecosystems individually, separated into polar (Arctic and Antarctic), temperate and tropical waters. As coastal waters are characterized by lower light penetration and higher DOM and nutrient concentrations, they are considered in a separate section. Freshwater systems are also governed by different conditions and therefore also treated in their own section. Overall, we show that although there are general common trends of changes in variables associated with global change (e.g. the impact of UVR on photosynthesis tends to decrease with increasing temperature and nutrient input), the responses of aquatic primary producers have great variability in the different ecosystems across latitudes. This is mainly due to direct or indirect effects associated with physico-chemical changes that occur within water bodies. Therefore we stress the need for regional predictions on the responses of primary producers to climate change as it is not warranted to extrapolate from one system to another.
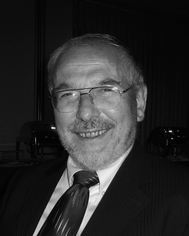 Donat-P. Häder | Prof. Dr Dr h.c. Donat-P. Häder is an emeritus from Friedrich-Alexander University, Biology Dept., Erlangen, Germany. He obtained his Ph.D. from the University of Marburg (Germany), received a Ph.D. honoris causa from Univille (Santa Catarina, Brasil) and held the chair of ecophysiology in Erlangen. He was the director of the institute and the Botanical Garden. He is currently an expert in a UNEP panel on the effects of ozone depletion and global climate change. His scientific interests are in ecophysiology and photobiology of aquatic ecosystems and graviorientation of microorganisms. |
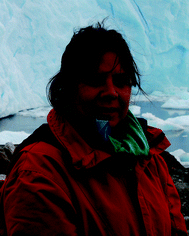 Virginia E. Villafañe | Dr Virginia E. Villafañe is currently a researcher from Consejo Nacional de Investigaciones Científicas y Técnicas (CONICET, Argentina). She obtained her Ph.D. from the University of Groningen (The Netherlands) and continued her research in Patagonia at Estación de Fotobiología Playa Unión (EFPU, Argentina). Dr Villafañe's scientific interests are in the areas of ecophysiology of plankton and photobiology. |
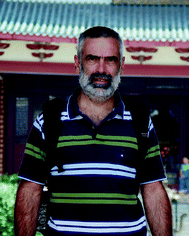 E. Walter Helbling | Dr E. Walter Helbling is currently the Director of Estación de Fotobiología Playa Unión (EFPU, Argentina) and a researcher from Consejo Nacional de Investigaciones Científicas y Técnicas (CONICET, Argentina). He obtained his Ph.D. from the Scripps Institution of Oceanography (UCSD, USA). His scientific interests are in the ecophysiology of plankton and the photobiology of aquatic systems in relation to climate change. |
Introduction
More than 70% of our planet is covered by water, but less than 1% of this is freshwater.1 The overwhelming share constitutes the vast marine ecosystems. In addition to their role in regulating the global climate by sequestering atmospheric CO2, marine ecosystems are major producers of human food sources including fish, crustaceans, mollusks and others.2,3 During the last 50 years, world fish production has increased faster than global population growth, and today fish is an important source of animal protein for much of the human population.4 Recreation and tourism is another significant aspect of marine ecosystems. For example, coral reefs have been estimated to generate an annual benefit of 9.6 billion US$.5,6 The basis of the marine food web is constituted by primary producers (Fig. 1), i.e. mainly phytoplankton, cyanobacteria and macroalgae that are the food source for primary and secondary consumers.7,8 Even though the biomass (standing crop) of these marine organisms equals only about 1% of all plants in all terrestrial ecosystems, their productivity rivals that of the combined biomass production on land.9 Other organisms have great significance in aquatic habitats, for example viruses are the most abundant biological particles in the sea10 and especially viruses11 play important roles in the structure and dynamics of aquatic ecosystems such as controlling phytoplankton populations.12 Bacteria also have a key role in the microbial loop and carbon fluxes in the oceans.13
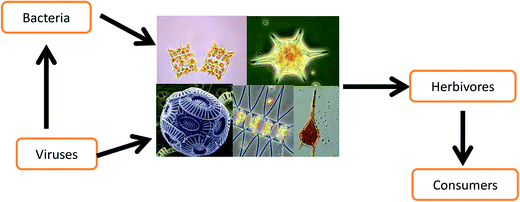 |
| Fig. 1 Interconnections between bacterio- and virioplankton with phytoplankton as well as the input from the primary biomass producers to primary and secondary consumers. | |
The marine ecosystems are a major sink for atmospheric carbon dioxide and take up a similar amount of CO2 as all terrestrial plants together and thus are a major player in the regulation of the atmospheric CO2 concentration.14a While the CO2 concentration in the atmosphere was estimated to be about 270 ppm before the industrial revolution it has currently increased to about 400 ppm.14b Without the constant uptake of CO2 by the marine primary producers this value would be higher since about 25% of the carbon fixed in the upper oceans sinks to the deep sea15 when the microorganisms decay or are eaten by primary and secondary consumers in the form of fecal pellets16 in a process known as the biological pump. The increasing CO2 concentration in the atmosphere might augment the growth and photosynthesis of phytoplankton.17,18 However, at the same time it causes acidification of the water19,20 and this anthropogenic impact on the ecosystem could be a threat for phytoplankton.21 Additionally, the increasing CO2 concentration,22 which affects the CO2 availability to the phytoplankton and also decreases the pH of the surface water, affects enzymatic and other biochemical processes as well as calcification in phytoplankton, macroalgae and animals with aragonite or calcite exo- or endoskeletons.23–26 On the other hand, high concentrations of phytoplankton may be a partial solution to acidification, because the organisms take up the CO2 during their photosynthetic activity.27
Primary productivity of marine organisms depends on a number of abiotic factors, with solar radiation being an essential environmental variable driving photosynthesis. Primary producers populate the euphotic zone, often defined as the water column between the surface and a depth where the surface irradiance has decreased to 1%, which allows positive net daily photosynthesis.28 Terrestrial plants, as well as macroalgae, seagrasses, etc. attached to the bottom of an aquatic system, are confined to a habitat with a defined light level: in contrast, phytoplankton has to adapt to a wide range of irradiances within short periods of time as it is subject to constant movement within the mixing layer due to the action of wind and waves.29 This passive movement is often superimposed by active vertical migration using flagella or changing their buoyancy.30
In addition to changes in the photosynthetic active radiation (PAR, 400–700 nm) these organisms are exposed to variable levels of UV radiation (280–400 nm) which can have detrimental effects on various morphological, physiological and genetic processes in the cells.2 The severity of the effects caused by UV radiation varies with the taxonomic group, habitat and developmental stage, and planktonic organisms are affected to a larger degree than other groups.31 In particular, effects such as reduction in primary production/photosynthesis rates, growth, calcification and disruptions in membranes have been determined.32 Since shorter wavelengths are more attenuated than longer ones due to Rayleigh scattering, UV-A (315–400 nm) can be more detrimental than UV-B (280–315 nm) radiation, even though the latter has higher damaging potential;33 particularly UV-B is responsible for damaging the DNA molecule, via the production of cyclobutane pyrimidine dimers (CPDs).34,35 However, there are variable responses, not only due to a high degree of species-specificity, but also due to the previous light history, for example when comparing phytoplankton of similar taxa (several chlorophytes and diatoms) isolated from tropical, temperate and Antarctic habitats, by exposing them to increasing doses of UV-A and UV-B, significant differences were found.36 UV-A did not have an effect even at the highest doses applied, but UV-B inhibited growth. Thus the sensitivity depended on the species and on the biogeographic origin. Out of nine species, the Antarctic Chlorella was the least sensitive. Bacteria and viruses were found to be more affected by UV radiation than phytoplankton due to the fact that small cells cannot protect themselves from excessive short-wavelength radiation with reasonable concentrations of photoprotective compounds due to size restrictions.37 This was confirmed for virioplankton in a transplant experiment simulating different latitudes. However viruses can adapt through evolutionary processes to different environmental conditions, such as UV radiation exposure.38 In general, little is known about the effects of changing climate parameters on the virioplankton populations and virus–phytoplankton interactions.39 While viruses are prone to be affected by UVR via radiation-induced DNA damage, they may obtain some protection from their hosts.40 In bacteria, it was found that UV radiation exposure results in significant changes in the species composition of the communities.41
Detrimental effects of solar radiation on primary producers can be minimized by a number of mechanisms, among which the repair of damage, and synthesis of UV-absorbing compounds (mainly mycosporine-like amino acids, MAAs) are the most frequently found. Animals are not capable of MAA synthesis, but they take up these UV-absorbing substances with their food and use them for the same purpose by storing them in their outer cell layers.42 In addition to their function as UV-absorbing pigments, MAAs were found to serve as antioxidant molecules scavenging toxic oxygen radicals.43 Phytoplankton also uses other protective mechanisms to scavenge UV-induced oxidants, e.g. superoxide dismutase, ascorbate peroxidase and glutathione.44,45 Also vertical mixing is essential in mitigating UV-induced photoinhibition as it allows the phytoplankton to repair damage to cellular compartments such as the DNA and the photosynthetic apparatus while being at the bottom of the mixing layer and therefore less exposed to intense solar UV.46 Additionally, many terrestrial and aquatic animals as well as some higher plants are known to show compensatory growth after periods of reduced growth due to stress such as UV radiation exposure or darkness. Recently it was confirmed that this phenomenon also exists in the marine diatom Phaeodactylum tricornutum after being exposed to UV radiation.47,48
The ambient water temperature is another important external factor governing cellular productivity49 since most biochemical processes are temperature-dependent. Only a few organisms can achieve net growth below freezing, and increasing temperatures result in higher productivity rates via a species-specific optimum up to a maximal permissive temperature.50 Different taxa of primary producers have different abilities to acclimate to changing growth conditions such as temperature51 which would result in different responses52 including changes in the species composition with extensive consequences for the whole marine food webs. Increasing temperatures also support the occurrence of harmful algal blooms, many of which belong to the dinoflagellates.
Fossil records of dinoflagellate cysts indicate that El Niño events and changes in the North Atlantic Oscillation affect the population density of these phytoplankton groups by altering surface stratification, ocean currents and accessibility of nutrients.53 Even when the supply of inorganic nitrogen is limited, blooms of toxic algae can develop, fed by a large pool of dissolved organic nitrogen (DON).54 Another source of nitrogen can be submarine groundwater discharge.55 In contrast, nitrogen limitation results in reduced biomass production and decreased synthesis of biogenic sulfur compounds such as DMS, DMSP and DMSO.56 Phosphate limitation causes reduced alkaline phosphatase activity in phytoplankton and bacteria.57
Further environmental factors affecting the productivity of marine ecosystems are salinity,58 pH,59 nutrient availability,60 competition between producers61 and herbivore pressure.62 Most of these factors are subject to marked changes caused by climate changes such as increasing temperature, ocean acidification, changes in nutrient availability due to changing oceanic streaming patterns and terrestrial runoff22,63 as well as increasing exposure to detrimental solar UV-B radiation due to stratospheric ozone depletion.64
Even though the external factors such as temperature, pH, CO2 supply, PAR and UV irradiances and mixing depths are known to be primary variables driving photosynthesis and production (Fig. 2), their interactions have received relatively little attention for both plankton65 and sessile organisms.66 This interacting web can only be disentangled by multifactorial analysis.67–69 In addition, most studies have been carried out under laboratory-controlled conditions in short-term experiments.70,71 In order to reveal the effects in real nature with its fast changing temperature, solar radiation and availability of nutrients, further studies need to be carried out in the open ocean with ecologically relevant values for the essential parameters.22,72 However, this is difficult and time-consuming regarding the vast areas to be covered and the low concentrations of cells in the water column. In order to evaluate the effectiveness of physiological and genetic adaptation to the changing growth conditions under a climate change scenario induced by anthropogenic activities, long-term studies together with multi-generation experiments need to be devised to understand how phytoplankton will cope with the various stress factors and take advantage of favorable conditions.73
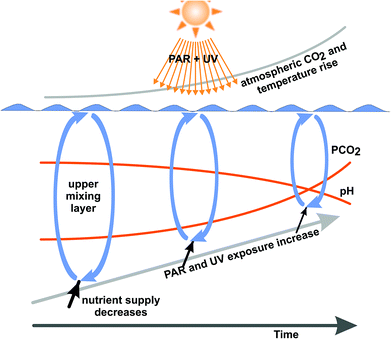 |
| Fig. 2 Environmental factors due to anthropogenic activities, including atmospheric CO2, ocean acidification, temperature increase with reduction of the upper mixing layer and resulting increased exposure to solar UV and PAR are expected to be responsible for changes in future phytoplankton communities and marine primary production (adapted from ref. 22). | |
The impact of increasing temperatures on phytoplankton communities due to anthropogenically induced climate change is fairly well understood74 and trends in abundance and shifts in the taxonomic composition are documented. However, the complex network of feedback loops,75 changes in ocean chemistry and circulation patterns as well as the impact of tropical storms76,77 need to be investigated since they will affect productivity and population dynamics with significant consequences for fisheries and climate development.78,79 Many of the phytoplankton may not be impacted by the increase of CO2 alone, but others, such as the ecologically important coccolithophorids, show a significant stimulation in growth.80 Simultaneous increases in temperature and CO2 in conjunction with increased UV radiation and nutrient limitations81 were found to result in increasing cell sizes and elemental stoichiometry which are reliable predictors for tracking changes in the phytoplankton community structure and trophic dynamics.82 Similarly, the inhibition of phytoplankton photosynthesis/growth by UV radiation cannot be seen as an isolated phenomenon as other factors such as temperature and grazer pressure play important additive, synergistic or mitigating roles in phytoplankton dynamics.83
Moreover, the effects of many feedback mechanisms on marine primary producers are largely unknown. Do higher temperatures of the oceans result in denser cloud covers? This could result in lower exposure of the phytoplankton to solar UV and PAR. Many phytoplankton and macroalgae are known to produce dimethylsulfoniopropionate (DMSP),84 an organosulfur compound (CH3)2S+CH2CH2COO− that acts as an osmolyte or as an antioxidant.85 This zwitterionic metabolite is excreted and partially broken down to dimethylsulfide (DMS, CH3SCH3).86,87 DMS enters the atmosphere where it reduces the incoming solar radiation and forms cloud nuclei.88,89 DMSP production and DMS release are correlated with sea ice melting.90 The environmental advantage of DMSP-producing phytoplankton due to increased temperature could further complicate the feedback mechanisms involving cloud formation. Another expected climate feedback implication is the effect of nitrogen limitation on phytoplanktonic DMSP production and DMS release.91
As gradients of the most important factors affecting the primary productivity, i.e. radiation and temperature, occur in a latitudinal sense, we choose to organize this perspective considering such gradients starting with polar (both Arctic and Antarctic) waters, and then continuing with temperate and tropical communities. Since coastal habitats differ from open ocean ecosystems in a number of environmental factors they are considered in a separate section. The final section covers freshwater ecosystems.
Phytoplankton in the Arctic Ocean
The Arctic Ocean covers an almost circular basin with about 14 million square kilometers surrounded by the Asian, European and American continents, with a small gap connecting to the Pacific Ocean via the Bering Strait, and another connection to the North Atlantic, with 45% of this area covered by permanent ice.92 Increasing temperatures due to global warming, however, have decreased the ice cap dramatically over the last few decades by 49% during the summer as compared to the average area between 1979 and 2000.93–96 The ice sheet also became much thinner and the total volume of ice in 2012 was only about 25% of that in 1979.97 The temperature increase of the global oceans was about 1 °C over the last 112 years (Fig. 3).98 The rise in Arctic near-surface air temperatures has been almost twice as large as the global average in the past 2–3 decades.99 This significant temperature increase is due to a feedback mechanism: while ice and snow reflect most of the incident solar radiation back into space, water and soil, which are no longer covered by ice, absorb most of this radiation causing a substantial warming.93 As a consequence, summer melting occurs earlier and freezing later in the year and these not only produce a feedback effect in the recovery of the polar stratospheric ozone,100 but also cause dramatic changes in the penetration of solar radiation in the water column.101 The significant temperature increase augments the phytoplankton productivity and also affects the distribution of many plankton organisms. For example, tropical radiolaria have intruded into Arctic waters.96,102 These plankton organisms were found in 2010 during a cruise northwest of Svalbard. Of the 145 taxa identified, 98 had probably been introduced with a pulse of warm Atlantic water which entered the Norwegian Sea and finally ended up in the Arctic Ocean. The tropical species even had different developmental stages indicating that they were reproducing here indicating increased ambient temperatures.
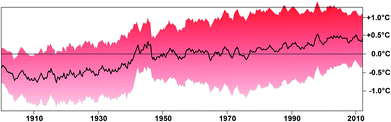 |
| Fig. 3 Global ocean surface temperatures (top 1 m) as compared to the average from 1900 to 2012 based on data compiled by Marinexplore (Sunnyvale, Calif). Redrawn from ref. 98. The gray area indicates the margin of error. The pronounced higher temperatures in the 1940s are an artifact due to the unavailability of data from Russia and Europe. | |
Phytoplankton concentrations are significantly higher in polar regions (both Arctic and Antarctic) than at mid or equatorial latitudes as seen from satellite imaging showing the chlorophyll fluorescence (Fig. 4).103 The result of earlier melting and later freezing of the open water surface has prolonged the season for phytoplankton blooms27 and also started about 50 days earlier than before.104 Receding ice during the summer results in phytoplankton productivity further north, and this development attracts more fish. The Atlantic cod feeds on capelin, which used to have a maximal distribution south of Svalbard (75 °N) in 2000. In 2012 they had moved to 78 °N and the cod followed them.105 Since the blooms are seeded from organisms entrapped in the ice over winter, the densest phytoplankton populations are found in the marginal ice zone within 100 km from the melting ice edge.106 The peak of the blooms occurs within 20 days after the water has become ice-free. This is supported by strong storms which increase the concentration of nutrients in the photic zone. An evaluation of fluorescence data derived from satellite measurements has shown a 20% increase in the net primary productivity in the open water of the Arctic Ocean between 1998 and 2009.107 Usually nitrogen is limited in these waters, while phosphate and silicate are relatively abundant, but the melting sea ice contains about four times more nitrogen than the water.108 These marginal sea-ice blooms have been confirmed for the Bering Sea, the Chukchi Sea and the Barents Sea.109–111 Later in the growing season there may be a second bloom, when the water has heated up to support thermal stratification.112 However, stratification also limits nutrient availability, since nutrient-rich bottom water is not easily mixed into the upper mixing layer (UML).113
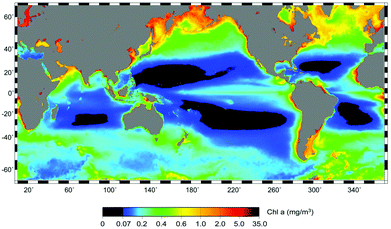 |
| Fig. 4 Surface chlorophyll a derived from satellite measurements (NOAA http://www.noaanews.noaa.gov/stories2008/20080305_oceandesert.html). | |
Similar to phytoplankton in the water column, ice algae, that account for ca. 50% of the biomass in Arctic waters, also showed earlier growth than before, together with shifts in plankton species communities towards smaller cell types.27 This selection of smaller phytoplankton types may be due to the low solar irradiances, which are more efficiently absorbed by small cells.114,115 Recent studies carried out in the Chukchi Sea off the coast of Alaska in 2012 discovered an unprecedented giant plankton bloom under the Arctic ice shield.116,117 The concentration of the phytoplankton was the highest ever recorded even in open waters. The blooms under the ice could not have been detected by remote sensing satellite-based fluorescence measurements. In some cases the blooms extended up to 50 m depth and more than 110 km from the edge under the ice. The melt water from the ice forms pools and puddles on the surface of the ice sheet which serve as “skylights”, where the light penetrates through the melt puddles almost unobstructed while the surrounding ice and snow are opaque.118a These pools focus sunlight through the ice and into the waters below. The penetrating light amounts to about 50% of the incident solar radiation,119 allowing a massive growth of phytoplankton blooms underneath, further supported by nutrient-rich water under the ice.118a Under normal conditions polar sea ice transmits only a few percent of the incident solar radiation.118b If this phenomenon is more widespread in the Arctic Ocean, the phytoplankton would constitute a massive sink for atmospheric CO2. The thinner ice sheet, earlier melting and later freezing expose the phytoplankton to higher solar visible and UV radiation. This is partially offset by increased terrestrial runoff with high DOM content (dissolved organic matter) which reduces the transparency.120,121 Melt water from sea ice and glaciers dilutes the sea water and reduces its salinity which may have far-reaching consequences for the food web since it changes the species composition and the abundance of phytoplankton.122 Freshwater input in conjunction with the temperature increase enhances stratification shoaling the upper mixed layer and limiting the nutrient availability by reduced mixing between the UML and the water column underneath,123 and this also favors smaller phytoplankton which has impacts on the food web via different food quality and size preferences of the food.124
Another factor damping the phytoplankton primary production in the Arctic is the reduction of solar radiation due to increasing cloudiness.125 The presence of grazers may also affect phytoplankton primary production.126 However, grazers (<500 μm) did not affect significantly the phytoplankton productivity of bloom-forming communities (i.e. Phaeocystis pouchetii) as determined along a glacier-to-open sea transect in the Greenland subarctic fjord Godthåbfjord.127 Visible light and UV radiation exposure also have important effects on the size structure of primary producers: picoplankton is more affected by solar radiation than nanoplankton, which could be explained by their smaller size, as shown in a mesocosm study in an Arctic fjord.128 A similar change in phytoplankton communities due to UV pressure has been found in Arctic freshwater habitats.129
Additional anthropogenic stress factors are increasing environmental pollution. Especially in the Arctic shallow-water marine habitats, crude oil spills have been found to affect algae and bacteria. For example, pyrene, which is a component of crude oil in the sediment, has been found to exert a synergistic negative effect with increased solar UV-B radiation.130 Phytoplankton has also been found to accumulate persistent organic pollutants (POP) in the Greenland Current and Arctic Ocean as documented during the ATOS-ARCTIC cruise.131
Phytoplankton in the Southern Ocean (Antarctic)
In contrast to the Arctic, which is a closed basin, the Southern Ocean surrounding the continent is open to the Southern Pacific, the Southern Atlantic and the Southern Indian Ocean. The Antarctic continent is covered with ice and most of its coastline is hidden under ice flowing into the sea. Low temperatures are one factor for limiting photosynthesis and growth, but a more decisive one is light limitation.132,133 Furthermore, phytoplankton growth in the Southern Ocean, away from the continental shelf, is limited by low concentrations of nutrients such as iron, nitrate and silicate.134,135 In fact, iron and temperature have a synergistic effect on Antarctic phytoplankton and microzooplankton communities.136 The major source of iron is from melting ice, Antarctic rocks and dust deposition.137,138 In order to spur productivity, experiments have been conducted in which iron was sprayed onto the water.139 This fertilization in fact resulted in a pronounced bloom of diatoms, taking up large amounts of CO2 from the atmosphere. However, these experiments are controversial, since further studies have indicated that the diatoms absorbed more iron into their silica shell than necessary for photosynthesis, depleting the Fe stock in the water.140
Antarctic shelf zones are generally very productive areas, and when the ice melts in spring nitrate, phosphate and silicate levels are high and allow vigorous growth.141,142 As in the Arctic, in spring and early summer phytoplankton seeded from stocks frozen into the ice rapidly multiply forming a very dense population which covers thousands of square kilometers.143,144 These dense blooms are several hundred times more concentrated than in mid-latitude oceans and provide food for primary consumers, mainly copepods,145 krill146,147 and salps.148 The amount of krill biomass has been estimated to exceed the weight of all human beings.149 Ammonium and iron excretion from krill (Euphausia superba) is an important supply for phytoplankton.150 The dominant phytoplankton species are Phaeocystis antarctica (in deeply mixed waters) and diatoms (in highly stratified waters).151 Due to the high nutrient supply from upwelling water152 and the long daylight hours biomass of phytoplankton and that of the following levels in the food web increase during the summer period. Physical gradients in the coastal waters and different stratified conditions are important external factors controlling the size structure and species composition in Antarctic phytoplankton.153,154 Phytoplankton standing stocks and carbon assimilation rapidly increase during spring, reach a maximum in summer and decrease during autumn and are close to zero in winter.155,156 During the winter in the Southern Atlantic the plankton is dominated by picoplankton (<2 μm) which represents 99% of the biomass. When the water temperature was below 1 °C mainly eukaryotes were found while above 1.3 °C cyanobacteria prevailed, such as Synechococcus. In contrast, Prochlorococcus was only found when the temperature exceeded 10 °C.157
Judging from 30 years of chlorophyll fluorescence data and field studies, densities of phytoplankton communities have dropped by 12% along the West side of the Antarctic Peninsula (Bellingshausen Sea) due to the rapid regional climate change.158 The previously dry and cold climate became warmer and more humid, and it has long been observed that the Antarctic Peninsula is heating faster than any other part of the southern hemisphere. Winter temperatures on the Antarctic Peninsula have risen five times faster than the global average over the past 50 years, and are expected to do so in the future.159 As a result, the sea ice cover has decreased dramatically allowing more wind mixing of the water column and more cloudiness, leading to decreased light levels and consequently less photosynthetic biomass production. In contrast, further south there are less mixing, fewer clouds and higher phytoplankton productivity.160
Heating of the water due to global warming has affected the base of the Antarctic food web. The accelerated warming resulted in a substantial loss of shelf ice and a significant input of freshwater into the sea. This dilution and reduction of salinity has far-reaching effects on the species composition of phytoplankton, bacteria and other components of the food web.161 This was confirmed by the marked changes in phytoplankton composition during the onset of Antarctic glaciations in the early Oligocene (33.5 million years ago) as indicated by the fossil record of marine dinoflagellate cysts.162 Predictions for the future posit a gradual loss of marine ice algae due to decreasing habitats as the sea ice disappears causing a cascade through the higher trophic levels of the food web; however, this is not regarded likely within the next 100 years. In contrast to the Arctic and the Antarctic Peninsula, the rest of the Antarctic continent has not experienced a marked warming.163
The Antarctic ecosystem is in a specific stress situation. Starting in the late 1970s a marked depletion of the stratospheric ozone has been observed developing during spring.164 This is due to specific chemical and physical conditions. Anthropogenically derived chlorofluorocarbons (CFC) and other trace gases accumulate in the stratosphere and are circled in a vortex over the Antarctic continent. These molecules trigger a catalytic destruction of ozone (O3) exceeding 50% loss.165 Since O3 is an effective absorber of solar UV-B radiation this energetic short-wavelength radiation reaching the water surface is dramatically increased. Even though UV-B radiation amounts to only about 1% of total solar radiation, it exerts damaging effects on different molecular targets in phytoplankton cells. Therefore a substantial increase in solar UV-B due to stratospheric ozone depletion can be assumed to have a marked effect on Antarctic phytoplankton primary production during ozone hole episodes.166,167 Other studies have shown that UV radiation caused other effects such as reduction of growth, changes in the taxonomic composition in Antarctic phytoplankton168 but these organisms have shown some capability to acclimate to solar UV radiation by the synthesis of ultraviolet-absorbing compounds.169,170
While a 25% of UV-afflicted inhibition of growth in the upper water column has been determined in Southern Ocean waters,171 Smith et al.166 calculated a 2% decrease for the annual primary production. In situ incubation of natural phytoplankton communities indicated that outside the ozone hole solar UV-B is responsible for about 4.9% inhibition of primary production, while under ozone hole conditions (150 Dobson Units) the inhibition rose to 8.7%.172 In contrast, Hamre et al.173 even found an enhanced aquatic primary production by 1% for 50% stratospheric ozone depletion. Antarctic coastal phytoplankton assemblages are generally much less sensitive to UV radiation than open ocean communities,174 with also higher rates of repair than pelagic communities. Variable irradiance conditions, as experienced by phytoplankton in the UML, were of key importance at the time to determine the impact of solar UVR. Helbling et al.175 determined that the combination of solar UVR and vertical mixing decreases the primary productivity of Antarctic phytoplankton. Later studies proposed a model showing that fast vertical mixing was more important than the low total column concentration in decreasing the primary productivity of phytoplankton in the Antarctic.176 This was related to the low temperatures in the Southern Ocean and the low repair capacities as compared to other ecosystems. Increasing global temperatures would have an important effect on polar phytoplankton by increasing metabolic rates and thus repair mechanisms to cope with solar UVR.
Phytoplankton from temperate ecosystems
In contrast to polar or tropical aquatic ecosystems, temperate environments undergo great changes in physical factors such as temperature, wind, etc.177,178 At temperate latitudes phytoplankton communities present seasonal cycles of abundance and species composition.179 These are mainly driven by factors varying seasonally,179 acting directly or indirectly, antagonistically, synergistically or additively.68 These factors include temperature (which affects biochemical and enzymatic processes such as SOD activity,180 and photosynthetic processes,181 among other things), light availability182 and the impact of excessive visible and UV solar radiation.183,184 For example, as the temperature increases towards the summer, stratification tends to increase, decreasing the thickness of the UML.185 In addition, many other external factors including water currents, pH,186 DOM concentration,187 salinity, mixing layer depth188 and nutrient availability like nitrogen and iron189 vary seasonally and affect phytoplankton development and abundance.190,191 In temperate environments, however, wind is perhaps the most important factor controlling the stratification of the water column and together with this, the development of winter blooms which favored large diatoms, while pre- and post-bloom periods were characterized by small (<10 μm) flagellates, as seen in Patagonian waters.179,192 Harmful red tide dinoflagellate blooms are also an important issue in temperate marine habitats, especially in coastal and estuarine ecosystems. These blooms have been found to increase with increasing nutrient availability, e.g. from terrestrial runoff. They are further enhanced by rising temperatures53,193 and spread globally by being carried by marine transport in ballast water.194
Rising temperatures due to global climate change are expected to affect temperate phytoplankton most pronouncedly during winter and early spring while the effects will be much smaller during the rest of the growth season.191 However, higher temperatures moderate the inhibition of carbon fixation and photochemical quantum yield, as shown for the cosmopolitan diatom Thalassiosira weissflogii.184,195 One of the reasons for this is a significantly higher RUBISCO activity and gene expression of this enzyme at 25 °C than at 20 °C. Therefore, this mechanism could reduce the UV stress on phytoplankton in a global climate change scenario. In contrast, a mesocosm experiment in the Saint Lawrence Estuary indicated that higher temperatures impair the cell cycle resulting in lower growth rates and cell concentrations in natural phytoplankton communities.196
Changes in stratification and in the UML depth will affect not only phytoplankton production, by changing the exposure of cells to solar radiation, but also to higher trophic levels such as fish from temperate zones.178 Under mixing conditions, UV-A can even have positive effects on growth, as it can be utilized for photosynthetic energy harvesting.197,198 However, the extent of UV effects strongly depends on the phytoplankton community composition as shown for natural assemblages in a temperate estuarine ecosystem.199 For example, cryptomonads were found to be significantly more sensitive to UV radiation than the common estuarine diatom Thalassiosira. Levels of MAAs have been found to increase with rising exposure to solar radiation. The occurrence of specific MAAs can be correlated with the advent of key phytoplankton species in the water column. For example, in the English Channel the concentration of mycosporine–glycine was found to increase in parallel to the occurrence of a Phaeocystis bloom in spring.200 Later in the year an unidentified MAA (λmax 328 nm) was found to match a bloom of the diatom Guinardia striata. The strong correlation between solar UV radiation and MAA concentration was proven by monitoring the daily variation in the cellular concentration of MAAs in the marine dinoflagellate Scrippsiella independent of cell volume or chlorophyll a concentration.201
The sensitivity to UV-B of temperate phytoplankton assemblages was found to be dependent on the nitrogen (and also phosphate and silicate) availability.202 As long as sufficient nutrients were available within the mesocosms UV-B effects were minimal but the cells suffered from significant photodamage to the PSII reaction center, monitored as a decrease in the D1 protein pool, when nutrient supply was limited.203 Also, in a survey on carbon fixation of natural summer phytoplankton assemblages from Patagonia high photosynthetic efficiency was found favored by the input of inorganic nutrients from the Chubut River which discharges close to the site of plankton collection.204 In natural phytoplankton communities the effects of solar UV-B on the photodegradation and repair of the PS II D1 protein were studied during a 5-year multidisciplinary project.205 These studies showed that the inhibition of the repair cycle by UV-B is more important than the original damage of the protein. Biochemical analysis indicated that the phosphorus supply, irradiance and temperature affect the fatty acid concentration in chlorophytes, cryptophytes and diatoms.206
Large-celled diatoms have a higher capacity to withstand and exploit high light irradiances.207 Small-celled phytoplankton such as the cyanobacterium Prochlorococcus and picoeukaryotes are more prone to UV-B-induced damage than larger cells.208–210 This was confirmed by exposing Atlantic picophytoplankton communities to natural levels of solar radiation.210–213 Even when using an experimental approach to simulate vertical mixing, solar UV radiation was found to affect microplanktonic diatom species (Odontella aurita) during the summer bloom and nanoplanktonic flagellates and Chaetoceros after the summer bloom in a temperate South Atlantic location (43 °S).197 Further in situ experiments at the same area confirmed that solar radiation induced DNA damage and caused inhibition of photosynthesis in the top 3 to 6 m.214
The concentration of phytoplankton strongly depends on the pressure by predators. In a study on the seasonal abundance and feeding patterns of copepods in a pelagic food web in the White Sea up to 85% of the phytoplankton standing crop was consumed by the calanoid copepods, playing a significant role in the transformation of particulate organic matter.215 As also seen in freshwater and tropical habitats, the extent of UV-B stress on phytoplankton affects the palatability of these cells during zooplankton grazing.216 While Daphnia grazing was not affected by UV-B stress on Chlamydomonas, Microcystis, Cryptomonas and Scenedesmus, Brachionus grazing rates decreased.
Tropical marine habitats
In contrast to temperate and polar areas, where the UML can exceed 100 m of depth, tropical aquatic systems tend to have a strongly stratified and stable mixed layer, driven by temperature, and typically restricted to the top 10–20 m.185 This stability in physical–chemical factors is evidenced in the low variability in phytoplankton species composition in the tropics.217 Most tropical waters are exceptionally clear, since the density of phyto- and zooplankton is very low due to limited nutrient supply in these oligotrophic waters. These low nutrient conditions favor growth of small-sized cells, and although numerous cruises confirmed a strong variability in phytoplankton chlorophyll concentrations in the Eastern North Atlantic Subtropical Gyral Province, they indicated that 54% of the primary production was contributed by cells <2 μm.218 Below the marked pycnocline nutrient concentrations are considerably higher and the irradiances are lower, resulting in a distinction of phytoplankton organisms populating the highly lit, but nutrient-deficient mixing layer and those in the zone below.219 Tropical offshore habitats also have a low concentration of nutrients due to the lack of upwelling water. Some of the nutrients such as iron are derived from aerosols especially from desert regions as shown for oligotrophic North Atlantic and Caribbean waters.220 Expected higher temperatures due to global climate change will even strengthen the pycnocline making transition in and out of the mixing layer more difficult.221 In addition, temperatures are at the upper limit for many phytoplankton species, so that further increases due to global climate change may exceed the permissive thermal window for some organisms.
As a result of low concentrations of particulate and dissolved materials in the water column, penetration of high irradiances of both PAR and UV radiation reaches levels deep down into the water column. This was confirmed by a global ocean-atmosphere model which showed that the 10% penetration at various UV wavelengths was the highest in the oceanic gyres and the lowest in optically complex continental shelf regions.222 In addition, in the tropics the solar zenith angle at noon is very low throughout the year, and thus tropical habitats have no marked seasonal changes with high PAR and UV irradiances year round.
As a consequence of high solar radiation levels, phytoplankton is under considerable radiation stress.223 Also, measurements in a tropical lagoon in New Caledonia revealed that inhibition of phyto- and bacterioplankton increases with oligotrophy and that the deepest limit of UV inhibition of phytoplankton was at about 8 m depth.224 On sunny days both small pico- and nanoplankton, but also larger microplankton, have been found to be strongly affected by solar UV radiation, mostly UV-B.225 Carbon incorporation was inhibited throughout the summer, and during the peak growth period the phytoplankton assemblages were dominated by the diatom Skeletonema, while in the rest of the time the phytoplankton concentration was low (as estimated by chl a concentrations) and the samples mainly contained monads and flagellates. In contrast, on cloudy days the microplankton (>20 μm) was found to utilize UV-A as an energy source for photosynthesis, while the pico- and nanoplankton were still inhibited by UV radiation.226 In coccolithophorids UV-B strongly inhibited the photosynthetic apparatus, while longer wavelengths impaired the calcification process.227 In Emiliania huxleyi decreased calcification resulted in a down-regulation of the photoprotective mechanism.71 Also, elevated temperatures increased photosynthesis and calcification in this organism, in contrast exposure to elevated levels of UV radiation decreased the rates of photosynthesis and calcification.227
Excessive solar radiation induces mortality in tropical marine picoplankton in oligotrophic waters.211 The cyanobacterium Synechococcus and eukaryotic phytoplankton were more resistant to solar UV and PAR than the picoplanktonic cyanobacterium Prochlorococcus. Total mortality was also significantly higher in all taxonomic groups when exposed to unfiltered solar radiation than to radiation with the UV-B component filtered out. Even short exposure of 30 min to tropical solar radiation induced mortality in Prochlorococcus. The mechanisms facilitating survival in some groups have not yet been determined.
Solar UV-B was found to affect natural phytoplankton assemblages in a subtropical marine bay in Southern China.209 When quantifying the quantum yield it was found that exposure to solar UV-B decreased growth by 17–49% under unfiltered solar radiation as compared to 11–22% reduction under radiation deprived of UV-B. Even though the phytoplankton was mixed passively in the upper mixing layer, samples collected at 4 m depth were more sensitive to solar UV-B than surface samples. Clearly, exposing phytoplankton samples at a fixed depth, e.g. when they are confined in a quartz vessel, inhibits growth and photosynthesis more than under natural conditions, when the organisms are moved within the mixing layer.228 Using simulated mixing during an experiment involving phytoplankton assemblages from the South China Sea confirmed that mixing of the cells within the water column largely reduced the UV-induced inhibition of photosynthesis which was dependent on the mixing frequency and the depth.229
Increasing temperatures due to global climate change may have additional effects on biomass production and species composition of tropical phytoplankton communities affecting higher levels in the trophic food web and increasing the vulnerability in the Barrier Reef ecosystems.230 In experiments carried out with natural assemblages isolated from a reef lagoon in the Mexican Caribbean grown in microcosms under two natural radiation conditions (unfiltered vs. only PAR radiation)231 it was found that at ambient temperature (28 °C) there was a shift from flagellates to diatoms during the 16 day incubation. At 3 °C higher temperature dinoflagellates and cryptophytes as well as the most frequent diatom Cylindrotheca decreased in density whereas small chlorophytes prospered and represented most of the biomass at the end of the exposure indicating that increasing temperatures will modify the species composition of natural phytoplankton communities exposed to natural solar radiation in tropical assemblages. There is evidence that shifts in species composition may also be induced by synergistic pollution (e.g. PAH) effects leading to higher densities of heterotrophs which will affect the carbon cycle in the ocean as well as changing the role of the ocean as a major sink for atmospheric CO2.232
Coastal phytoplankton communities
Coastal habitats are characterized by higher concentrations of organic and inorganic particulate and dissolved materials, decreasing the penetration of solar radiation into the water column (Fig. 5). Specifically the concentrations of sediments233 and UV-absorbing DOM are significantly higher,234 exposing the phytoplankton to lower levels of detrimental UV-B than in open ocean habitats at comparative water depths.235,236 Coastal phytoplankton (with the exception of Antarctic ecosystems) is commonly more sensitive to solar UV radiation than open ocean species.179 This is due to the higher DOM concentrations and lower transparency so that the cells are “dark” acclimated and need less protection mechanisms237 and, for example, they have lower MAA concentrations.238 Still the levels of natural UV-B are detrimental in many coastal areas, partly because the phytoplankton is confined to a shallower mixing layer. Comparing photosynthetic carbon fixation in coastal and offshore surface waters in the South China Sea confirmed that the corresponding levels of solar UV-B caused similar inhibition (about 28% under clear skies), but UV-A effects increased with the distance from shore, about 4% for coastal waters vs. 13% for offshore waters, respectively.239
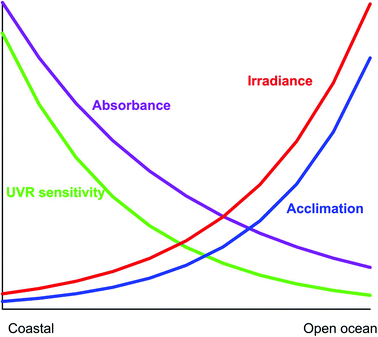 |
| Fig. 5 Gradients of key environmental factors as a function of the distance from the coast to open waters. | |
Usually coastal waters have higher nutrient concentrations due to both upwelling on the continental shelf and terrestrial runoff240 and can thus accommodate higher phytoplankton growth.241 Higher nutrient availability can also result in a different species composition from open oceanic waters as shown in an enclosure experiment with added N and P.242 High solar UV irradiances affect phosphorus cycling243 and the uptake by phytoplankton as demonstrated with 32P. Experiments carried out in a 13000-l mesocosm set up in an estuarine environment in Rhode Island showed that UV-B induced significant inhibition of phytoplankton above the thermocline but not below, indicating the significance of stratification within the water column.244 A series of mesocosm experiments at three different latitudes (Canada, Brazil and Southern Argentina) using lamp-enhanced UV-B radiation, simulating 30% or 60% ozone depletion, respectively, showed pronounced changes in species composition of both phytoplankton and grazers (ciliates).245 However, no significant decrease in algal biomass was detected which was attributed to the mixing inside the mesocosms. High nutrient concentrations in coastal waters often were responsible for blooms of toxic phytoplankton but these were found not to be affected by solar UV-B radiation in coastal waters of Japan.183
Coastal marine environments are also under the pressure of human influences.246 Pollutants such as polycyclic aromatic hydrocarbons (PAH)247 and water-soluble fractions of heavy oil also affect plankton communities.248 When added to a mesocosm, bacterial growth accelerated quickly, but decreased two days later, corresponding to an increase in small heterotrophic flagellates and viruses. The oil fraction attached to diatoms, which in turn sedimented, so that the oil pollutant was removed from the water column by vertical sedimentation.249 These aliphatic and aromatic hydrocarbons are also taken up by phytoplankton communities where they accumulate.250 Another study investigated the synergistic and non-synergistic ecotoxicological effects of solar UV-B and organic pollutants, such as atrazine, tributyltin or crude oil251 which enter coastal waters from terrestrial drainage or maritime traffic.251,252 Natural phytoplankton communities pre-stressed with UV-B were more susceptible to the pollutants than those grown under UV-free conditions. The toxicity of PAH for oceanic phytoplankton is also enhanced by solar UV as shown with natural communities from the Mediterranean Sea, Atlantic, Arctic and Southern Ocean.253 The PAH toxicity was confirmed for all phytoplankton groups, but only for the picoplankton the synergy was observed with UV radiation.
Coastal waters usually also harbor high bacterioplankton concentrations due to the availability of humic substances.254,255 This was confirmed for a coastal upwelling system, where photodegradation of residual humic substances provided nutrients for bacterioplankton growth.256 Even though they are under high pressure from UV-B and UV-A they recovered rapidly when the solar radiation decreased during the daily cycle.257–259 In an in situ analysis the highest bacterioplankton activity was found between 5 and 10 m, where short-wavelength UV-B radiation was largely attenuated.
Freshwater phytoplankton
One of the main differences between open oceans and freshwater ecosystems is the transparency to visible and UV radiation. High concentrations of DOM and particulate organic material (POM) attenuate solar radiation within the top layer of freshwater environments,260 making them similar to coastal systems. However, penetration of solar radiation also depends on the salinity of the water, i.e. UV radiation penetrates more deeply in saline prairie lakes than in freshwater habitats with the same concentration of DOC.261 Using long-term data from five North Andean Patagonian lakes, Modenutti et al.262a showed that ashes from the Puyehue volcano eruption in 2011 resulted in 1.5- to 8-fold increases in total suspended solids, light extinction and phosphorus concentrations relative to pre-eruption conditions. While the light attenuation resulted in lower light availability, this was overcompensated by enhanced growth due to the increased nutrient input. The UV transparency in lakes undergoes pronounced seasonal variations; this is due to the timing of plant litter shedding in temperate zones, and storm events such as the case of Saharan dust over high mountain lakes in Sierra Nevada.262b But even above the tree line a change in transparency was found associated with changes in phytoplankton biomass.263 While the transparency in the top 4 m of an alpine lake was fairly constant over time, it decreased in the lower zone (4–9 m depth) with time after the ice break due to increasing CDOM concentrations.264 Other external factors such as temperature, nutrients, dissolved oxygen and pH also affect the seasonality of phytoplankton growth.265 Also phytoplankton contributes to the concentration of biodegradable DOC as shown in diatom blooms in freshwater enclosures.266 The high transparency of alpine lakes together with the natural increase in solar UV-B radiation with altitude267 are the major factors affecting phytoplankton in these habitats.268 In an attempt to predict future changes in Arctic and Subarctic small lakes due to global warming, models have been developed using environmental factors such as dissolved organic carbon (DOC) concentrations, weather and water acidification affecting the transparency and thus the development of organisms.269,270
Depending on the concentration of CDOM growth inhibition of phytoplankton by solar UV-B can vary between 2.5 and 26% as shown in a study by Harrison and Smith.271 The exposure to low UV fluxes should reduce the risk of cellular damage in phytoplankton; but being adapted to lower levels of short-wavelength radiation and thus being more sensitive at least partially offset the protection by strong attenuation.7 Hessen et al.62 found an increase in phytoplankton growth in an Arctic lake when they artificially added DOC. However, this effect was soon canceled by the fast growth by more than 300% of the zooplankton predators which consisted almost exclusively of Daphnia tenebrosa. In other locations, with higher solar UV radiation, this factor may also affect the zooplankton, as shown in 53 lakes in Patagonia and Chile.272 The input of allochthonous organic carbon from terrestrial runoff increases the growth rate of bacterioplankton which mineralizes the humic substances, thus providing higher nutrient concentrations for phytoplankton which affects the biomass productivity and community structure within both the phyto- and zooplankton.273 Different taxa of phytoplankton have different sensitivities to solar UV radiation as shown in incubation experiments with species from three Arctic lakes.129 Small chlorophytes, diatoms and picocyanobacteria were more affected by UV-B than larger colony-forming cyanobacteria and chrysophytes. Therefore the latter dominated the assemblages at the end of the exposure. This was supported by the fact that the larger colony-forming species could not easily be consumed by daphnids. UV radiation can also stimulate the freshwater food webs as DOC is broken down into smaller fragments it causes an increase in bacterial abundance resulting in an increase in bacteriovore populations which positively affects the subsequent trophic levels via the microbial loop.274
In order to harvest sufficient solar energy for photosynthesis, phytoplankton moves actively or passively higher in the water column. In addition, the mixing layer tends to be thinner in freshwater habitats than in oceanic waters therefore exposing the cells to high solar radiation.275 When studying the combined impact of UV radiation, nutrient and vertical mixing over a series of lakes with different attenuations of solar radiation, Helbling et al.69 demonstrated that vertical mixing under ambient nutrient conditions produced a synergistic effect with UV radiation, increasing phytoplankton photosynthetic inhibition and excretion of organic carbon from opaque lakes as compared to algae that received constant mean irradiance within the epilimnion. In clear lakes, however, these effects were antagonistic with mixing partially counteracting the negative effects of UV radiation. Addition of nutrients, mimicking atmospheric pulses from Saharan dust, reversed this general effect, suggesting an alteration in the microbial loop and trophic interactions due to enhancement of EOC.69
Growth inhibition of phytoplankton by UV radiation is further aggravated by the lack of phosphorus supply.276 Because of differential sensitivities of species, simultaneous UV exposure and P limitation caused shifts in the species composition in boreal lake phytoplankton communities.277 Mesocosm studies in a high-altitude Spanish lake have shown that addition of P increased the fatty acid content, ω3-polyunsaturated fatty acids and the chlorophyll a to carbon as well as the C to N ratios in seston.278 UV radiation also increased the fatty acid content and ω3-polyunsaturated fatty acids, but reduced the C to P ratio in seston. These results show that the interaction between UV and P reduced the food quality of phytoplankton by reducing the content of highly unsaturated fatty acids for the next trophic level especially in oligotrophic habitats exposed to high solar UV. Exposure to solar UV-B also affects the quality of phytoplankton as food for zooplankton such as Daphnia.279 Size at maturity was lower in organisms fed on UV-B-irradiated Cryptomonas but not on Chlamydomonas. Thus exposure of phytoplankton to UV-B affects the energy transfer from the first to the subsequent levels in the food web. However, another study showed that UV radiation affects photosynthesis and the pigment composition in periphyton but not the food quality.280
Solar UV-B damages cellular DNA in freshwater phytoplankton inducing mainly CPDs and, to a smaller extent, pyrimidine (6–4) pyrimidone photoproducts (6–4 PP) and their Dewar isomers.281 Thymine dimers in three rice-field cyanobacteria (Anabaena, Nostoc and Scytonema) were quantified using blotting and the chemiluminescence method.282 The frequency of thymine dimers increased with increasing exposure time; after 120 min it reached 35–40 T^T Mbp−1 in all three studied cyanobacteria. UV-B had a much more pronounced effect than UV-A on CPD formation in Nostoc sp. In this cyanobacterium dimer formation started as early as 30 min of UV-B exposure. UV radiation also induced single- and/or double-strand breaks in various cyanobacterial species.283 Other effects of UV-B exposure are a rapid inhibition of photosynthesis, as shown in the cyanobacterium Scytonema javanicum.284 The inhibition of photosynthesis in picoplankton (0.2–2 μm) and nanoplankton (2–20 μm) by solar UV radiation was studied in an oligotrophic high mountain lake in the Swiss Alps using carbon uptake.285 The study revealed that the assemblages were most affected by UV-A. Picoplankton turned out to be more sensitive than nanoplankton. Also, Li et al.286 showed that UV-B radiation affects the phosphatase activity of the terrestrial cyanobacterium Nostoc flagelliforme. Likewise, the uptake of NO3−, NH4+, and urea is inhibited by exposure to solar UV-B.287
As do marine phytoplankton, freshwater organisms protect themselves against detrimental solar UV radiation and photo-oxidative stress by producing MAAs as well as photoprotective carotenoids.288–291 Some chlorophytes use sporopollenin or sporopollenin-like substances (algenans), biopolymers of variable composition, for screening solar UV, such as Scenedesmus communis292 and Chlamydomonas nivalis.293 These almost undegradable molecules are linked to the algal cell wall and can be found in zygospores. They absorb in the UV and blue regions of the spectrum and are responsible for the observed UV-resistance of these organisms.294–296
Conclusions
Many studies and predictions have shown that climate change-related variables have an overall common behavior that, for example, includes the increase in temperature and CO2 in the water column, the increase in solar radiation due to shallower UML, the decrease of nutrients due to isolation of the UML from deep waters with increasing stratification, or the increase of nutrients in coastal areas due to increased continental run off. In this perspective, however, we show that although the general trends are observed, the responses of aquatic primary producers have great variability in the different ecosystems. While an increase in temperature seems to be beneficial in polar waters as it enhances primary production, an indirect negative effect is the decrease of penetration of solar radiation in the water column (and thus limiting photosynthesis) due to melting of glaciers and run off of particles. Nevertheless, this melting of ice and reduction in ice thickness allow extensive blooms of phytoplankton under the ice due to more penetration of solar radiation. In contrast, increased temperature in tropical areas put more stress on cells already growing at the high limit of temperature. It also puts more strength in the picnocline thus isolating the UML even more and tending to reduce even further the potential input of nutrients from deep waters. Increasing CO2 concentrations in the water column also have contrasting effects in polar and tropical areas. In general calcified tropical organisms seem to be the most affected. In addition to these few examples, the interaction among factors changes over the aquatic systems; while an increase in temperature seems to counteract (i.e., antagonistic effect) the impact of solar UV radiation in polar and temperate systems, it reinforces it (i.e., synergistic effect) in tropical waters. Moreover, coastal areas and inland freshwater bodies are receiving increasing amounts of particulate and dissolved materials of terrestrial origin, thus changing the underwater light field, and so the exposure of cells in the water column. This latter is also conditioned by wind and depth of the UML. So, overall, we are directing towards a new “disequilibrium” and multiple factors’ interactions should be considered in order to improve our predicted general and regional models of how primary producers would respond to global change and how this would affect the whole aquatic food web.
Acknowledgements
We thank for the comments and suggestions of two anonymous reviewers that helped in improving our manuscript. This work was supported by Consejo Nacional de Investigaciones Científicas y Técnicas – CONICET (PIP 11220100100228), Agencia Nacional de Promoción Científica y Tecnológica – ANPCyT (PICT 2012-0271) and Fundación Playa Unión. This is contribution no. 140 of Estación de Fotobiología Playa Unión.
References
- M. A. Charette and W. H. F. Smith, The Volume of Earth's Ocean, Oceanography, 2010, 23, 112–114 CrossRef.
- D.-P. Häder, H. D. Kumar, R. C. Smith and R. C. Worrest, Effects on aquatic ecosystems, J. Photochem. Photobiol., B, 1998, 46, 53–68 CrossRef.
- H. K. Lotze, B. Worm, M. Molis and M. Wahl, Effects of UV radiation and consumers on recruitment and succession of a marine macrobenthic community, Mar. Ecol. Prog. Ser., 2002, 243, 57–66 CrossRef.
-
FAO, The state of world fisheries and aquaculture 2012, FAO, Rome, 2012 Search PubMed.
-
H. Cesar, L. Burke and L. Pet-Soede, The Economics of Worldwide Coral Reef Degradation, WWF and ICRAN, 2003 Search PubMed.
- A. V. Parisi and M. G. Kimlin, Personal solar UV exposure measurements employing modified polysulphone with an extended dynamic range, Photochem. Photobiol., 2004, 79, 411–415 CrossRef CAS.
- D.-P. Häder, H. D. Kumar, R. C. Smith and R. C. Worrest, Effects of solar UV radiation on aquatic ecosystems and interactions with climate change, Photochem. Photobiol. Sci., 2007, 6, 267–285 Search PubMed.
- M. M. Ali, A. A. Mageed and M. Heikal, Importance of aquatic macrophyte for invertebrate diversity in large subtropical reservoir, Limnologica – Ecol. Manage. Inland Waters, 2007, 37, 155–169 CrossRef PubMed.
-
P. Tardent, Meeresbiologie, Eine Einführung, Thieme, Stuttgart, 2005 Search PubMed.
- S. W. Wilhelm and A. R. Matteson, Freshwater and marine virioplankton: a brief overview of commonalities and differences, Freshwater Biol., 2008, 53, 1076–1089 CrossRef PubMed.
- A. S. Lang, M. L. Rise, A. I. Culley and G. F. Steward, RNA viruses in the sea, FEMS Microbiol. Rev., 2009, 33, 295–233 CrossRef CAS PubMed.
- C. P. D. Brussaard, Viral control of phytoplankton populations - a review, J. Eukaryot. Microbiol., 2004, 51, 125–138 CrossRef PubMed.
- F. Azam, Microbial control of oceanic carbon flux: The plot thickens, Science, 1998, 280, 694–696 CrossRef CAS.
-
(a)
R. Chester and T. Jickells, Marine Geochemistry, Wiley–Blackwell, Chichester, 2012 Search PubMed;
(b)
IPCC, Summary for Policymakers: Climate change 2013 – The physical science basis, Working Group 1 Contribution to the IPCC Fifth Assessment Report, 2013, pp. 1–38.
- P. Falkowski, R. J. Scholes, E. Boyle, J. Canadell, D. Canfield, J. Elser, N. Gruber, K. Hibbard, P. Högberg, S. Linder, F. T. Mackenzie, B. Moore Iii, T. Pedersen, Y. Rosenthal, S. Seitzinger, V. Smetacek and W. Steffen, The global carbon cycle: a test of our knowledge of earth as a system, Science, 2000, 290, 291–296 CrossRef CAS.
-
J. E. Girard, Principles of Environmental Chemistry, Jones & Bartlett Learning, Burlington, MA, 2013 Search PubMed.
- M. Hein and K. Sand-Jensen, CO2 increases oceanic primary production, Nature, 1997, 388, 526–527 CrossRef CAS PubMed.
- P. Schippers, M. Lürling and M. Scheffer, Increase of atmospheric CO2 promotes phytoplankton productivity, Ecol. Lett., 2004, 7, 446–451 CrossRef PubMed.
- O. Hoegh-Guldberg, Dangerous shifts in ocean ecosystem function?, ISME J., 2010, 1090–1092 CrossRef PubMed.
- R. E. Zeebe, J. C. Zachos, K. Caldeira and T. Tyrrell, Oceans - Carbon emissions and acidification, Science, 2008, 321, 51–52 CrossRef CAS PubMed.
- R. Terrado, K. Scarcella, M. Thaler, W. F. Vincent and C. Lovejoy, Small phytoplankton in Arctic seas: vulnerability to climate change, Biodivers. Conserv., 2013, 14, 2–18 Search PubMed.
- K. Gao, E. W. Helbling, D.-P. Häder and D. A. Hutchins, Responses of marine primary producers to interactions between ocean acidification, solar radiation, and warming, Mar. Ecol. Prog. Ser., 2012, 470, 167–189 CrossRef CAS.
- K. R. N. Anthony, J. A. Maynard, G. Diaz-Pulido, P. J. Mumby, P. A. Marshall, L. Cao and O. Hoegh-Guldenberg, Ocean acidification and warming will lower coral reef resilience, GCB, 2011, 17, 1798–1808 CrossRef PubMed.
- V. J. Fabry, B. A. Seibel, R. A. Feely and J. C. Orr, Impacts of ocean acidification on marine fauna and ecosystem processes, ICES J. Mar. Sci., 2008, 65, 414–432 CrossRef CAS.
- L. Beaufort, I. Probert, T. De Garidel-Thoron, E. M. Bendif, D. Ruiz-Pino, N. Metzl, C. Goyet, N. Buchet, P. Coupel, M. Grelaud, B. Rost, R. E. M. Rickaby and C. de Vargas, Sensitivity of coccolithophores to carbonate chemistry and ocean acidification, Nature, 2011, 476, 80–83 CrossRef CAS PubMed.
- S. C. Doney, V. J. Fabry, R. A. Feely and J. A. Kleypas, Ocean acidification: the other CO2 problem, Annu. Rev. Mar. Sci., 2009, 1, 169–192 CrossRef PubMed.
- P. Coupel, H. Y. Jin, M. Joo, R. Horner, H. A. Bouvet, V. Garçon, M.-A. Sicre, J.-C. Gascard, J. F. Chen and D. Ruiz-Pino, Phytoplankton distribution in unusually low sea ice cover over the Pacific Arctic, Biogeosciences, 2012, 9, 4835–4850 CAS.
- J. Marra, V. P. Lance, R. D. Vaillancourt and B. R. Hargreaves, Resolving the ocean's euphotic zone, Deep Sea Res. Pt. I, 2014, 83, 45–50 CrossRef PubMed.
- D.-P. Häder, Penetration and effects of solar UV-B on phytoplankton and macroalgae, Plant Ecol., 1997, 128, 4–13 Search PubMed.
-
D.-P. Häder, Influence of ultraviolet radiation on phytoplankton ecosystems, in Algae, Environment and Human Affairs, ed. W. Wiessner, E. Schnepf and R. C. Starr, Biopress Limited, Bristol, England, 1995, pp. 41–55 Search PubMed.
- B. A. Bancroft, N. J. Baker and A. R. Blaustein, Effects of UVB radiation on marine and freshwater organisms: a synthesis through meta-analysis, Ecol. Lett., 2007, 10, 332–345 CrossRef PubMed.
-
V. E. Villafañe, K. Sundbäck, F. L. Figueroa and E. W. Helbling, Photosynthesis in the aquatic environment as affected by UVR, in UV effects in aquatic organisms and ecosystems, ed. E. W. Helbling and H. E. Zagarese, Royal Society of Chemistry, London, 2003, pp. 357–397 Search PubMed.
- B. Olesen and S. C. Maberly, The effect of high levels of visible and ultra-violet radiation on the photosynthesis of phytoplankton from a freshwater lake, Arch. Hydrobiol., 2001, 151, 301–315 CAS.
- D.-P. Häder, H. D. Kumar, R. C. Smith and R. C. Worrest, Aquatic ecosystems: effects of solar ultraviolet radiation and interactions with other climatic change factors, Photochem. Photobiol. Sci., 2003, 2, 39–50 Search PubMed.
- M. D. Lamare, M. F. Barker, M. P. Lesser and C. Marshall, DNA photorepair in echinoid embryos: effects of temperature on repair rate in Antarctic and non-Antarctic species, J. Exp. Biol., 2006, 209, 5017–5028 CrossRef CAS PubMed.
- C. Wong, W. Chu, H. Marchant and S. Phang, Comparing the response of Antarctic, tropical and temperate microalgae to ultraviolet radiation (UVR) stress, J. Appl. Phycol., 2007, 19, 689–699 CrossRef.
- X. Yuan, K. Yin, P. J. Harrison and J. Zhang, Phytoplankton are more tolerant to UV than bacteria and viruses in the northern South China Sea, Aquat. Microb. Ecol., 2011, 65, 117–128 CrossRef.
- Y. Bettarel, T. Bouvier and M. Bouvy, Viral persistence in water as evaluated from a tropical/temperate cross-incubation, J. Plankton Res., 2009, 31, 909–916 CrossRef PubMed.
- S. W. Wilhelm, W. H. Jeffrey, A. L. Dean, J. Meador, J. D. Pakulski and D. L. Mitchell, UV radiation induced DNA damage in marine viruses along a latitudinal gradient in the southeastern Pacific Ocean, Aquat. Microb. Ecol., 2003, 31, 1–8 CrossRef.
- S. Jacquet and G. Bratbak, Effects of ultraviolet radiation on marine virus-phytoplankton interactions, FEMS Microbiol. Ecol., 2003, 44, 279–289 CrossRef CAS.
- J. M. Manrique, A. Y. Calvo, S. R. Halac, V. E. Villafañe, L. R. Jones and E. W. Helbling, Effects of UV radiation on the taxonomic composition of natural bacterioplankton communities from Bahía Engaño (Patagonia, Argentina), J. Photochem. Photobiol., B, 2012, 117, 171–178 CrossRef CAS PubMed.
- K. Whitehead, D. Karentz and J. I. Hedges, Mycosporine-like amino acids (MAAs) in phytoplankton, a herbivorous pteropod (Limacina helicina), and its pteropod predator (Clione antarctica) in McMurdo Bay, Antarctica, Mar. Biol., 2001, 139, 1013–1019 CrossRef CAS.
- A. Oren and N. Gunde-Cimerman, Mycosporines and mycosporine-like amino acids: UV protectants or multipurpose secondary metabolites?, FEMS Microbiol. Lett., 2007, 269, 1–10 CrossRef CAS PubMed.
- P. J. Janknegt, C. M. De Graaff, W. H. Van De Poll, R. J. Visser, E. W. Helbling and A. G. Buma, Antioxidative responses of two marine microalgae during acclimation to static and fluctuating natural UV radiation, Photochem. Photobiol., 2009, 85, 1336–1345 CrossRef CAS PubMed.
- P. J. Janknegt, J. W. Rijstenbil, W. H. Van de Poll, T. S. Gechev and A. G. Buma, A comparison of quantitative and qualitative superoxide dismutase assays for application to low temperature microalgae, J. Photochem. Photobiol., B, 2007, 87, 218–226 CrossRef CAS PubMed.
- V. E. Villafañe, K. Gao, P. Li and E. W. Helbling, Vertical mixing within the epilimnion modulates UVR-induced photoinhibition in tropical freshwater phytoplankton from southern China, Freshwater Biol., 2007, 52, 1260–1270 CrossRef PubMed.
- Z. Cai, S. Duan and W. Wei, Darkness and UV radiation provoked compensatory growth in marine phytoplankton Phaeodactylum tricornutum (Bacillariophyceae), Aquacult. Res., 2009, 40, 1559–1562 CrossRef PubMed.
- C. Belzile, S. Demers, G. A. Ferreyra, I. Schloss, C. Nozais, K. Lacoste, B. Mostajir, S. Roy, M. Gosselin and E. Pelletier, UV effects on marine planktonic food webs: A synthesis of results from mesocosm studies, Photochem. Photobiol., 2006, 82, 850–856 CrossRef CAS PubMed.
- J. A. Raven, Physiology of inorganic C acquisition and implications for resource use efficiency by marine phytoplankton: relation to increased CO2 and temperature, Plant Cell Environ., 1991, 14, 779–794 CrossRef CAS PubMed.
- A. Neori and O. Holm-Hansen, Effect of temperature on rate of photosynthesis in Antarctic phytoplankton, Polar Biol., 1982, 1, 33–38 CrossRef CAS.
- I. E. Huertas, M. Rouco, V. López-Rodas and E. Costas, Warming will affect phytoplankton differently: evidence through a mechanistic approach, Proc. R. Soc. London, B, 2011, 278, 3534–3543 CrossRef PubMed.
- L. J. Falkenberg, B. D. Russell and S. D. Connell, Contrasting resource limitations of marine primary producers: Implications for competitive interactions under enriched CO2 and nutrient regimes, Oecologia, 2013, 172, 575–583 CrossRef PubMed.
- G. M. Hallegraeff, Ocean climate change, phytoplankton community responses, and harmful algal blooms: a formidable predictive challenge, J. Phycol., 2010, 46, 220–235 CrossRef CAS PubMed.
- D. Bronk, J. See, P. Bradley and L. Killberg, DON as a source of bioavailable nitrogen for phytoplankton, Biogeosciences, 2007, 4, 283–296 CrossRef CAS PubMed.
- A. Paytan, G. G. Shellenbarger, J. H. Street, M. E. Gonneea, K. Davis and M. B. Young, Submarine groundwater discharge: An important source of new inorganic nitrogen to coral reef ecosystems, Limnol. Oceanogr., 2006, 51, 343–348 CrossRef CAS.
- H. Harada, M. Vila-Costa, J. Cebrian and R. P. Kiene, Effects of UV radiation and nitrate limitation on the production of biogenic sulfur compounds by marine phytoplankton, Aquat. Bot., 2009, 90, 37–42 CrossRef CAS PubMed.
- C. Labry, D. Delmas and A. Herbland, Phytoplankton and bacterial alkaline phosphatase activities in relation to phosphate and DOP availability within the Gironde plume waters (Bay of Biscay), J. Exp. Mar. Biol. Ecol., 2005, 318, 213–225 CrossRef CAS PubMed.
- F. Roncarati, J. W. Rijstenbil and R. Pistocchi, Photosynthetic performance, oxidative damage and antioxidants in Cylindrotheca closterium in response to high irradiance, UVB radiation and salinity, Mar. Biol., 2008, 153, 965–973 CrossRef CAS.
- U. Riebesell, K. G. Schulz, R. G. J. Bellerby, M. Botros, P. Fritsche, M. Meyerhöfer, C. Neill, G. Nondal, A. Oschlies, J. Wohlers and E. Zöllner, Enhanced biological carbon consumption in a high CO2 ocean, Nature, 2007, 450, 545–548 CrossRef CAS PubMed.
-
P. G. Falkowski and J. A. Raven, Aquatic Photosynthesis, Blackwell Science, Massachusetts, USA, 1997 Search PubMed.
-
J. Passarge, J. Huisman, U. Sommer and B. Worm, Competition in well-mixed habitats: From competitive exclusion to competitive chaos, in Competition and Coexistence, Springer-Verlag, Berlin, 2002, pp. 7–42 Search PubMed.
- D. O. Hessen, P. Blomqvist, G. Dahl-Hansen, S. Drakare and E. S. Lindström, Production and food web interactions of Arctic freshwater plankton and responses to increased DOC, Arch. Hydrobiol., 2004, 159, 289–307 CrossRef.
- D. W. Schindler and J. P. Smol, Cumulative effects of climate warming and other human activities on freshwaters of Arctic and Subarctic North America, Ambio, 2006, 35, 160–168 CrossRef.
- G. A. Ferreyra, B. Mostajir, I. R. Schloss, K. Chatila, M. E. Ferrario, P. Sargian, S. Roy, J. Prod'homme and S. Demers, Ultraviolet-B radiation effects on the structure and function of lower trophic levels of the marine planktonic food web, Photochem. Photobiol., 2006, 82, 887–897 CrossRef CAS.
- K. Gao, J. Xu, G. Gao, Y. Li, D. A. Hutchins, B. Huang, Y. Zheng, P. Jin, X. Cai, D.-P. Häder, W. Li, K. Xu, N. Liu and U. Riebesell, Rising carbon dioxide and increasing light exposure act synergistically to reduce marine primary productivity, Nat. Clim. Change, 2012, 2, 519–523 CAS.
- B. D. Russell, C. A. Passarelli and S. D. Connell, Forecasted CO2 modifies the influence of light in shaping subtidal habitat, J. Phycol., 2011, 47, 744–752 CrossRef PubMed.
- P. W. Boyd, Beyond ocean acidification, Nat. Geosci., 2011, 4, 273–274 CrossRef CAS.
- D.-P. Häder, E. W. Helbling, C. E. Williamson and R. C. Worrest, Effects of UV radiation on aquatic ecosystems and interactions with climate change, Photochem. Photobiol. Sci., 2011, 10, 242–260 Search PubMed.
- E. W. Helbling, P. Carrillo, J. M. Medina-Sánchez, C. Durán, G. Herrera, M. Villar-Argaiz and V. E. Villafañe, Interactive effects of vertical mixing, nutrients and ultraviolet radiation: in situ photosynthetic responses of phytoplankton from high mountain lakes in Southern Europe, Biogeosciences, 2013, 10, 1037–1050 Search PubMed.
- G. Malanga and S. Puntarulo, Oxidative stress and antioxidant content in Chlorella vulgaris after exposure to ultraviolet-B radiation, Physiol. Plant., 1995, 94, 672–679 CrossRef CAS PubMed.
- K. Xu, K. Gao, V. Villafañe and E. Helbling, Photosynthetic responses of Emiliania huxleyi to UV radiation and elevated temperature: roles of calcified coccoliths, Biogeosciences, 2011, 8, 1441–1452 CrossRef CAS.
- J. E. Cloern, S. Q. Foster and A. E. Fleckner, Review: phytoplankton primary production in the world's estuarine-coastal ecosystems, Biogeosci. Discuss., 2014, 10, 17725–17783 Search PubMed.
- G. C. Hays, A. J. Richardson and C. Robinson, Climate change and marine plankton, Trends Ecol. Evol., 2005, 20, 337–344 CrossRef PubMed.
- M. Rajadurai, E. Poornima, S. Narasimhan, V. Rao and V. Venugopalan, Phytoplankton growth under temperature stress: laboratory studies using two diatoms from a tropical coastal power station site, J. Therm. Biol., 2005, 30, 299–305 CrossRef PubMed.
-
P. W. Boyd and S. C. Doney, The impact of climate change and feedback processes on the ocean carbon cycle, in Ocean Biogeochemistry, ed. M. J. R. Fasham, Springer, 2003, pp. 157–193 Search PubMed.
- G. Li, Y. Wu and K. Gao, Effects of Typhoon Kaemi on coastal phytoplankton assemblages in the South China Sea, with special reference to the effects of solar UV radiation, J. Geophys. Res.: Biogeosci., 2009, 114, G04029, DOI:10.1029/2008JG000896.
- S. Ringuet and F. T. Mackenzie, Controls on nutrient and phytoplankton dynamics during normal flow and storm runoff conditions, southern Kaneohe Bay, Hawaii, Estuaries, 2005, 28, 327–337 CrossRef CAS.
- C. D. Harley, A. Randall Hughes, K. M. Hultgren, B. G. Miner, C. J. Sorte, C. S. Thornber, L. F. Rodriguez, L. Tomanek and S. L. Williams, The impacts of climate change in coastal marine systems, Ecol. Lett., 2006, 9, 228–241 CrossRef PubMed.
- B. M. Clark, Climate change: A looming challenge for fisheries management in southern Africa, Mar. Policy, 2006, 30, 84–95 CrossRef PubMed.
- J. Beardall, C. Sobrino and S. Stojkovic, Interactions between the impacts of ultraviolet radiation, elevated CO2, and nutrient limitation on marine primary producers, Photochem. Photobiol. Sci., 2009, 8, 1257–1265 CAS.
- J. Beardall, S. Stojkovic and S. Larsen, Living in a high CO2 world: impacts of global climate change on marine phytoplankton, Plant Ecol. Divers., 2009, 2, 191–205 CrossRef.
- Z. V. Finkel, J. Beardall, K. J. Flynn, A. Quigg, T. A. V. Rees and J. A. Raven, Phytoplankton in a changing world: cell size and elemental stoichiometry, J. Plankton Res., 2010, 32, 119–137 CrossRef CAS PubMed.
- C. E. Williamson, C. Salm, S. L. Cooke and J. E. Saros, How do UV radiation, temperature, and zooplankton influence the dynamics of alpine phytoplankton communities?, Hydrobiologia, 2010, 648, 73–81 CrossRef.
- C. Ruiz-González, M. Galí, E. Sintes, G. J. Herndl, J. M. Gasol and R. Simó, Sunlight effects on the osmotrophic uptake of DMSP-sulfur and leucine by Polar phytoplankton, PLoS One, 2012, 7 DOI:10.1371/journal.pone.0045545.
- W. Sunda, D. J. Kieber, R. P. Kiene and S. Huntsman, An antioxidant function for DMSP and DMS in marine algae, Nature, 2002, 418, 317–320 CrossRef CAS PubMed.
- U. Karsten, C. Wiencke and G. O. Kirst, The effect of light intensity and daylength on the β-dimethylsulphoniopropionate (DMSP) content of marine green macroalgae from Antarctica, Plant, Cell Environ., 1990, 13, 989–993 CrossRef CAS PubMed.
- A. Merzouk, M. Levasseur, M. Scarratt, S. Michaud and M. Gosselin, Influence of dinoflagellate diurnal vertical migrations on dimethylsulfoniopropionate and dimethylsulfide distribution and dynamics (St. Lawrence Estuary, Canada), Can. J. Fish. Aquat. Sci., 2004, 61, 712–720 CrossRef CAS.
- F. S. E. Buckley and S. M. Mudge, Dimethylsulphide and ocean-atmosphere interactions, Chem. Ecol., 2004, 20, 73–95 CrossRef CAS.
- W. G. Sunda and D. R. Hardison, Contrasting seasonal patterns in dimethylsulfide, dimethylsulfoniopropionate, and chlorophyll a in a shallow North Carolina estuary and the Sargasso Sea, Aquat. Microb. Ecol., 2008, 53, 281–294 CrossRef.
- A. J. Trevena and G. B. Jones, Dimethylsulphide and dimethylsulphoniopropionate in Antarctic sea ice and their release during sea ice melting, Mar. Chem., 2006, 98, 210–222 CrossRef CAS PubMed.
- W. G. Sunda, R. Hardison, R. P. Kiene, E. Bucciarelli and H. Harada, The effect of nitrogen limitation on cellular DMSP and DMS release in marine phytoplankton: climate feedback implications, Aquat. Sci., 2007, 69, 341–351 CrossRef CAS.
-
M. B. Usher, Principles of Conserving the Arctic's Biodiversity, in Arctic Climate Impact Assessment, ed. C. Symon, L. Arris and B. Heal, Cambridge University Press, New York, 2005 Search PubMed.
- C. Deser, J. E. Walsh and M. S. Timlin, Arctic sea ice variability in the context of recent atmospheric circulation trends, J. Clim., 2000, 13, 617–633 CrossRef.
- J. C. Comiso, C. L. Parkinson, R. Gersten and L. Stock, Accelerated decline in the Arctic sea ice cover, Geophys. Res. Lett., 2008, 35, L01703, DOI:10.1029/2007GL031972.
-
http://nsidc.org, http://nsidc.org/arcticseaicenews/2012/09/arctic-sea-ice-extent-settles-at-record-seasonal-minimum/.
-
http://earth.columbia.edu, http://earth.columbia.edu/articles/view/2993.
- D. A. Rothrock, Y. Yu and G. A. Maykut, Thinning of the Arctic sea-ice cover, Geophys. Res. Lett., 1999, 26, 3469–3472 CrossRef.
- M. Fischetti, Deep heat threatens marine life, Sci. Am., 2013, 2013, 72 Search PubMed.
- J. A. Screen and I. Simmonds, The central role of diminishing sea ice in recent Arctic temperature amplification, Nature, 2010, 464, 1334–1337 CrossRef CAS PubMed.
- J. F. Scinocca, M. C. Reader, D. A. Plummer, M. Sigmond, P. J. Kushner, T. G. Shepherd and A. R. Ravishankara, Impact of sudden Arctic sea-ice loss on stratospheric polar ozone recovery, Geophys. Res. Lett., 2009, 36, L24701, DOI:10.1029/2009GL041239.
- B. Light, T. C. Grenfell and D. K. Perovich, Transmission and absorption of solar radiation by Arctic sea ice during the melt season, J. Geophys. Res., 2008, 113, C03023, DOI:10.1029/2006JC003977.
- O. R. Anderson, Modern incursions of tropical Radiolaria into the Arctic Ocean, J. Micropalaeontol., 2012, 31, 139–158 CrossRef PubMed.
-
W. Parry, Arctic's Spring Phytoplankton Blooms Arrive Earlier, http://www.livescience.com/13082-arctic-plankton-blooms-ocean-climate-change.html.
- M. Kahru, V. Brotas, B. Manzano-Sarabia and B. G. Mitchell, Are phytoplankton blooms occurring earlier in the Arctic?, GCB, 2010, 17, 1733–1739 CrossRef PubMed.
- Economist, http://www.economist.com/news/science-and-technology/21571386.
-
M. Perrette, G. Quartly, E. E. Popova and A. Yool, in ESA Living Planet Symposium, European Space Agency (ESA Publication, SP-686), Bergen, 2010 Search PubMed.
- K. R. Arrigo and G. L. van Dijken, Secular trends in Arctic Ocean net primary production, J. Geophys. Res.: Oceans (1978–2012), 2011, 116, 160–168 Search PubMed.
- Z. Yanpei, J. Haiyan, C. Jianfang, W. Bin, L. Hongliang, C. Fajin, L. Yong and X. Jie, Nutrient status and phytoplankton-pigments response to ice melting in the Arctic Ocean, Adv. Polar Sci., 2012, 24, 151–158 Search PubMed.
- V. Alexander and H. J. Niebauer, Oceanography of the Eastern Bering Sea ice-edge zone in spring, Limnol. Oceanogr., 1981, 26, 1111–1125 CrossRef.
- V. Hill, G. F. Cota and D. Stockwell, Spring and summer phytoplankton communities in the Chukchi and Eastern Beaufort Seas, Deep Sea Res., Part II, 2005, 52, 3369–3385 CrossRef PubMed.
- A. Luchetta, M. Lipizer and G. Socal, Temporal evolution of primary production in the central Barents Sea, J. Mar. Syst., 2000, 27, 177–193 CrossRef.
- M. Perrette, A. Yool, G. D. Quartly and E. E. Popova, Near-ubiquity of ice-edge blooms in the Arctic, Biogeosciences, 2011, 8, 515–524 CrossRef.
- P. G. Falkowski and M. J. Oliver, Mix and match: how climate selects phytoplankton, Nature, 2007, 5, 813–819 CAS.
- L. N. M. Duysens, Energy transformations in photosynthesis, Ann. Rev. Plant Physiol., 1956, 7, 25–50 CrossRef CAS.
- A. Matsuoka, P. Larouche, M. Poulin, W. Vincent and H. Hattori, Phytoplankton community adaptation to changing light levels in the southern Beaufort Sea, Canadian Arctic, Estuarine, Coastal Shelf Sci., 2009, 82, 537–546 CrossRef CAS PubMed.
- L. Morello and ClimateWire, Thinning Arctic Ice Allows Plankton Bloom, Sci. Am., 2012, June, 289–307 Search PubMed.
- K. R. Arrigo, D. K. Perovich, R. S. Pickart, Z. W. Brown, G. L. van Dijken, K. E. Lowry, M. M. Mills, M. A. Palmer, W. M. Balch, F. Bahr, N. R. Bates, C. Benitez-Nelson, B. Bowler, E. Brownlee, J. K. Ehn, K. E. Frey, R. Garley, S. R. Laney, L. Lubelczyk, J. Mathis, A. Matsuoka, B. G. Mitchell, G. W. K. Moore, E. Ortega-Retuerta, S. Pal, C. M. Polashenski, R. A. Reynolds, B. Schieber, H. M. Sosik, M. Stephens and J. H. Swift, Massive phytoplankton blooms under Arctic sea ice, Science, 2012, 336, 1408 CrossRef CAS PubMed.
-
(a)
R. Lindsey, Melt pond “skylights” enable massive under-ice bloom in Arctic, http://www.climatewatch.noaa.gov/article/2012/melt-pond-skylights-enable-massive-under-ice-bloom-in-arctic;
(b) D. K. Perovich, A theoretical model of ultraviolet light transmission through Antarctic sea ice, J. Geophys. Res., 1993, 98,22,579-22, 587 Search PubMed.
- K. E. Frey, D. K. Perovich and B. Light, The spatial distribution of solar radiation under a melting Arctic sea ice cover, Geophys. Res. Lett., 2011, 38, L22501 CrossRef.
-
E. C. Carmack, The Arctic Ocean's freshwater budget: Sources, storage, and export, in The Freshwater Budget of the Arctic Ocean, ed. E. L. Lewis, Kluwer Acad., Norwell, Mass., 2000, pp. 91–126 Search PubMed.
- R. G. M. Spencer, G. R. Aiken, K. D. Butler, M. M. Dornblaser, R. G. Striegl and P. J. Hernes, Utilizing chromophoric dissolved organic matter measurements to derive export and reactivity of dissolved organic carbon exported to the Arctic Ocean: A case study of the Yukon River, Alaska, Geophys. Res. Lett., 2009, 36, L06401, DOI:10.1029/2008GL036831.
- C. U. I. Shikai, H. E. Jianfeng, H. E. Peimin, Z. Fang, L. Ling and M. A. Yuxin, The adaptation of Arctic phytoplankton to low light and salinity in Kongsfjorden (Spitsbergen), Adv. Polar Sci., 2012, 23, 19–24 Search PubMed.
- M. Steinacher, F. Joos, T. L. Froelicher, L. Bopp, P. Cadule, V. Cocco, S. C. Doney, M. Gehlen, K. Lindsay, J. K. Moore, B. Schneider and J. Segschneider, Projected 21st century decrease in marine productivity: a multi-model analysis, Biogeosciences, 2010, 7, 979–1005 CrossRef CAS.
- M.-Y. Sun, L. M. Clough, M. L. Carroll, J. Dai, W. G. Ambrose Jr. and G. R. Lopez, Different responses of two common Arctic macrobenthic species (Macoma balthica and Monoporeia affinis) to phytoplankton and ice algae: Will climate change impacts be species specific?, J. Exp. Mar. Biol. Ecol., 2009, 376, 110–121 CrossRef PubMed.
- S. Bélanger, M. Babin and J.-E. Tremblay, Increasing cloudiness in Arctic damps the increase in phytoplankton primary production due to sea ice receding, Biogeosciences, 2013, 10, 4087–4101 Search PubMed.
- S. Rysgaard, T. G. Nielsen and B. W. Hansen, Seasonal variation in nutrients, pelagic primary production and grazing in a high-Arctic coastal marine ecosystem, Young Sound, Northeast Greenland, Mar. Ecol. Prog. Ser., 1999, 179, 13–25 CrossRef CAS.
- A. Calbet, K. Riisgaard, E. Saiz, S. Zamora, C. A. Stedmon and T. Gissel Nielsen, Phytoplankton growth and microzooplankton grazing along a sub-Arctic fjord (Godthåbsfjord, west Greenland), Mar. Ecol. Prog. Ser., 2011, 442, 11–22 CrossRef CAS.
- S. A. Wängberg, K. I. M. Andreasson, K. Gustavson, T. Reinthaler and P. Henriksen, UV-B effects on microplankton communities in Kongsfjord, Svalbard - A mesocosm experiment, J. Exp. Mar. Biol. Ecol., 2008, 365, 156–163 CrossRef PubMed.
-
E. Van Donk, B. A. Faafeng, H. J. De Lange and D. O. Hessen, Differential sensitivity to natural ultraviolet radiation among phytoplankton species in Arctic lakes (Spitsbergen, Norway), in Plant Ecology. Special Issue: Responses of Plants to UV-B Radiation, ed. J. Rozema, Y. Manetas and L. O. Björn, Kluwer Academic Publishers, Dordrecht, Boston, London, 2001, pp. 249–259 Search PubMed.
- D. G. Petersen and I. Dahllöf, Combined effects of pyrene and UV-light on algae and bacteria in an Arctic sediment, Ecotoxicology, 2007, 16, 371–377 CrossRef CAS PubMed.
-
C. J. Galbán-Malagón, A. Cabrerizo, N. Berrojálbiz, M. J. Ojeda and J. Dachs, Air-water exchange and phytoplankton accumulation of persistent organic pollutants in the Greenland current and Arctic Ocean, http://132.246.11.198/2012-ipy/pdf-all/ipy2012arAbstract00801.pdf.
- B. G. Mitchell, E. A. Brody, O. Holm-Hansen, C. R. McClain and J. Bishop, Light limitation of phytoplankton biomass and macronutrient utilization in the Southern Ocean, Limnol. Oceanogr., 1991, 36(8), 1662–1677 CrossRef.
- M. H. Taylor, M. Losch and A. Bracher, On the drivers of phytoplankton blooms in the Antarctic marginal ice zone: a modeling approach, J. Geophys. Res., 2013, 118, 63–75 Search PubMed.
- J. H. Martin, R. M. Gordon and S. E. Fitzwater, Iron in Antarctic waters, Nature, 1990, 345, 156–158 CrossRef CAS.
- U. Sommer, Nitrate- and silicate-competition among antarctic phytoplankton, Mar. Biol., 1986, 91, 345–351 CrossRef CAS.
- J. M. Rose, Y. Feng, G. R. DiTullio, R. B. Dunbar, C. E. Hare, P. A. Lee, M. Lohan, M. Long, W. O. Smith Jr., B. Sohst, S. Tozzi, Y. Zhang and D. A. Hutchins, Synergistic effects of iron and temperature on Antarctic phytoplankton and microzooplankton assemblages, Biogeosciences, 2009, 6, 3131–3147 CrossRef CAS.
- B. Dold, E. Gonzalez-Toril, A. Aguilera, E. Lopez-Pamo, M. E. Cisternas, F. Bucchi and R. Amils, Acid rock drainage and rock weathering in Antarctica: Important sources for iron cycling in the Southern Ocean, Environ. Sci. Technol., 2013, 47, 6129–6136 CAS.
- P. N. Sedwick and G. R. DiTullio, Regulation of algal blooms in Antarctic Shelf Waters by the release of iron from melting sea ice, Geophys. Res. Lett., 1997, 24, 2515–2518 CrossRef CAS.
- A. Agustí and C. M. Duarte, Experimental induction of a large phytoplankton bloom in Antarctic coastal waters, Mar. Ecol. Prog. Ser., 2000, 206, 73–85 CrossRef.
- E. D. Ingall, J. M. Diaz, A. F. Longo, M. Oakes, L. Finney, S. Vogt, B. Lai, P. L. Yager, B. S. Twining and J. A. Brandes, Role of biogenic silica in the removal of iron from the Antarctic seas, Nat. Commun., 2013 DOI:10.1038/ncomms2981.
- A. McMinn, N. Bleakley, K. Steinberner, D. Roberts and L. J. Trenerry, Effect of permanent sea ice cover and different nutrient regimes on the phytoplankton succession of fjords of the Vestfold Hills Oasis, eastern Antarctica, J. Plankton Res., 2000, 22, 287–303 CrossRef CAS PubMed.
- K. R. Arrigo and G. L. van Dijken, Phytoplankton dynamics within 37 Antarctic coastal polynya systems, J. Geophys. Res., 2003, 108, 3271 CrossRef.
- C. Lancelot, S. Mathot, V. Veth and H. de Baar, Factors controlling phytoplankton ice-edge blooms in the marginal ice-zone of the northwestern Weddell Sea during sea ice retreat 1988: Field observations and mathematical modelling, Polar Biol., 1993, 13, 377–387 CrossRef.
- S.-H. Kang, J.-S. Kang, S. Lee, K. H. Chung, D. Kim and M. G. Park, Antarctic phytoplankton assemblages in the marginal ice zone of the Northwestern Weddell Sea, J. Plankton Res., 2001, 23, 333–352 CrossRef CAS PubMed.
- K. M. Swadling, J. A. E. Gibson, D. A. Ritz, P. D. Nicols and D. E. Hughes, Grazing of phytoplankton by copepods in eastern Antarctic coastal waters, Mar. Biol., 1997, 128, 39–48 CrossRef.
- A. Atkinson, B. Meyer, D. Stübing, W. Hagen, K. Schmidt and U. V. Bathmann, Feeding and energy budgets of Antarctic krill Euphausia superba at the onset of winter - II. Juveniles and adults, Limnol. Oceanogr., 2002, 47, 953–966 CrossRef.
- K. Schmidt, A. Atkinson, D. Stübing, J. W. McClelland, J. P. Montoya and M. Voss, Trophic relationships among Southern Ocean copepods and krill: some uses and limitations of a stable isotope approach, Limnol. Oceanogr., 2003, 48, 277–289 CrossRef.
- A. Tanimura, S. Kawaguchi, N. Oka, J. Nishikawa, S. Toczko, K. T. Takahashi, M. Terazaki, T. Odate, M. Fukuchi and G. Hosie, Abundance and grazing impacts of krill, salps and copepods along the 140 °E meridian in the Southern Ocean during summer, Antarctic Sci., 2008, 20, 365–379 Search PubMed.
- V. Siegel, V. Loeb and J. Gröger, Krill (Euphausia superba) density, proportional and absolute recruitment and biomass in the Elephant Island region (Antarctic Peninsula) during the period 1977 to 1997, Polar Biol., 1998, 19, 393–398 CrossRef.
- M. J. Whitehouse, A. Atkinson and A. P. Rees, Close coupling between ammonium uptake by phytoplankton and excretion by Antarctic krill, Euphausia superba, Deep Sea Res., Part I, 2011, 58, 725–732 CrossRef CAS PubMed.
- K. R. Arrigo, D. H. Robinson, D. L. Worthen, R. B. Dunbar, G. R. DiTullio, M. VanWoert and M. P. Lizotte, Phytoplankton community structure and the drawdown of nutrients and CO2 in the Southern Ocean, Science, 1999, 283, 365–367 CrossRef CAS.
- S. S. Jacobs, On the nature and significance of the Antarctic slope front, Mar. Chem., 1991, 35, 9–24 CrossRef.
- J. Rodríguez, F. Jiménez-Gómez, J. M. Blanco and F. L. Figueroa, Physical gradients and spatial variability of the size structure and composition of phytoplankton in the Gerlache Strait (Antarctica), Deep Sea Res., Part II, 2002, 49, 693–706 CrossRef.
- I. Hense, R. Timmermann, A. Beckmann and U. V. Bathmann, Regional and interannual variability of ecosystem dynamics in the Southern Ocean, Ocean Dyn., 2003, 53, 1–10 CrossRef PubMed.
-
O. Holm-Hansen, S. Z. El-Sayed, G. A. Franceschini, R. L. Cuhel and I. Smithsonian, Primary production and the factors controlling phytoplankton growth in the Southern ocean, in Adaptations within Antarctic ecosystems, Smithsonian Institution, Houston, Texas, 1977, pp. 11–50 Search PubMed.
- W. O. Smith Jr., J. Marra, M. R. Hiscock and R. T. Barber, The seasonal cycle of phytoplankton biomass and primary productivity in the Ross Sea, Antarctica, Deep Sea Res., Part II, 2000, 47, 3119–3140 CrossRef.
- D. F. Doolittle, W. K. W. Li and M. Wood, Wintertime abundance of picoplankton in the Atlantic sector of the Southern Ocean, Nova Hedwigia, Beiheft, 2008, 133, 147–160 Search PubMed.
- D. G. Boyce, M. R. Lewis and B. Worm, Global phytoplankton decline over the past century, Nature, 2010, 466, 591–596 CrossRef CAS PubMed.
- A. Schmittner, A. Oschlies, H. D. Matthews and E. D. Galbraith, Future changes in climate, ocean circulation, ecosystems, and biogeochemical cycling simulated for a business-as-usual CO2 emission scenario until year 4000 AD, Global Biogeochem. Cycles, 2008, 22, GB1013, DOI:10.1029/2007GB002953.
- M. Montes-Hugo, S. C. Doney, H. W. Ducklow, W. Fraser, D. Martinson, S. E. Stammerjohn and O. Schofield, Recent changes in phytoplankton communities associated with rapid regional climate change along the Western
Antarctic Peninsula, Science, 2009, 323, 1470–1473 CrossRef CAS PubMed.
-
M. Hernando, I. Schloss, G. Malanga, S. Puntarulo, M. Hoffmeyer and G. Ferreyra, Impact of coastal melt-waters on the Antarctic phytoplankton, http://www.uam.es/otros/cn-scar/4th_SCAR_Open_Science/pdf/osc2010343.pdf.
- A. J. P. Houben, P. K. Bijl, J. Pross, S. M. Bohaty, S. Passchier, C. E. Stickley, U. Röhl, S. Sugisaki, L. Tauxe, T. van de Flierdt, M. Olney, F. Sangiorgi, A. Sluijs, C. Escutia, H. Brinkhuis and t. E. scientists, Reorganization of Southern Ocean plankton ecosystem at the onset of Antarctic glaciation, Science, 2013, 340, 341–344 CrossRef CAS PubMed.
- J. Overland, J. Turner, J. Francis, N. Gillett, G. Marshall and M. Tjernström, The Arctic and Antarctic: Two faces of climate change, Trans., Am. Geophys. Union, 2008, 89, 177–178 CrossRef.
- J. C. Farman, B. G. Gardiner and J. D. Shanklin, Large losses of total ozone in Antarctica reveal seasonal ClO x/NO x interaction, Nature, 1985, 315, 207–210 CrossRef CAS.
- R. L. McKenzie, P. J. Aucamp, A. F. Bais, L. O. Björn, M. Ilyas and S. Madronich, Ozone depletion and climate change: impacts on UV radiation, Photochem. Photobiol. Sci., 2011, 10, 182–198 CAS.
- R. C. Smith, B. B. Prézelin, K. S. Baker, R. R. Bidigare, N. P. Boucher, T. Coley, D. Karentz, S. MacIntyre, H. A. Matlick, D. Menzies, M. Ondrusek, Z. Wan and K. J. Waters, Ozone depletion: ultraviolet radiation and phytoplankton biology in Antarctic waters, Science, 1992, 255, 952–959 CAS.
-
C. S. Weiler and P. A. Penhale, Ultraviolet radiation in Antarctica, in Measurements and Biological Effects, Am. Geophys. Union, Washington DC, 1994 Search PubMed.
-
M. Vernet, E. A. Brody, O. Holm-Hansen and B. G. Mitchell, The response of Antarctic phytoplankton to ultraviolet radiation: absorption, photosynthesis, and taxonomic composition, in Ultraviolet Radiation in Antarctica: Mesurements and Biological Effects, ed. C. S. Weiler and P. A. Penhale, American Geophysical Union, Washington DC, 1994, pp. 207–227 Search PubMed.
- E. W. Helbling, B. E. Chalker, W. C. Dunlap, O. Holm-Hansen and V. E. Villafañe, Photoacclimation of Antarctic marine diatoms to solar ultraviolet radiation, J. Exp. Mar. Biol. Ecol., 1996, 204, 85–101 CrossRef.
- M. P. Lesser, P. J. Neale and J. J. Cullen, Acclimation of Antarctic phytoplankton to ultraviolet radiation: Ultraviolet-absorbing compounds and carbon fixation, Mol. Mar. Biol. Biotechnol., 1996, 5, 314–325 CAS.
- N. P. Boucher and B. B. Prezelin, An in situ biological weighting function for UV inhibition of phytoplankton carbon fixation in the Southern Ocean, Mar. Ecol. Prog. Ser., 1996, 144, 223–236 CrossRef.
- O. Holm-Hansen, E. W. Helbling and D. Lubin, Ultraviolet radiation in Antarctica: inhibition of primary production, Photochem. Photobiol., 1993, 58, 567–570 CrossRef CAS PubMed.
- B. Hamre, J. J. Stamnes, Ø. Frette and K. Stamnes, Could stratospheric ozone depletion lead to enhanced aquatic primary production in the polar regions?, Limnol. Oceanogr., 2008, 53, 332–338 CrossRef CAS.
- J. J. Fritz, P. J. Neale, R. F. Davis and J. A. Peloquin, Response of Antarctic phytoplankton to solar UVR exposure: Inhibition and recovery of photosynthesis in coastal and pelagic assemblages, Mar. Ecol. Prog. Ser., 2008, 365, 1–16 CrossRef CAS.
-
E. W. Helbling, V. E. Villafañe and O. Holm-Hansen, Effects of ultraviolet radiation on Antarctic marine phytoplankton photosynthesis with particular attention to the influence of mixing, in Ultraviolet Radiation in Antarctica: Mesurements and Biological Effects, ed. C. S. Weiler and P. A. Penhale, American
Geophysical Union, Washington, DC, 1994, pp. 207–227 Search PubMed.
- P. J. Neale, R. F. Davis and J. J. Cullen, Interactive effects of ozone depletion and vertical mixing on photosynthesis of Antarctic phytoplankton, Nature, 1998, 392, 585–589 CrossRef CAS PubMed.
-
H. U. Sverdrup, M. W. Johnson and R. H. Fleming, The Oceans. Their Physics, Chemistry, and General Biology, Prentice-Hall, Inc., New York (USA), 1942 Search PubMed.
- R. J. Gonçalves, M. S. Souza, J. Aigo, B. Modenutti, E. Balseiro, V. E. Villafañe, V. Cussac and E. W. Helbling, Responses of plankton and fish from temperate zones to UVR and temperature in a context of gobal change, Ecol. Austral, 2010, 20, 129–153 Search PubMed.
- V. E. Villafañe, E. S. Barbieri and E. W. Helbling, Annual patterns of ultraviolet radiation effects on temperate marine phytoplankton off Patagonia, Argentina, J. Plankton Res., 2004, 26, 167–174 CrossRef PubMed.
- A. Perelman, Z. Dubinsky and R. Martínez, Temperature dependence of superoxide dismutase activity in plankton, J. Exp. Mar. Biol. Ecol., 2006, 334, 229–235 CrossRef CAS PubMed.
- C. Sobrino and P. J. Neale, Short-term and long-term effects of temperature on photosynthesis in the diatom Thalassiosira pseudonana under UVR exposures, J. Phycol., 2007, 43, 426–436 CrossRef CAS PubMed.
- H. Bouman, T. Platt, S. Sathyendranath and V. Stuart, Dependence of light-saturated photosynthesis on temperature and community structure, Deep Sea Res., Part I, 2005, 52, 1284–1299 CrossRef PubMed.
- T. Sugawara, K. Hamasaki, T. Toda, T. Kikuchi and S. Taguchi, Response of natural phytoplankton assemblages to solar ultraviolet radiation (UV-B) in the coastal water, Japan, Hydrobiologia, 2003, 493, 17–26 CrossRef.
- E. W. Helbling, A. G. Buma, P. Boelen, H. J. Van der Strate, M. V. Fiorda Giordanino and V. E. Villafañe, Increase in Rubisco activity and gene expression due to elevated temperature partially counteracts ultraviolet radiation-induced photoinhibition in the marine diatom Thalassiosira weissflogii, Limnol. Oceanogr., 2011, 56, 1330–1342 CrossRef CAS.
-
P. J. Neale, E. W. Helbling and H. E. Zagarese, Modulation of UVR exposure and effects by vertical mixing and advection, in UV effects in aquatic organisms and ecosystems, ed. E. W. Helbling and H. E. Zagarese, Royal Society of Chemistry, 2003, pp. 108–134 Search PubMed.
- K. Gao, Z. Ruan, V. E. Villafañe, J. P. Gattuso and E. W. Helbling, Ocean acidification exacerbates the effect of UV radiation on the calcifying phytoplankter Emiliania huxleyi, Limnol. Oceanogr., 2009, 54, 1855–1862 CrossRef CAS.
- Z.-P. Mei, F. J. Saucier, V. Le Fouest, B. Zakardjian, S. Sennville, H. Xie and M. Starr, Modeling the timing of spring phytoplankton bloom and biological production of the Gulf of St. Lawrence (Canada): Effects of colored dissolved organic matter and temperature, Cont. Shelf Res., 2010, 30, 2027–2042 CrossRef PubMed.
- E. W. Helbling, D. E. Pérez, C. D. Medina, M. G. Lagunas and V. E. Villafañe, Phytoplankton distribution and photosynthesis dynamics in the Chubut River estuary (Patagonia, Argentina) throughout tidal cycles, Limnol. Oceanogr., 2010, 55, 55–65 CrossRef.
- M. Öztürk, P. L. Croot, S. Bertilsson, K. Abrahamsson, B. Karlson, R. David, A. Fransson and E. Sakshaug, Iron enrichment and photoreduction of iron under UV and PAR in the presence of hydroxycarboxylic acid: implications for phytoplankton growth in the Southern Ocean, Deep Sea Res., Part II, 2004, 51, 2841–2856 CrossRef PubMed.
- J. M. Beman, C.-E. Chow, A. L. King, Y. Feng, J. A. Fuhrman, A. Andersson, N. R. Bates, B. N. Popp and D. A. Hutchins, Global declines in oceanic nitrification rates as a consequence of ocean acidification, Proc. Natl. Acad. Sci. U. S. A., 2011, 108, 208–213 CrossRef CAS PubMed.
- P. A. Staehr and K. Sand-Jensen, Seasonal changes in temperature and nutrient
control of photosynthesis, respiration and growth of natural phytoplankton communities, Freshwater Biol., 2006, 51, 249–262 CrossRef CAS PubMed.
- E. W. Helbling, E. S. Barbieri, M. A. Marcoval, R. J. Gonçalves and V. E. Villafañe, Impact of solar ultraviolet radiation on marine phytoplankton of Patagonia, Argentina, Photochem. Photobiol., 2005, 81, 807–818 CrossRef CAS PubMed.
- D.-I. Kim, Y. Matsuyama, S. Nagasoe, M. Yamaguchi, Y.-H. Yoon, Y. Oshima, N. Imada and T. Honjo, Effects of temperature, salinity and irradiance on the growth of the harmful red tide dinoflagellate Cochlodinium polykrikoides Margalef (Dinophyceae), J. Plankton Res., 2004, 26, 61–66 CrossRef PubMed.
- J. M. Drake and D. M. Lodge, Global hot spots of biological invasions: evaluating options for ballast-water management, Proc. R. Soc. London, Ser. B, 2004, 271, 575–580 CrossRef PubMed.
- S. R. Halac, V. E. Villafañe and E. W. Helbling, Temperature benefits the photosynthetic performance of the diatoms Chaetoceros gracilis and Thalassiosira weissflogii when exposed to UVR, J. Photochem. Photobiol., B, 2010, 101, 196–205 CrossRef CAS PubMed.
- M. Thyssen, G. Ferreyra, S. Moreau, I. Schloss, M. Denis and S. Demers, The combined effect of ultraviolet B radiation and temperature increase on phytoplankton dynamics and cell cycle using pulse shape recording flow cytometry, J. Exp. Mar. Biol. Ecol., 2011, 406, 95–107 CrossRef CAS PubMed.
- E. S. Barbieri, V. E. Villafañe and E. W. Helbling, Experimental assessment of UV effects on temperate marine phytoplankton when exposed to variable radiation regimes, Limnol. Oceanogr., 2002, 47, 1648–1655 CrossRef.
- C. Mengelt and B. B. Prézelin, UVA enhancement of carbon fixation and resilience to UV inhibition in the genus Pseudo-nitzschia may provide a competitive advantage in high UV surface waters, Mar. Ecol. Prog. Ser., 2005, 301, 81–93 CrossRef CAS.
- E. Litchman and P. J. Neale, UV effects on photosynthesis, growth and acclimation of an estuarine diatom and cryptomonad, Mar. Ecol. Prog. Ser., 2005, 300, 53–62 CrossRef CAS.
- C. Llewellyn and D. Harbour, A temporal study of mycosporine-like amino acids in surface water phytoplankton from the English Channel and correlation with solar irradiation, J. Mar. Biol. Assoc. U. K., 2003, 83, 1–10 CAS.
- H. Taira, S. Aoki, B. Yamanoha and S. Taguchi, Daily variation in cellular content of UV-absorbing compounds mycosporine-like amino acids in the marine dinoflagellate Scrippsiella sweeneyae, J. Photochem. Photobiol., B, 2004, 75, 145–155 CrossRef CAS PubMed.
- J. Bouchard, M. Longhi, S. Roy, D. Campbell and G. Ferreyra, Interaction of nitrogen status and UVB sensitivity in a temperate phytoplankton assemblage, J. Exp. Mar. Biol. Ecol., 2008, 359, 67–76 CrossRef CAS PubMed.
- M. L. Longhi, G. Ferreyra, I. Schloss and S. Roy, Variable phytoplankton response to enhanced UV-B and nitrate addition in mesocosm experiments at three latitudes (Canada, Brazil and Argentina), Mar. Ecol. Prog. Ser., 2006, 313, 57–72 CrossRef CAS.
- V. E. Villafañe, P. J. Janknegt, M. de Graaff, R. J. W. Visser, W. H. van de Poll, A. G. J. Buma and E. W. Helbling, UVR-induced photoinhibition of summer marine phytoplankton communities from Patagonia, Mar. Biol., 2008, 154, 1021–1029 CrossRef.
- J. N. Bouchard, S. Roy and D. A. Campbell, UVB effects on the photosystem II-D1 protein of phytoplankton and natural phytoplankton communities, Photochem. Photobiol., 2006, 82, 936–951 CrossRef CAS PubMed.
- M. Piepho, M. T. Arts and A. Wacker, Species-specific variation in fatty acid concentrations of four phytoplankton species: Does phosphorus supply influence the effect of light intensity or temperature?, J. Phycol., 2012, 48, 64–73 CrossRef CAS PubMed.
- T. Key, A. McCarthy, D. A. Campbell, C. Six, S. Roy and Z. V. Finkel, Cell size trade-offs govern light exploitation strategies in marine phytoplankton, Environ. Microbiol., 2010, 12, 95–104 CrossRef CAS PubMed.
- R. Sommaruga, J. S. Hofer, L. Alonso-Sáez and J. M. Gasol, Differential sunlight sensitivity of picophytoplankton
from surface mediterranean coastal waters, Appl. Environ. Microbiol., 2005, 71, 2154–2157 CrossRef CAS PubMed.
- X. Yuan, K. Yin, W. Zhou, W. Cao, X. Xu and D. Zhao, Effects of ultraviolet radiation B (UV-B) on photosynthesis of natural phytoplankton assemblages in a marine bay in Southern China, Chin. Sci. Bull., 2007, 52, 545–552 CrossRef PubMed.
- M. Llabrés, S. Agustí, P. Alonso-Laita and G. Herndl,
Synechococcus and Prochlorococcus cell death induced by UV radiation and the penetration of lethal UVR in the Mediterranean Sea, Mar. Ecol. Prog. Ser., 2010, 399, 27–37 CrossRef.
- M. Llabrés and S. Agusti, Picophytoplankton cell death induced by UV radiation: evidence for oceanic Atlantic communities, Limnol. Oceanogr., 2006, 51, 21–29 CrossRef.
- M. Llabrés, S. Agustí, M. Fernández, A. Canepa, F. Maurin, F. Vidal and C. M. Duarte, Impact of elevated UVB radiation on marine biota: a meta-analysis, Global Ecol. Biogeogr., 2013, 22, 131–144 CrossRef PubMed.
- S. Agusti and M. Llabres, Solar radiationi-induced mortality of marine pico-phytoplankton in the oligotrophic ocean, Photochem. Photobiol., 2007, 83, 793–801 CrossRef CAS PubMed.
- E. W. Helbling, A. G. Buma, M. K. de Boer and V. E. Villafañe, In situ impact of solar ultraviolet radiation on photosynthesis and DNA in temperate marine phytoplankton, Mar. Ecol. Prog. Ser., 2001, 211, 43–49 CrossRef CAS.
- D. M. Martynova, N. A. Kazus, U. V. Bathmann, M. Graeve and A. A. Sukhotin, Seasonal abundance and feeding patterns of copepods Temora longicornis, Centropages hamatus and Acartia spp. in the White Sea (66 N), Polar Biol., 2011, 34, 1175–1195 CrossRef.
- H. J. De Lange and M. Lürling, Effects of UV-B irradiated algae on zooplankton grazing, Hydrobiologia, 2003, 491, 133–144 CrossRef.
- Y. Dandonneau, Y. Montel, J. Blanchot, J. Giraudeau and J. Neveux, Temporal variability in phytoplankton pigments, picoplankton and coccolithophores along a transect through the North Atlantic and tropical southwestern Pacific, Deep Sea Res., Part I, 2006, 53, 689–712 CrossRef CAS PubMed.
- E. Teira, B. Mouriño, E. Marañón, V. Pérez, M. J. Pazó, P. Serret, D. de Armas, J. Escanez, E. M. S. Woodward and E. Fernández, Variability of chlorophyll and primary production in the Eastern North Atlantic Subtropical Gyre: potential factors affecting phytoplankton activity, Deep Sea Res., Part I, 2005, 52, 569–588 CrossRef CAS PubMed.
- E. L. Venrick, The vertical distributions of chlorophyll and phytoplankton species in the North Pacific central environment, J. Plankton Res., 1988, 10(5), 987–998 CrossRef PubMed.
- Y. Chen and R. L. Siefert, Seasonal and spatial distributions and dry deposition fluxes of atmospheric total and labile iron over the tropical and subtropical North Atlantic Ocean, J. Geophys. Res., 2004, 109, D09305, DOI:10.1029/2003JD003958.
- G. T. Taylor, F. E. Muller-Karger, R. C. Thunell, M. I. Scranton, Y. Astor, R. Varela, L. T. Ghinaglia, L. Lorenzoni, K. A. Fanning and S. Hameed, Ecosystem responses in the southern Caribbean Sea to global climate change, Proc. Natl. Acad. Sci. U. S. A., 2012, 109, 19315–19320 CrossRef CAS PubMed.
- T. Smyth, Penetration of UV irradiance into the global ocean, J. Geophys. Res., 2011, 116, C11020 CrossRef.
-
D.-P. Häder, Photoinhibition and UV response in the aquatic environment, in Photoprotection, Photoinhibition, Gene Regulation, and Environment, ed. B. Demmig-Adams, W. W. Adams III and A. K. Mattoo, Springer, The Netherlands, 2006, pp. 87–105 Search PubMed.
- P. Conan, F. Joux, J.-P. Torréton, M. Pujo-Pay, T. Douki, E. Rochelle-Newall and X. Mari, Effect of solar ultraviolet radiation on bacterio-and phytoplankton activity in a large coral reef lagoon (southwest New Caledonia), Aquat. Microb. Ecol., 2008, 52, 83 CrossRef.
- K. Gao, G. Li, E. W. Helbling and V. E. Villafañe, Variability of UVR effects on photosynthesis of summer phytoplankton assemblages from a tropical coastal area of the South China Sea, Photochem. Photobiol., 2007, 83, 802–809 CrossRef CAS PubMed.
- G. Li and K. Gao, Cell size-dependent effects of solar UV radiation on primary production in coastal waters of the South China Sea, Estuaries Coasts, 2013, 1–9 Search PubMed.
- W. Guan and K. Gao, Impacts of UV radiation on photosynthesis and growth of the coccolithophore Emiliania huxleyi (Haptophyceae), Environ. Exp. Bot., 2010, 67, 502–508 CrossRef CAS PubMed.
- W. Zhou, K. Yin, X. Yuan and X. Ning, Comparison of the effects of short-term UVB radiation exposure on phytoplankton photosynthesis in the temperate Changjiang and subtropical Zhujiang estuaries of China, J. Oceanogr., 2009, 65, 627–638 CrossRef PubMed.
- E. W. Helbling, K. Gao, R. J. Gonçalves, H. Wu and V. E. Villafañe, Utilization of solar UV radiation by coastal phytoplankton assemblages off SE China when exposed to fast mixing, Mar. Ecol. Prog. Ser., 2003, 259, 59–66 CrossRef CAS.
-
A. D. McKinnon, A. J. Richardson, M. A. Burford and M. J. Furnas, in Effects on the Reef, The Great Barrier Reef Marine Park Authority, 2007 Search PubMed.
- S. R. Halac, S. D. Guendulain-García, V. E. Villafañe, E. W. Helbling and A. T. Banaszak, Responses of tropical plankton communities from the Mexican Caribbean to solar ultraviolet radiation exposure and increased temperature, J. Exp. Mar. Biol. Ecol., 2013, 445, 99–107 CrossRef PubMed.
- M. van den Belt, O. A. Bianciotto, R. Costanza, S. Demers, S. Diaz, G. A. Ferreyra, E. W. Koch, F. R. Momo and M. Vernet, Mediated modeling of the impacts of enhanced UV-B radiation on ecosystem services, Photochem. Photobiol., 2006, 82, 865–877 CrossRef CAS PubMed.
- K.-H. Gin, S. Koh and I.-I. Lin, Spectral irradiance profiles of suspended marine clay for the estimation of suspended sediment concentration in tropical waters, Int. J. Remote Sens., 2003, 24, 3235–3245 CrossRef.
- V. Kitidis, A. P. Stubbins, G. Uher, R. C. Upstill Goddard, C. S. Law and E. M. S. Woodward, Variability of chromophoric organic matter in surface waters of the Atlantic Ocean, Deep Sea Res., Part II, 2006, 53, 1666–1684 CrossRef PubMed.
- M. Tedetti and R. Sempéré, Penetration of ultraviolet radiation in the marine environment. A review, Photochem. Photobiol., 2006, 82, 389–397 CrossRef CAS PubMed.
- S. R. Erga, K. Aursland, Ø. Frette, B. Hamre, J. K. Lotsberg, J. Stamnes, J. Aure, F. Rey and K. Stamnes, UV transmission in Norwegian marine waters: controlling factors and possible effects on primary production and vertical distribution of phytoplankton, Mar. Ecol. Prog. Ser., 2005, 305, 79–100 CrossRef.
- A. G. J. Buma, R. J. Visser, W. Van de Poll, V. E. Villafañe, P. J. Janknegt and E. W. Helbling, Wavelength-dependent xanthophyll cycle activity in marine microalgae exposed to natural ultraviolet radiation, Eur. J. Phycol., 2009, 44, 515–524 CrossRef CAS.
- L. M. Ayoub, P. Hallock, P. G. Coble and S. S. Bell, MAA-like absorbing substances in Florida Keys phytoplankton vary with distance from shore and CDOM: Implications for coral reefs, J. Exp. Mar. Biol. Ecol., 2012, 420, 91–98 CrossRef PubMed.
- G. Li, K. Gao and G. Gao, Differential impacts of solar UV radiation on photosynthetic carbon fixation from the coastal to offshore surface waters in the South China Sea, Photochem. Photobiol., 2011, 87, 329–334 CrossRef CAS PubMed.
- F. Joux, W. H. Jeffrey, M. Abboudi, J. Neveux, M. Pujo-Pay, L. Oriol and J. J. Naudin, Ultraviolet radiation in the Rhone river lenses of low salinity and in marine waters of the northwestern Mediterranean sea: Attenuation and effects on bacterial activities and net community production, Photochem. Photobiol., 2009, 85, 783–793 CrossRef CAS PubMed.
- V. Stuart, O. Ulloa, G. Alarcón, S. Sathyendranath, H. Major, E. Head and T. Platt, Bio-optical characteristics of phytoplankton populations in the upwelling system off the coast of Chile, Rev. Chil. Hist. Nat., 2004, 77, 1 Search PubMed.
- M. A. Marcoval, V. E. Villafañe and E. W. Helbling, Combined effects of solar ultraviolet radiation and nutrients addition on growth, biomass and taxonomic composition of coastal marine phytoplankton communities of Patagonia, J. Photochem. Photobiol., B, 2008, 91, 157–166 CrossRef CAS PubMed.
- L. Aubriot, D. Conde, S. Bonilla and R. Sommaruga, Phosphate uptake behavior of natural phytoplankton during exposure to solar ultraviolet radiation in a shallow coastal lagoon, Mar. Biol., 2004, 144, 623–631 CrossRef CAS.
- A. Keller, P. Hargraves, H. Jeon, G. Klein-MacPhee, E. Klos, C. Oviatt and J. Zhang, Effects of ultraviolet-B enhancement on marine trophic levels in a stratified coastal system, Mar. Biol., 1997, 130, 277–287 CrossRef.
- S. Roy, B. Mohovic, S. M. Gianesella, I. Schloss, M. Ferrario and S. Demers, Effects of enhanced UV-B on pigment-based phytoplankton biomass and composition of mesocosm-enclosed natural marine communities from three latitudes, Photochem. Photobiol., 2006, 82, 909–922 CrossRef CAS.
- C. M. Crain, B. S. Halpern, M. W. Beck and C. V. Kappel, Understanding and managing human threats to the coastal marine environment, Ann. N. Y. Acad. Sci., 2009, 1162, 39–62 CrossRef PubMed.
- N. Bihari, B. Hamer and B. Kralj-Bilen, PAH content, toxicity and genotoxicity of coastal marine sediments from the Rovinj area, Northern Adriatic, Croatia, Sci. Total Environ., 2006, 366, 602–611 CrossRef CAS PubMed.
- R. B. Peachey, The synergism between hydrocarbon pollutants and UV radiation: a potential link between coastal pollution and larval mortality, J. Exp. Mar. Biol. Ecol., 2005, 315, 103–114 CrossRef CAS PubMed.
- K. Ohwada, M. Nishimura, M. Wada, H. Nomura, A. Shibata, K. Okamoto, K. Toyoda, A. Yoshida, H. Takada and M. Yamada, Study of the effect of water-soluble fractions of heavy-oil on coastal marine organisms using enclosed ecosystems, mesocosms, Mar. Pollut. Bull., 2003, 47, 78–84 CrossRef CAS.
- N. Salas, L. Ortiz, M. Gilcoto, M. Varela, J. Bayona, S. Groom, X. A. Álvarez-Salgado and J. Albaiges, Fingerprinting petroleum hydrocarbons in plankton and surface sediments during the spring and early summer blooms in the Galician coast (NW Spain) after the Prestige oil spill, Mar. Environ. Res., 2006, 62, 388–413 CrossRef CAS PubMed.
- P. Sargian, B. Mostajir, K. Chatila, G. A. Ferreyra, É. Pelletier and S. Demers, Non-synergistic effects of water-soluble crude oil and enhanced ultraviolet-B radiation on a natural plankton assemblage, Mar. Ecol. Prog. Ser., 2005, 294, 63–77 CrossRef CAS.
- E. Pelletier, P. Sargian, J. Payet and S. Demers, Ecotoxicological effects of combined UVB and organic contaminants in coastal waters: a review, Photochem. Photobiol., 2006, 82, 981–993 CrossRef CAS.
- P. Echeveste, S. Agustí and J. Dachs, Cell size dependence of additive versus synergetic effects of UV radiation and PAHs on oceanic phytoplankton, Environ. Pollut., 2011, 159, 1307–1316 CrossRef CAS PubMed.
- G. J. Herndl, A. Brugger, S. Hager, E. Kaiser, I. Obernosterer, B. Reitner and D. Slezak, Role of ultraviolet-B radiation on bacterioplankton and the availability of dissolved organic matter, Plant Ecol., 1997, 128, 42–51 Search PubMed.
- C. Romera-Castillo, H. Sarmento, X. A. Álvarez-Salgado, J. M. Gasol and C. Marrasé, Net production and consumption of fluorescent colored dissolved organic matter by natural bacterial assemblages growing on marine phytoplankton exudates, Appl. Environ. Microbiol., 2011, 77, 7490–7498 CrossRef CAS PubMed.
- M. Nieto-Cid, X. A. Álvarez-Salgado and F. F. Pérez, Microbial and photochemical reactivity of fluorescent dissolved organic matter in a coastal upwelling system, Limnol. Oceanogr., 2006, 51, 1391–1400 CrossRef CAS.
- E. Kaiser and G. J. Herndl, Rapid recovery of marine bacterioplankton activity after inhibition by UV radiation in coastal waters, Appl. Environ. Microbiol., 1997, 63, 4026–4031 CAS.
- K. L. Hernández, R. A. Quiñones, G. Daneri, M. E. Farías and E. W. Helbling, Solar UV radiation modulates daily production and DNA damage of marine bacterioplankton from a productive upwelling zone (36 °S), Chile, J. Exp. Mar. Biol. Ecol., 2007, 343, 82–95 CrossRef PubMed.
- L. Alonso-Sáez, J. M. Gasol, T. Lefort, J. Hofer and R. Sommaruga, Effect of natural sunlight on bacterial activity and differential sensitivity of natural bacterioplankton groups in northwestern Mediterranean coastal waters, Appl. Environ. Microbiol., 2006, 72, 5806–5813 CrossRef PubMed.
- D. P. Morris, H. Zagarese, C. E. Williamson, E. G. Balseiro, B. R. Hargreaves, B. Modenutti, R. Moeller and C. Queimalinos, The attenuation of solar UV radiation in lakes and the role of dissolved organic carbon, Limnol. Oceanogr., 1995, 40, 1381–1391 CrossRef CAS.
- M. T. Arts, R. D. Robarts, F. Kasai, M. J. Waiser, V. P. Tumber, A. J. Plante, H. Rai and H. J. De Lange, The attenuation of ultraviolet radiation in high dissolved organic carbon waters of wetlands and lakes on the northern Great Plains, Limnol. Oceanogr., 2000, 45, 292–299 CrossRef CAS.
-
(a) B. E. Modenutti, E. G. Balseiro, J. J. Elser, M. Bastidas Navarro, F. Cuassolo, C. Laspoumaderes, M. S. Souza and M. S. Díaz Villanueva, Effect of volcanic eruption on nutrients, light, and phytoplankton in oligotrophic lakes, Limnol. Oceanogr., 2013, 58, 1165–1175 Search PubMed;
(b) P. Carrillo, J. A. Delgado-Molina, J. M. Medina-Sánchez, F. J. Bullejos and M. Villar-Argaiz, Phosphorus inputs unmask negative effects of ultraviolet radiation on algae in a high mountain lake, GCB, 2008, 14, 423–439 CrossRef PubMed.
- R. J. Goncalves, E. S. Barbieri, V. E. Villafane and E. W. Helbling, Motility of Daphnia spinulata as affected by solar radiation throughout an annual cycle in mid-latitudes of Patagonia, Photochem. Photobiol., 2007, 83, 824–832 CrossRef CAS PubMed.
- R. Sommaruga and G. Augustin, Seasonality in UV transparency of an alpine lake is associated to changes in phytoplankton biomass, Aquat. Sci., 2006, 68, 129–141 CrossRef.
- M. Alam, N. Jahan, L. Thalib, B. Wei and T. Maekawa, Effects of environmental factors on the seasonally change of phytoplankton populations in a closed freshwater pond, Environ. Int., 2001, 27, 363–371 CrossRef CAS.
- M. Søndergaard, N. H. Borch and B. Riemann, Dynamics of biodegradable DOC produced by freshwater plankton communities, Aquat. Microb. Ecol., 2000, 23, 73–83 CrossRef.
-
M. Blumthaler and A. R. Webb, UVR climatology, in UV effects in aquatic organisms and ecosystems, ed. E. W. Helbling and H. E. Zagarese, The Royal Society of Chemistry, Cambridge, 2003, pp. 21–58 Search PubMed.
- R. Sommaruga, The role of solar UV radiation in the ecology of alpine lakes, J. Photochem. Photobiol., B, 2001, 62, 35–42 CrossRef CAS.
- W. B. Keller, J. Heneberry, J. Leduc, J. Gunn and N. Yan, Variations in epilimnion thickness in small boreal shield lakes: Relationships with transparency, weather and acidification, Environ. Monit. Assess., 2006, 115, 419–431 CrossRef CAS PubMed.
- A. M. Paterson, K. M. Somers, P. J. Dillon, J. Heneberry, W. B. Keller and A. Ford, Relationships between dissolved organic carbon concentrations, weather, and acidification in small Boreal Shield lakes, Can. J. Fish. Aquat. Sci., 2008, 65, 786–795 CrossRef.
- J. W. Harrison and R. E. H. Smith, Effects of ultraviolet radiation on the productivity and composition of freshwater phytoplankton communities, Photochem. Photobiol. Sci., 2009, 8, 1218–1232 CAS.
- M. C. Marinone, S. M. Marque, D. A. Suárez, M. del Carmen Diéguez, P. Pérez, P. De Los Ríos, D. Soto and H. E. Zagarese, UV radiation as a potential driving force for zooplankton community structure in Patagonian lakes, Photochem. Photobiol., 2006, 82, 962–971 CrossRef CAS PubMed.
- M. Jansson, A.-K. Bergström, P. Blomqvist and S. Drakare, Allochthonous organic carbon and phytoplankton/bacterioplankton production relationships in lakes, Ecology, 2000, 81, 3250–3255 CrossRef.
- H. J. De Lange, D. P. Morris and C. E. Williamson, Solar ultraviolet photodegradation of DOC may stimulate freshwater food webs, J. Plankton Res., 2003, 25, 111–117 CrossRef CAS PubMed.
- J. Köhler, M. Schmitt, H. Krumbeck, M. Kapfer, E. Litchman and P. J. Neale, Effects of UV on carbon assimilation of phytoplankton in a mixed water column, Aquat. Sci., 2001, 63, 294–309 CrossRef.
- M. A. Xenopoulos, P. C. Frost and J. J. Elser, Joint effects of UV radiation and phosphorus supply on algal growth rate and elemental composition, Ecology, 2002, 83, 423–435 CrossRef.
- M. A. Xenopoulos and P. C. Frost, UV radiation, phosphorus, and their combined effects on the taxonomic composition of phytoplankton in a boreal lake, J. Phycol., 2003, 39, 291–302 CrossRef CAS.
- M. Villar-Argaiz, J. M. Medina-Sánchez, F. J. Bullejos, J. A. Delgado-Molina, O. R. Pérez and J. C. Navarro, UV radiation and phosphorus interact to influence the biochemical composition of phytoplankton, Freshwater Biol., 2009, 54, 1233–1245 CrossRef CAS PubMed.
- H. J. De Lange and P. L. Van Reeuwijk, Negative effects of UVB-irradiated phytoplankton on life history traits and fitness of Daphnia magna, Freshwater Biol., 2003, 48, 678–686 CrossRef.
- A. E. Mcnamara and W. R. Hill, UV-B irradiance gradient affects photosynthesis and pigments but not food quality of periphyton, Freshwater Biol., 2000, 43, 649–662 CrossRef.
- R. P. Sinha and D.-P. Häder, UV-induced DNA damage and repair: a review, Photochem. Photobiol. Sci., 2002, 1, 225–236 CAS.
- R. P. Sinha, M. Dautz and D.-P. Häder, A simple and efficient method for the quantitative analysis of thymine dimers in cyanobacteria, phytoplankton and macroalgae, Acta Protozool., 2001, 40, 187–195 CAS.
- Y. Y. He and D.-P. Häder, Reactive oxygen species and UV-B: effect on cyanobacteria, Photochem. Photobiol. Sci., 2002, 1, 729–736 CAS.
- L. Chen, S. Deng, R. De Philippis, W. Tian, H. Wu and J. Wang, UV-B resistance as a criterion for the selection of desert microalgae to be utilized for inoculating desert soils, J. Appl. Phycol., 2013, 25, 1009–1015 CrossRef CAS.
- C. Callieri, G. Morabito, Y. Huot, P. J. Neale and E. Litchman, Photosynthetic response of pico-and nanoplanktonic algae to UVB, UVA and PAR in a high mountain lake, Aquat. Sci., 2001, 63, 286–293 CrossRef.
- P. Li, W. Liu and K. Gao, Effects of temperature, pH, and UV radiation on alkaline phosphatase activity in the terrestrial cyanobacterium Nostoc flagelliforme, J. Appl. Phycol., 2012, 25, 1031–1038 CrossRef.
- J. Fauchot, M. Gosselin, M. Levasseur, B. Mostajir, C. Belzile, S. Demers, S. Roy and P. Z. Villegas, Influence of UV-B radiation on nitrogen utilization by a natural assemblage of phytoplankton, J. Phycol., 2000, 36, 484–496 CrossRef CAS.
- I. Laurion, A. Lami and R. Sommaruga, Distribution of mycosporine-like amino acids and photoprotective carotenoids among freshwater phytoplankton assemblages, Aquat. Microb. Ecol., 2002, 26, 283–294 CrossRef.
- R. P. Sinha, S. P. Singh and D.-P. Häder, Database on mycosporines and mycosporine-like amino acids (MAAs) in fungi, cyanobacteria, macroalgae, phytoplankton and animals, J. Photochem. Photobiol., B, 2007, 89, 29–35 CrossRef CAS PubMed.
- A. Gröniger, R. P. Sinha, M. Klisch and D.-P. Häder, Photoprotective compounds in cyanobacteria, phytoplankton
and macroalgae - a database, J. Photochem. Photobiol., B, 2000, 58, 115–122 CrossRef.
- Z. Liu, D.-P. Häder and R. Sommaruga, Occurrence of mycosporine-like amino acids (MAAs) in the bloom-forming cyanobacterium Microcystis aeruginosa, J. Plankton Res., 2004, 26, 963–966 CrossRef CAS PubMed.
- P. Blokker, S. Schouten, J. W. de Leeuw, J. S. Sinnighe Damst and H. van den Ende, Molecular structure of the resistant biopolymer in zygospore cell walls of Chlamydomonas monoica, Planta, 1999, 207, 539–543 CrossRef CAS.
- H. L. Gorton and T. C. Vogelmann, Ultraviolet radiation and the snow alga Chlamydomonas nivalis (Bauer) Wille, Photochem. Photobiol., 2003, 77, 608–615 CrossRef CAS.
- F. Xiong, J. Komenda, J. Kopecky and L. Nedbal, Strategies of ultraviolet-B protection in microscopic algae, Physiol. Plant., 1997, 100, 378–388 CrossRef CAS PubMed.
- F. Pescheck, K. Bischof and W. Bilger, Screening of ultraviolet-A and ultraviolet-B radiation in marine green macroalgae (Chlorophyta), J. Phycol., 2010, 46, 444 CrossRef CAS PubMed.
- K. P. Van Winkle-Swift and W. L. Rickoll, The zygospore wall of Chlamydomonas monoica (Chlorophyceae): morphogenesis and evidence for the presence of sporopollenin, J. Phycol., 1997, 33, 655–665 CAS.
|
This journal is © The Royal Society of Chemistry and Owner Societies 2014 |