Chiroptical molecular propellers based on hexakis(phenylethynyl)benzene through the complexation-induced intramolecular transmission of local point chirality†
Received
28th July 2014
, Accepted 28th August 2014
First published on 28th August 2014
Abstract
We designed hexakis(phenylethynyl)benzene derivatives with a tertiary amide group on each blade to achieve a helically biased propeller arrangement through the complexation-induced intramolecular transmission of point chirality. A hydrogen-bonding ditopic guest was captured at two amide groups, and thus could pair two neighboring blades to form a supramolecular cyclic structure, in which an auxiliary chiral group associated with a blade acted as a chiral handle to control the helical bias, while the chiral auxiliary did not exert any helical influence on the dynamic helicity in the absence of a guest due to the high flexibility of each blade.
Introduction
Molecular propellers1,2 are interesting in terms of helical chirality in addition to actual helices such as polymeric chains3 and helicenes.4 Among them, dynamic molecules that show interconversion between conformations with (M)- or (P)-helicity2 are suitable for the studies on transmission of chirality,5 which is the central issue in the design of functional molecules, e.g. asymmetric catalysts, sensors, and memory materials. To investigate and utilize helical chirality based on a dynamic molecular propeller, the molecule should be designed to satisfy the following requirements: (1) all blades around the central core should twist in a conrotatory manner, and (2) the helical preference should show a particular bias. However, it is not sufficient to develop a methodology for both constructing a propeller-shaped molecule and biasing the dynamic helicity to prefer a particular sense at the molecular level. Previously, we reported the design of chiroptical molecular propellers based on tertiary tetraarylterephthalamides,6 in which six sp2 carbons of four aryl and two amide blades were directly connected to the benzene ring as a central core, and the molecules satisfied the above two requirements even in solution, as well as in the crystal form. We have recently been studying the framework of hexakis(phenylethynyl)benzenes (HPEBs),2a,b,7,8 in which six phenyl groups are connected to a central benzene ring through a triple bond to give an extended π-electron system. HPEBs have also provided molecular motifs for studies on columnar assemblies by stacking,2a,7a including discotic liquid crystals,9 and unique optical properties based on the large π-plane.7a–e,10 The peripheral phenyl rings rotate freely about the single bond and the molecule can adopt many different conformations. Among these numerous conformations, only the two conformers in which all six blades are twisted in a conrotatory manner are regarded as chiral propellers (Scheme 1). It is a challenging task to force the molecule to adopt propeller conformations based on the HPEB motif at the molecular level, and to purposefully control the dynamic helicity to prefer a particular sense, while there have been a few reports that a helical conformation helped to produce enhanced chiroptical signals in a helically stacked assembly.7a
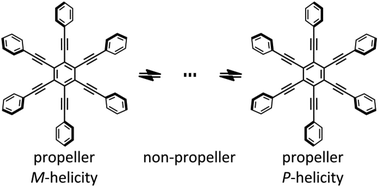 |
| Scheme 1 Diversity in the conformation of hexakis(phenylethynyl)benzene (HPEB) including chiral propellers with (M)- or (P)-helicity. | |
In this study, we designed HPEBs 1 with a tertiary amide group on each blade (Fig. 1). The chiral auxiliary [(R)-C*HMe(cHex)] on the amide nitrogen should provide a local chiral space in the blade, but should not collaborate with the neighboring blades to force the whole molecule to adopt a helically-biased propeller arrangement due to the high flexibility of the peripheral phenyl rings. Therefore, we would need to pair two neighboring blades to achieve chiral communication between blades.8 We envisioned that a ditopic guest molecule would bind at the two amide carbonyls in a pair of neighboring blades through hydrogen bonds to form a supramolecular cyclic structure, in which the two blades would be forced to work in collaboration with each other by twisting in a conrotatory manner and the local point chirality would act as a chiral handle to control the direction of twisting to prefer a particular sense of (M)- or (P)-helicity. Consequently, the 1
:
3 complexation of (R,R,R)-1b [X = CH2(cHex), Y = (R)-C*HMe(cHex)] or (R,R,R,R,R,R)-1c [X = Y = (R)-C*HMe(cHex)] with an achiral ditopic guest would lead to a C3-symmetric propeller arrangement in the HPEB framework, and the helical preference of the propeller would be biased by the complexation-induced intramolecular transmission of local point chirality (R) in each of the three supramolecular cyclic structures (Scheme 2). We describe below the details of a method for the complexation-induced intramolecular transmission of chirality on the basis of results with double-bladed substructures 2, which are 1,2-bis(phenylethynyl)benzene derivatives that are considered to be one-third of the HPEB framework (Fig. 1), and then apply this method to the HPEB framework to achieve enhanced chiroptical signals from (R,R,R)-1b and (R,R,R,R,R,R)-1c in their complexed states.
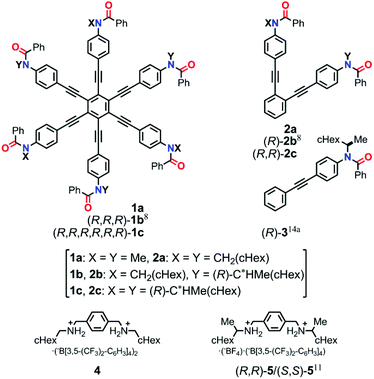 |
| Fig. 1 Chemical structures of HPEBs 1, double-bladed 2, single-bladed 3, and ditopic guests 4 and 5. | |
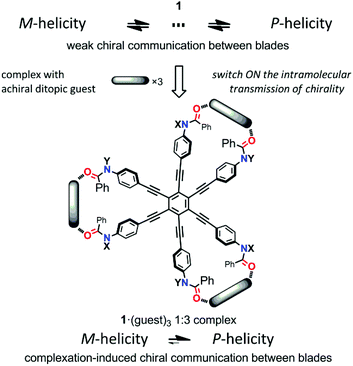 |
| Scheme 2 Generation of a chiroptical propeller through the complexation-induced intramolecular transmission of point chirality. | |
Results and discussion
Molecular design and preparation
We used three combinations of auxiliaries (X and Y) on the amide nitrogens in HPEBs 1 and the double-bladed substructures 2 (Fig. 1): neither X nor Y has a chiral carbon for 1a and 2a, only Y has a chiral carbon for (R,R,R)-1b and (R)-2b,8 and both X and Y have a chiral carbon for (R,R,R,R,R,R)-1c and (R,R)-2c. D6h-Symmetric 1a and C6-symmetric (R,R,R,R,R,R)-1c were prepared by Sonogashira coupling reactions of hexachlorobenzene7d with the corresponding phenylacetylenes. Double-bladed substructures 2a and (R,R)-2c were also obtained by Sonogashira coupling reactions, and an achiral ditopic hydrogen-bonding guest 4, without any chiral element, was prepared by acidification of a known 1,4-xylylenediamine,12 followed by counter anion exchange to achieve high solubility in organic media, and used for complexation with 1 or 2 (Scheme S1†).
Molecular structure and spectroscopic characterization of double-bladed substructures 2
A single-crystal X-ray analysis for 2a demonstrated a helical conformation with the two phenylethynyl blades twisting in a conrotatory manner and the two benzoyl groups adopting a cis-conformation13 and facing inwards (Fig. 2). In the crystal, two conformers with (M)- or (P)-helicity were present in 1
:
1 ratio. In a conformational search for a model 2a′ [X = Y = Me], we found that a conformation similar to that seen in the crystal of 2a was the most energy-minimized structure (Fig. 3).
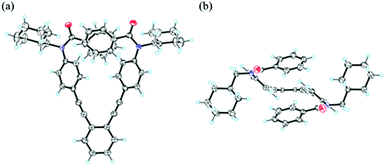 |
| Fig. 2 X-ray structure of (P)-2a [X = Y = CH2(cHex)] (P21/c, Z = 4) in rac-2a·chloroform crystal: (a) top view and (b) side view. The crystallization solvent is omitted for clarity. | |
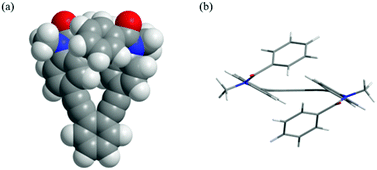 |
| Fig. 3 Energy-minimized structure for 2a′ [X = Y = Me] obtained by a conformational search with the MacroModel software (v9.9 Monte Carlo Multiple Minimum method, OPLS_2005, non-solvated, 20 000 steps): (a) top view (space filling representation) and (b) side view (stick representation). Only one of the enantiomeric conformations with (M)- or (P)-helicity is depicted. | |
The 1H NMR spectrum of 2a showed only one set of averaged resonances, which was assigned to a single C2v-symmetric species in solution (Fig. S1†). The observed symmetry was explained by assuming high flexibility in the para-phenylene and benzoyl groups, involving interconversion between the two energetically-equivalent helical conformations with (M)- or (P)-helicity (dynamic helicity). The UV spectra of 2a–c showed two major absorptions that were assigned to the diphenylacetylene unit (293 nm for 2a, 291 nm for 2b, and 289 nm for 2c) and the longer conjugation through the ortho-phenylene group (around 330 nm sh) (Fig. 4a),14 and the similarity in absorption suggested that a common structure was present in solution for the double-bladed substructures 2.15 In the UV spectrum of single-bladed 3, which is a diphenylacetylene derivative, we observed only one set of absorptions around 291 nm, as expected (Fig. 4a).14
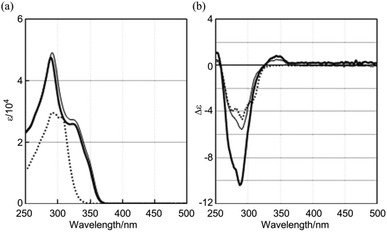 |
| Fig. 4 (a) UV and (b) CD spectra of (R)-2b (thin line), (R,R)-2c (thick line), and (R)-3 (dashed line), measured in CH2Cl2 at room temperature. | |
The CD spectrum of (R)-2b mainly showed negatively-signed Cotton effects around 290 nm (Fig. 4b), which did not seem to be due to a helically-biased double-bladed dynamic helicity, but rather mainly resulted from an interaction between a benzoyl group and a phenylethynyl blade (weak chiral communication between blades). This idea was supported by the fact that quite similar Cotton effects were also present in the spectrum of single-bladed (R)-3, which is a diphenylacetylene derivative with the same chiral auxiliary [(R)-C*HMe(cHex)] on the amide nitrogen. In the spectrum of (R,R)-2c with the same chiral auxiliary attached to each of the two blades, the molar CDs around 290 nm were simply doubled compared to those of (R)-2b or (R)-3 (Fig. 4b), which indicated that the local chirality on each blade did not significantly induce a preference regarding the double-bladed dynamic helicity, but presented a local chiral space for each blade independently.15
Complexation-induced intramolecular transmission of local point chirality
First, we investigated the 1
:
1 complexation of a double-bladed substructure 2 with a ditopic guest by 1H NMR spectroscopy. The stoichiometry was confirmed to be 1
:
1 by a Job plot for the complexation of 2a with (R,R)-5
11 based on a continuous change in the chemical shift of 2a (Fig. 5a). We estimated the association constant (2 × 103 M−1) for the 1
:
1 complexation through a titration experiment, followed by a curve-fitting method (Fig. S3a†). Then, we monitored the complexation of 2a, which has no chiral element other than the dynamic helicity interconverting between (M)- and (P)-helical conformations, with chiral ditopic guests (R,R)-5/(S,S)-5 by CD spectroscopy. In the CD spectrum of 2a, we found that bisignated Cotton effects were induced in the absorption region of 2a upon the gradual addition of (R,R)-5 into a solution of 2a [λext(Δε) 287 nm (−6), 343 nm (+2)] (Fig. 5b). Mirror images of the supramolecularly-induced Cotton effects were obtained using (S,S)-5 instead of (R,R)-5 (Fig. 5b), and indicated that the dynamic helicity of 2a was biased to prefer a particular sense in each of the enantiomeric complexes through the supramolecular transmission of chirality in a guest to the double-bladed dynamic helicity of the host. These bisignated Cotton effects were considered to be the same as those seen around at 350 nm in the spectrum of (R)-2b or (R,R)-2c,15 and are discussed again in the following experiments.
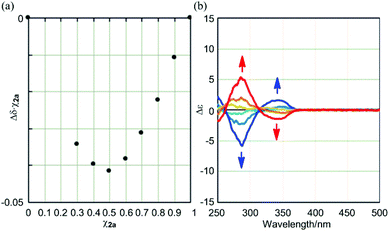 |
| Fig. 5 (a) Job plot for the complexation of 2a with (R,R)-5 in CDCl3 at 303 K using continuous changes (Δδ = δ2a(R,R)-5 − δ2a) in the chemical shift for phenylene protons (close to the amide group) in the blades ([2a] + [5] = 2 mM). Some chemical shifts were not recorded due to peak-broadening in the region of 0 < χ2a < 0.3; (b) continuous changes in the CD spectrum of 2a (3.5 × 10−4 M) upon complexation with a chiral ditopic guest (R,R)-5 (blue lines; 1, 2, and 4 equiv.) or (S,S)-5 (red lines; 1, 2, and 4 equiv.), measured in CH2Cl2 at room temperature. Molar CDs from the chiral guests 5 were very small (Δε < ±0.1) in their absorption region.11 | |
We describe here a method for the complexation-induced intramolecular transmission of chirality: the local point chirality associated with a blade is intramolecularly transferred to the dynamic helicity to act as a chiral handle only in the supramolecular cyclic structure formed by complexation, leading to a biased helicity through the complexation-induced communication of chirality between blades (Scheme 3). We examined the complexation of (R)-2b with the achiral ditopic guest 4, which has no preference for a particular sense, upon the gradual addition of 4 to a solution of (R)-2b. The Cotton effects were remarkably changed from the original ones of (R)-2b itself to show a positive couplet [λext(Δε) 285 nm (−14), 329 nm (+5)] (Fig. 6a). This continuous change in the CD spectrum indicated that the local point chirality associated with one of the two blades of (R)-2b was intramolecularly transferred to the double-bladed dynamic helicity by the formation of a supramolecular cyclic structure to give one-third of a propeller arrangement based on the HPEB framework, and the helical preference was biased to a particular handedness. This is also the case for a complex of (R,R)-2c with 4, and the induced Cotton effects were similar in appearance, and greatly enhanced (Fig. 6b).16 As a control experiment to confirm that induced changes in UV and CD spectra were due to a complexed species through hydrogen bonds, we added acetonitrile (10 wt%) to a solution of (R,R)-2c in CH2Cl2 in the presence of 4, and confirmed that no change was induced in both UV and CD spectra of (R,R)-2c (Fig. S4†).
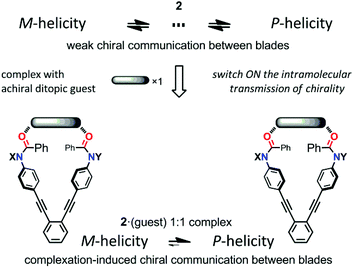 |
| Scheme 3 The intramolecular transmission of chirality in a supramolecular cyclic structure that was formed by complexation with an achiral ditopic guest. | |
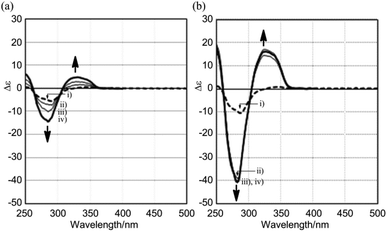 |
| Fig. 6 Continuous changes in the CD spectra of (a) (R)-2b (2.6 × 10−4 M) and (b) (R,R)-2c (2.2 × 10−4 M), upon complexation with achiral ditopic guest 4 [(i) 0 equiv. (2 only, dashed line), (ii) 1 equiv., (iii) 2 equiv., and (iv) 4 equiv. (solid lines)]. All spectra were measured in CH2Cl2 at room temperature. | |
Molecular structure and spectroscopic characterization of HPEBs 1, and formation of a chiroptical molecular propeller
A single-crystal X-ray analysis for 1a showed a centrosymmetric structure (P
, Z = 2), in which four blades were twisted and the rest were planar toward the central benzene core (Fig. 7).17 All amide groups adopted a cis-conformation as in the case of 2a.
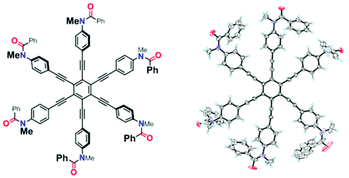 |
| Fig. 7 X-ray structure of 1a·H2O [X = Y = Me] (P , Z = 2). The crystal contained water, which is omitted for clarity. | |
In a conformational search for 1a, a C3-symmetric propeller arrangement was predicted as the most energy-minimized structure (Fig. 8), and is considered to be composed of a threefold helical double-bladed substructure.
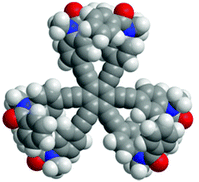 |
| Fig. 8 Energy-minimized structure for 1a [X = Y = Me] obtained by a conformational search with the MacroModel software (v9.9 Monte Carlo Multiple Minimum method, OPLS_2005, non-solvated, 50 000 steps). Only one of the enantiomeric conformations with (M)- or (P)-helicity is depicted. | |
In the 1H NMR spectra of 1, only one set of averaged resonances for phenylene protons among all of the HPEBs was observed (Fig. S1†), and these observed symmetries indicated that a single species with (pseudo)six-fold symmetry was present in solution, even for (R,R,R)-1c, which actually reflects C3 symmetry. As was demonstrated for 2, these averaged resonances suggested high flexibility in the para-phenylene and benzoyl groups of 1 in solution. The UV-vis spectra of 1 showed typical absorptions for HPEBs consisting of a maximum (367 nm for 1a, 368 nm for 1b, and 366 nm for 1c) and a shoulder (around at 390 nm) (Fig. 9a), both of which underwent bathochromic shifts compared to the corresponding absorptions of the parent HPEB (350 nm and 370(sh) nm, respectively)7f due to para-substitution in the blade.7c,d As in the case for 2, the similarity in absorption suggested that a common structure was present in solution for HPEBs 1, regardless of the bulkiness of an auxiliary on the amide nitrogen.
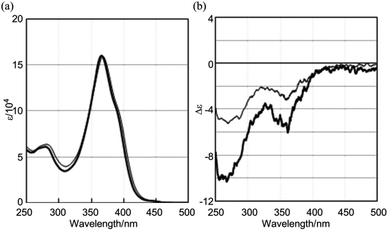 |
| Fig. 9 (a) UV-vis and (b) CD spectra of (R,R,R)-1b (thin line) and (R,R,R,R,R,R)-1c (thick line), measured in CH2Cl2 at room temperature. | |
The CD spectra of (R,R,R)-1b and (R,R,R,R,R,R)-1c were similar with regard to the shape of the two major negatively-signed Cotton effects, and their molar CDs increased negatively with an increase in the number of chiral auxiliaries [λext(Δε) around 270 nm (−5 for 1b and −10 for 1c) and around 360 nm (−3 for 1b and −6 for 1c)] (Fig. 9b). The spectral shape was different than those of double-bladed (R)-2b or single-bladed (R)-3 (Fig. 5b), and therefore the spectral intensities were not simply tripled or sextupled compared to those of (R)-2b or (R)-3 due to the increase in associated chromophores. The simple duplication of molar CDs in the CD spectra of (R,R,R)-1b and (R,R,R,R,R,R)-1c was accounted for by the absence of chiral communication between blades, as in the case for (R)-2b and (R,R)-2c, rather than by a biased propeller-shaped helicity in 1b and 1c, even though a helical conformation was predicted for 1 as the most stable structure by a conformational search (Fig. 8). We indeed found remarkable changes in the CD spectrum of (R,R,R)-1b upon gradual addition of the achiral ditopic guest 4, in which multiple bisignated Cotton effects emerged in the absorption region of 1b, and which were substantially distinct from the original Cotton effects of (R,R,R)-1b itself (Fig. 10a). Also, we found a quite similar and significant change in the molar CDs when the guest was mixed with (R,R,R,R,R,R)-1c, which possesses the local point chirality in every blade (Fig. 10b). Molar CDs of 1b and 1c in the presence or absence of a ditopic guest are summarized in Table 1 along with those of 2. During complexation, we observed a hypsochromic shift from 368 to 357 nm for the absorption maximum of 1b (366 to 357 nm for 1c) in UV-vis spectroscopy, which indicated that blades relating to the longest diametrical π-conjugation were induced to prefer a twisting and/or strained conformation in the complex.7a,b,18,19 Thus, we considered that these complexation-induced spectral changes were the result of a chiroptical molecular propeller induced in the 1
:
3 complexes (R,R,R)-1b·43 and (R,R,R,R,R,R)-1c·43 (Scheme 2).
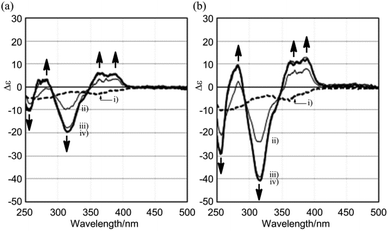 |
| Fig. 10 Continuous changes in the CD spectra of (a) (R,R,R)-1b (8.7 × 10−5 M) and (b) (R,R,R,R,R,R)-1c (8.1 × 10−5 M), upon complexation with achiral ditopic guest 4 [(i) 0 equiv. (1 only, dashed line), (ii) 3 equiv., (iii) 6 equiv., and (iv) 12 equiv. (solid lines)]. All spectra were measured in CH2Cl2 at room temperature. | |
Table 1 Molar CDs of 1, 2 and 3, in the presencea,b or absence of a ditopic guest, measured in CH2Cl2 at 293 K
λ
ext/nm (Δε) |
In the presence of 4 (4 equiv.).
In the presence of (R,R)-5 (4 equiv.).
|
(R,R,R)-1b |
358 (−3), 321 (−2), 269 (−5) |
(R,R,R,R,R,R)-1c |
362 (−6), 335 (−4), 268 (−10) |
(R,R,R)-1b |
386a (+6), 367 (+6), 315 (−20), 283 (+3), 254 (−10) |
(R,R,R,R,R,R)-1c |
389a (+12), 361 (+11), 316 (−41), 282 (+10), 256 (−29) |
|
(R)-2b |
344 (+1), 290 (−6) |
(R,R)-2c |
349 (+1), 287 (−10) |
2a
|
341b (+2), 286 (−6) |
(R)-2b |
331a (+5), 285 (−14) |
(R,R)-2c |
326a (+16), 283 (−40) |
|
(R)-3 |
291 (−5) |
Conclusions
We have demonstrated a method for constructing a chiroptical molecular propeller based on the HPEB framework. The important issue is how to force all of the blades to twist in a conrotatory manner and to prefer a particular sense of the propeller arrangements with (M)- or (P)-helicity. We focused on three pairs of neighboring blades and regarded the HPEB framework as a threefold double-bladed substructure. By pairing two blades, a local point chirality was transmitted to the double-bladed dynamic helicity and acted as a chiral handle to control the helical preference, while such a local chirality did not seem to exert any helical influence on the dynamic helicity by itself due to the high flexibility of each blade.
We found a helical conformation with neighboring blades twisting in a conrotatory manner with the attachment of a suitable tertiary amide to each of the blades. The tertiary amide nitrogen was modified with benzoyl and a series of alkyl substituents [Me, CH2(cHex), or (R)-CH*Me(cHex)]. The chiral auxiliary failed to induce a preference in dynamic helicity by itself (weak chiral communication between blades). The benzoyl groups in neighboring blades provided a binding site for capturing a ditopic guest through hydrogen bonds, to give a threefold supramolecular cyclic structure. The point chirality (R) acted as a chiral handle to control the propeller-shaped helicity only when HPEB was in a complexed state (complexation-induced chiral communication between blades), where two neighboring blades twisted in a conrotatory manner preferred a particular handedness, leading to a C3-symmetric chiroptical molecular propeller in the HPEB framework.
Notes and references
- K. Schlögl, W. Weissensteiner and M. Widhalm, J. Org. Chem., 1982, 47, 5025 CrossRef CAS; S. Liang, C.-H. Lee, S. I. Kozhushkov, D. S. Yufit, J. A. K. Howard, K. Meindl, S. Rühl, C. Yamamoto, Y. Okamoto, P. R. Schreiner, B. Christopher Rinderspacher and A. de Meijere, Chem. – Eur. J., 2005, 11, 2012 CrossRef PubMed; T. Benincori, G. Celentano, T. Pilati, A. Ponti, S. Rizzo and F. Sannicolò, Angew. Chem., Int. Ed., 2006, 45, 6193 CrossRef PubMed; H. Ito, T. Abe and K. Saigo, Angew. Chem., Int. Ed., 2011, 50, 7144 CrossRef PubMed; B. Driesschaert, R. Robiette, F. Lucaccioni, B. Gallez and J. Marchand-Brynaert, Chem. Commun., 2011, 47, 4793 RSC.
-
(a) K. Sakajiri, T. Sugisaki and K. Moriya, Chem. Commun., 2008, 3447 RSC;
(b) S. Nobusue, Y. Mukai, Y. Fukumoto, R. Umeda, K. Tahara, M. Sonoda and Y. Tobe, Chem. – Eur. J., 2012, 18, 12814 CrossRef CAS PubMed;
(c) J. C. J. Bart, Acta Crystallogr., Sect. B: Struct. Crystallogr. Cryst. Chem., 1968, 24, 1277 CrossRef CAS;
(d) Y. Rubin, Chimia, 1998, 52, 118 CAS;
(e) J. P. Bolender and F. S. Richardson, Biophys. Chem., 2003, 105, 293 CrossRef CAS;
(f) P. Axe, S. D. Bull, M. G. Davidson, M. D. Jones, D. E. J. E. Robinson, W. L. Mitchell and J. E. Warren, Dalton Trans., 2009, 10169 RSC;
(g) E. Gagnon, T. Maris and J. D. Wuest, Org. Lett., 2010, 12, 404 CrossRef CAS PubMed;
(h) A. Martinez, L. Guy and J.-P. Dutasta, J. Am. Chem. Soc., 2010, 132, 16733 CrossRef CAS PubMed;
(i) V. M. Tormyshev, A. M. Genaev, G. E. Sal'nikov, O. Y. Rogozhnikova, T. I. Troitskaya, D. V. Trukhin, V. I. Mamatyuk, D. S. Fadeev and H. J. Halpern, Eur. J. Org. Chem., 2012, 623 CrossRef CAS PubMed;
(j) S. Sathyamoorthi, J. T. Mague and R. A. Pascal Jr., Org. Lett., 2012, 14, 3427 CrossRef CAS PubMed.
- Recent reviews: M. Suginome, T. Yamamoto, Y. Nagata, T. Yamada and Y. Akai, Pure Appl. Chem., 2012, 84, 1759 CrossRef CAS; R. P. Megens and G. Roelfes, Chem. – Eur. J., 2011, 17, 8514 CrossRef PubMed; Y. Furusho and E. Yashima, Macromol. Rapid Commun., 2011, 32, 136 CrossRef PubMed; E. Yashima, K. Maeda, H. Iida, Y. Furusho and K. Nagai, Chem. Rev., 2009, 109, 6102 CrossRef PubMed.
- Recent reviews: M. Gingras, Chem. Soc. Rev., 2013, 42, 968 RSC; M. Gingras, G. Félix and R. Peresutti, Chem. Soc. Rev., 2013, 42, 1007 RSC; M. Gingras, Chem. Soc. Rev., 2013, 42, 1051 RSC; Y. Shen and C.-F. Chen, Chem. Rev., 2012, 112, 1463 CrossRef CAS PubMed.
- Y. Yang, Y. Zhang and Z. Wei, Adv. Mater., 2013, 25, 6039 CrossRef CAS PubMed; J. Crassous, Chem. Commun., 2012, 48, 9684 RSC; J. Clayden, Nat. Chem., 2011, 3, 842 CrossRef PubMed; S. Pieraccini, S. Masiero, A. Ferrarini and G. P. Spada, Chem. Soc. Rev., 2011, 40, 258 RSC; J. Clayden, Chem. Soc. Rev., 2009, 38, 817 RSC.
- R. Katoono, H. Kawai, K. Fujiwara and T. Suzuki, Chem. Commun., 2008, 4906 RSC.
-
(a) K. Sakajiri, T. Sugisaki, K. Moriya and S. Kutsumizu, Org. Biomol. Chem., 2009, 7, 3757 RSC;
(b) K. Kamada, L. Antonov, S. Yamada, K. Ohta, T. Yoshimura, K. Tahara, A. Inaba, M. Sonoda and Y. Tobe, ChemPhysChem, 2007, 8, 2671 CrossRef CAS PubMed;
(c) K. Kobayashi and N. Kobayashi, J. Org. Chem., 2004, 69, 2487 CrossRef CAS PubMed;
(d) P. Ehlers, A. Neubauer, S. Lochbrunner, A. Villinger and P. Langer, Org. Lett., 2011, 13, 1618 CrossRef CAS PubMed;
(e) B. Traber, J. J. Wolff, F. Rominger, T. Oeser, R. Gleiter, M. Goebel and R. Wortmann, Chem. – Eur. J., 2004, 10, 1227 CrossRef CAS PubMed;
(f) K. Kondo, S. Yasuda, T. Sakaguchi and M. Miya, J. Chem. Soc., Chem. Commun., 1995, 55 RSC;
(g) W.-Y. Chai, E.-Q. Yang, Y.-L. Zhang, A.-L. Xie and X.-P. Cao, Synthesis, 2012, 439 CAS.
- R. Katoono, H. Kawai, M. Ohkita, K. Fujiwara and T. Suzuki, Chem. Commun., 2013, 49, 10352 RSC.
- K. Sakajiri, H. Yoshida, K. Moriya and S. Kutsumizu, Chem. Lett., 2009, 38, 1066 CrossRef CAS; S. K. Varshney, H. Takezoe and D. S. S. Rao, Bull. Chem. Soc. Jpn., 2008, 81, 163 CrossRef; S.-C. Chien, H.-H. Chen, H.-C. Chen, Y.-L. Yang, H.-F. Hsu, T.-L. Shih and J.-J. Lee, Adv. Funct. Mater., 2007, 17, 1896 CrossRef PubMed; S. Kumar and S. K. Varshney, Angew. Chem., Int. Ed., 2000, 39, 3140 CrossRef; B. Kohne and K. Praefcke, Chimia, 1987, 41, 196 Search PubMed.
- E. L. Spitler, C. A. Johnson II and M. M. Haley, Chem. Rev., 2006, 106, 5344 CrossRef CAS PubMed; T. Yoshimura, A. Inaba, M. Sonoda, K. Tahara, Y. Tobe and R. V. Williams, Org. Lett., 2006, 8, 2933 CrossRef PubMed.
- R. Katoono, H. Kawai, K. Fujiwara and T. Suzuki, J. Am. Chem. Soc., 2009, 131, 16896 CrossRef CAS PubMed.
- Y.-H. Sohgawa, H. Fujimori, J. Shoji, Y. Furusho, N. Kihara and T. Takata, Chem. Lett., 2001, 30, 774 CrossRef.
- I. Azumaya, H. Kagechika, Y. Fujiwara, M. Itoh, K. Yamaguchi and K. Shudo, J. Am. Chem. Soc., 1991, 113, 2833 CrossRef CAS; L. Chabaud, J. Clayden, M. Helliwell, A. Page, J. Raftery and L. Vallverdú, Tetrahedron, 2010, 66, 6936 CrossRef PubMed.
-
(a) R. Katoono, K. Fujiwara and T. Suzuki, Chem. Commun., 2014, 50, 5438 RSC;
(b) J. S. Melinger, Y. Pan, V. D. Kleiman, Z. Peng, B. L. Davis, D. McMorrow and M. Lu, J. Am. Chem. Soc., 2002, 124, 12002 CrossRef CAS PubMed;
(c) S. Samori, S. Tojo, M. Fujitsuka, T. Ryhding, A. G. Fix, B. M. Armstrong, M. M. Haley and T. Majima, J. Org. Chem., 2009, 74, 3776 CrossRef CAS PubMed;
(d) R. H. Grubbs and D. Kratz, Chem. Ber., 1993, 126, 149 CrossRef CAS PubMed.
- Almost the same helical structure was found for a model substructure (R)-2b′ [X = Me, Y = (R)-C*HMe(cHex)], which indicated that a double-bladed substructure seems to prefer the helical conformation in solution as well as in the crystal form, regardless of the bulkiness of an auxiliary on the amide nitrogen: a helical conformation with (P)-helicity was the most energy-minimized structure (Fig. S2a†), and interestingly, another helical conformation with inversed helicity was found at a slightly higher energy level (+2.78 kJ mol−1) (Fig. S2b†). This small difference in energy between diastereomers with (P)- or (M)-helicity might reflect weak chiral communication between blades in the absence of a guest, as shown by small positively-signed Cotton effects around at 350 nm (Δε < +1) in the CD spectra of (R)-2b and (R,R)-2c. Thus, we concluded that these small effects around at 350 nm were a part of bisignated Cotton effects due to a slightly-preferred sense of the double-bladed dynamic helicity.
- Upon complexation, changes in absorption regarding the longer conjugation (>300 nm) were also induced (Fig. S3b†), and reflected some changes in conformation of the host in a supramolecular cyclic structure.
- Such a geometry was often seen in crystals of HPEB derivatives,7e,g in addition to a propeller arrangement.2b,7d.
- J. A. Marsden, J. J. Miller, L. D. Shirtcliff and M. M. Haley, J. Am. Chem. Soc., 2005, 127, 2464 CrossRef CAS PubMed.
- Upon complexation of 1a (7.2 × 10−5 M) with (R,R)-5 (12 equiv.), we observed a hypsochromic shift in the absorption of 1a (367 to 364 nm) similar to those induced for (R,R,R)-1b or (R,R,R,R,R,R)-1c, while in the CD spectra no significant change was induced. These results indicated that 1a [X = Y = Me] formed a complex with (R,R)-5 through hydrogen bonds, however any preference for a particular sense was not induced.
Footnote |
† Electronic supplementary information (ESI) available: Supplementary figures, and experimental details of new compound preparation and X-ray analysis. CCDC 1011177 and 1011992. For ESI and crystallographic data in CIF or other electronic format see DOI: 10.1039/c4ob01601g |
|
This journal is © The Royal Society of Chemistry 2014 |
Click here to see how this site uses Cookies. View our privacy policy here.