Enhanced catalysis and enantioselective resolution of racemic naproxen methyl ester by lipase encapsulated within iron oxide nanoparticles coated with calix[8]arene valeric acid complexes
Received
21st May 2014
, Accepted 1st July 2014
First published on 2nd July 2014
Abstract
In this study, two types of nanoparticles have been used as additives for the encapsulation of Candida rugosa lipase via the sol–gel method. In one case, the nanoparticles were covalently linked with a new synthesized calix[8]arene octa valeric acid derivative (C[8]-C4-COOH) to produce new calix[8]arene-adorned magnetite nanoparticles (NP-C[8]-C4-COOH), and then NP-C[8]-C4-COOH was used as an additive in the sol–gel encapsulation process. In the other case, iron oxide nanoparticles were directly added into the sol–gel encapsulation process in order to interact electrostatically with both C[8]-C4-COOH and Candida rugosa lipase. The catalytic activities and enantioselectivities of two novel encapsulated lipases (Enc-NP-C[8]-C4-COOH and Enc-C[8]-C4-COOH@Fe3O4) in the hydrolysis reaction of racemic naproxen methyl ester were evaluated. The results showed that the activity and enantioselectivity of the lipase were improved when the lipase was encapsulated in the presence of calixarene-based additives. Indeed, the encapsulated lipases have an excellent rate of enantioselectivity, with E = 371 and 265, respectively, as compared to the free enzyme (E = 137). The lipases encapsulated with C[8]-C4-COOH and iron oxide nanoparticles (Enc-C[8]-C4-COOH@Fe3O4) retained more than 86% of their initial activities after 5 repeated uses and 92% with NP-C[8]-C4-COOH.
Introduction
Researchers have developed various methods involving enzymatic and non-enzymatic catalysts to enrich a derivative for one of the enantiomers from the reaction product.1–9 Biocatalysis has been applied as a viable and preferred technique in organic synthesis for the production of enantiopure compounds, particularly for pharmaceutical compounds.10,11Candida rugosa lipase has a wide range of natural substrates and is thus commonly chosen as a biocatalyst.12 Moreover, lipases are usually used as aqueous solutions, which makes their recovery and reuse problematic and can also result in contamination of the product.13 In an attempt to enhance the activity and enantioselectivity of C. rugosa, researchers have tried immobilizing C. rugosa using various types of carriers such as celite, kaolin, cyclodextrin, amberlite XAD 7, sporopollenin, chitosan, and calixarene.14–16
Recently, immobilizing the lipase using calixarenes has become a common way of increasing the lipase activity and enantioselectivity.12,17,18 Calixarenes are used to this end because they are strong building blocks and maintain functionalization at both lower-rim and upper-rim positions.19–24
Over the past few years, scientists have grafted certain calixarene derivatives onto the surface of silica-modified-iron oxide nanoparticles in order to provide easier separation and more reusable processes. Furthermore, in order to improve the activity and enantioselectivity of lipases in the hydrolysis reaction of racemic naproxen methyl ester, some calixarene-grafted magnetite nanoparticles have successfully immobilized the lipase via the sol–gel method, which opens up a wide range of opportunities for future research.14,25
In the present study, p-tert-butylcalix[8]arene was substituted with valeric acid, and selectively grafted onto aminosilica-modified magnetite nanoparticles to afford the corresponding calix[8]arene-hepta acid-immobilized magnetite nanoparticles (NP-C[8]-C4-COOH). The C. rugosa lipase was encapsulated within a sol–gel system8,9,12,14,17,18 formed through polycondensation with tetraethoxysilane (TEOS) and octyltriethoxysilane (OTES) in the presence or absence of octa valeric acid functionalized calix[8]arene (C[8]-C4-COOH) with magnetite nanoparticles to afford Enc-C[8]-C4-COOH@Fe3O4. Moreover, NP-C[8]-C4-COOH was also employed as an additive for the encapsulation of C. rugosa lipase to produce Enc-C[8]-C4-COOH. The activity and enantioselectivity of the encapsulated lipases were also evaluated under different conditions such as temperature and pH influences. The magnetite properties of these encapsulated lipases with nanoparticles provide easy separation with respect to reducing the labor force, as well.
Results and discussion
Synthesis of calixarene molecules
In our previous study,26 we prepared an octa-acid derivative of p-tert-butylcalix[8]arene (6),33 and examined its usability as an additive in the encapsulation of lipases via the sol–gel process.1 To obtain 6, p-tert-butylcalix[8]arene was treated with methylbromo acetate to synthesize its octa ester derivative (5). Upon hydrolysis, 5 yielded an octa-acid derivative of p-tert-butylcalix[8]arene (6)26 (Scheme 1). The focus of the current study was to prepare two novel encapsulated lipases (Enc-NP-C[8]-C4-COOH and Enc-C[8]-C4-COOH@Fe3O4) in order to evaluate their catalytic and enantioselective properties. To this end, p-tert-butylcalix[8]arene was initially treated with 5-bromovalerate to afford the octa-valerate derivative C[8]-C4-COOEt (2). The structure of C[8]-C4-COOEt was confirmed not only by the appearance of a new vibration band at 1732 cm−1, which is the carbonyl group of the ester derivative (2) on the FTIR spectrum, but also by the appearance of the peak at 1.11 ppm (24H, –CH3) and the peaks of –CH2 which came from the valerate groups on the 1H-NMR spectrum. The octa-valeric acid derivatives of p-tert-butylcalix[8]arene (C[8]-C4-COOH) (3) having a longer alkyl chain than 6 were synthesized by hydrolysis of 2 with an aqueous solution of NaOH for the first time (Scheme 1). The FTIR spectrum of C[8]-C4-COOH clearly shows that the ester carbonyl vibration shifted to an acid carbonyl vibration band at 1704 cm−1 (Fig. 1). The 1H-NMR spectrum of C[8]-C4-COOH also proves the structure of 3 by disappearance of the –CH3 peak of C[8]-C4-COOEt.
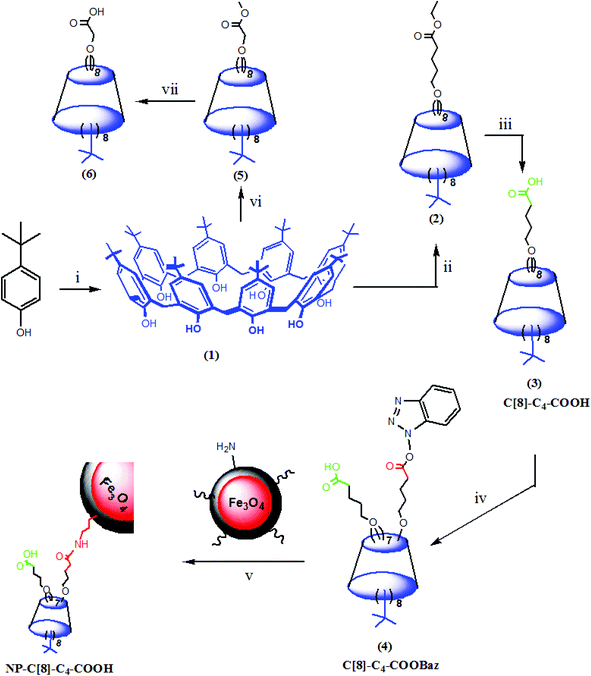 |
| Scheme 1 Preparation of C[8]-C4-COOH and NP-C[8]-C4-COOH. Reaction conditions: (i) paraformaldehyde, NaOH; (ii) K2CO3, NaI, 5-bromovalerate; (iii) 15% NaOH solution; (iv) 1-hydroxybenzotriazole, DCC; (v) Fe3O4-APTES; (vi) methylbromoacetate, K2CO3; (vii) 15% NaOH solution. | |
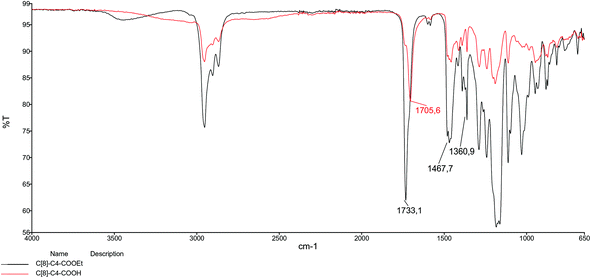 |
| Fig. 1 FTIR spectra of C[8]-C4-COOEt and C[8]-C4-COOH. | |
One acid moiety of C[8]-C4-COOH (3) was selectively treated with 1-hydroxybenzotriazol to afford the corresponding 5,11,17,23,29,35,41,47-octa-tert-butyl-49-mono-(benzotriazol-1)-oxycarbonylbutoxy-50,51,52,53,54,55,56-hepta-hydroxycarbonyl butoxy-calix[8]arene (C[8]-C4-COOBaz) (4) (Scheme 1). The 1H-NMR spectra of 4 confirmed exclusive functionalization by giving the expected splitting pattern (two doublets at 7.69 and 7.96 ppm and two triplets at 7.39 and 7.52 ppm for 1 integral ratio proton of ArH of the benzotriazole unit). Subsequently, C[8]-C4-COOBaz was grafted onto the aminosilica-modified Fe3O4 nanoparticles (Fe3O4-APTES), which were prepared according to the literature,27 in order to produce NP-C[8]-C4-COOH (Scheme 1). NP-C[8]-C4-COOH was then used as an additive in the encapsulation of the lipase. FTIR spectra were used to elaborate the structure of NP-C[8]-C4-COOH (Fig. 2). The characteristic peaks of NP-C[8]-C4-COOH appeared at 1641 (COONa) and 1621 cm−1 (COONH). Additional peaks centered at 1542, 1468, 1413 and 1363 cm−1, which are stretching vibrations of the aromatic C
C bonds of the calix[8]arene derivative. Moreover, the FTIR spectrum also shows peaks of the magnetite nanoparticles at 1149, 1046, 957 and 790 cm−1 for the Si–O group and at 578 cm−1 for the Fe–O group (see Fig. 2).
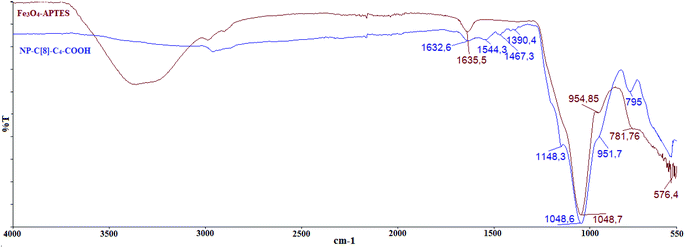 |
| Fig. 2 FTIR spectra of Fe3O4-APTES and NP-C[8]-C4-COOH. | |
Transmission electron microscopy (TEM) analysis was used to obtain more information about particle size and morphology (see Fig. 3) of Fe3O4-nanoparticles (Fig. 3a), and NP-C[8]-C4-COOH (Fig. 3b). TEM images of Fe3O4-nanoparticles are observed as intensive aggregates due to the lack of any repulsive force between the magnetite nanoparticles, which is due to a single magnetic crystallite and the uniform nano-size of the Fe3O4, which has a typical size range of 8 ± 3 nm and is surrounded by a silica-based material and calixarene units that are about 19 ± 5 nm thick. Using calix[8]arene derivative immobilization, the dispersion of particles was improved greatly (Fig. 3b) due to the production of an electrostatic repulsion force and steric hindrance between the calix[8]arene and the surface of the Fe3O4 nanoparticles.
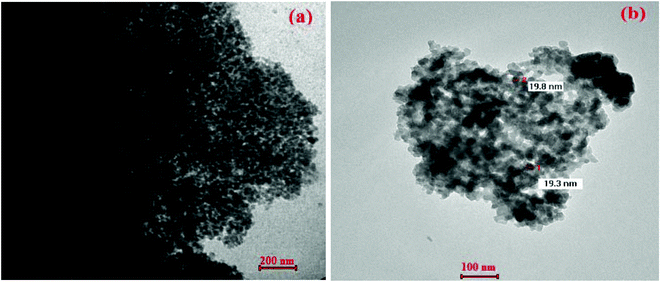 |
| Fig. 3 TEM micrographs of (a) Fe3O4-nanoparticles and (b) NP-C[8]-C4-COOH. | |
Thermogravimetric analysis (TGA) was used to determine the amount of C[8]-C4-COOH (Fig. 4) on the aminosilica-modified Fe3O4 nanoparticles (Fe3O4-APTES). As depicted in Fig. 4, the TGA curve of NP-C[8]-C4-COOH reveals that the weight loss of 30% mass was due to the decomposition of C[8]-C4-COOH and the 3-aminopropyltrimethoxysilane groups in the range of 325–625 °C.
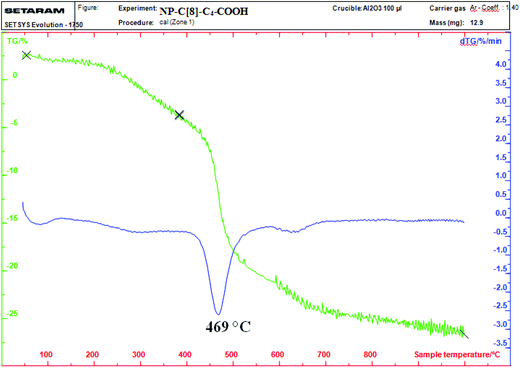 |
| Fig. 4 TGA curves of NP-C[8]-C4-COOH. | |
Sol–gel procedure for the encapsulation of C. rugosa lipase on additives
In our previous study, calix[8]arene octa acid (6) was used as an additive in the sol–gel encapsulation process of lipases in order to estimate its catalytic ability in the enantioselective hydrolysis of racemic naproxen methyl ester.26 It was found that the activity and enantioselectivity of the encapsulated lipase with 6 were extremely high when compared with the affinity of the encapsulated lipase without any additives.26 This increase in activity and enantioselectivity of the lipase was attributed to a complex between the –COOH groups of the calix[8]arene derivative and the lysine moieties of the enzymes as well as host–guest interactions and the cooperative affinities of calix[8]arene derivatives.18,26 The present study aimed to extend the number of calix[8]arene-based additives and compare their catalytic abilities. Furthermore, either a calix[8]arene octa valeric acid derivative (C[8]-C4-COOH) and Fe3O4-nanoparticles or hepta valeric acid-substituted calix[8]arene-grafted iron oxide nanoparticles (NP-C[8]-C4-COOH) were employed as additives for the encapsulation of the lipase (see Fig. 5). A sol–gel procedure was used to encapsulate the lipase with or without additives, which provided mechanical entrapment of the enzyme according to the published procedure.9,14,17 The Bradford method regarding bovine serum albumin was used as a standard to determine the amount of protein in the solution and in the elution solute.28 The changes in activity of the encapsulated lipases were determined according to the literature.29,30
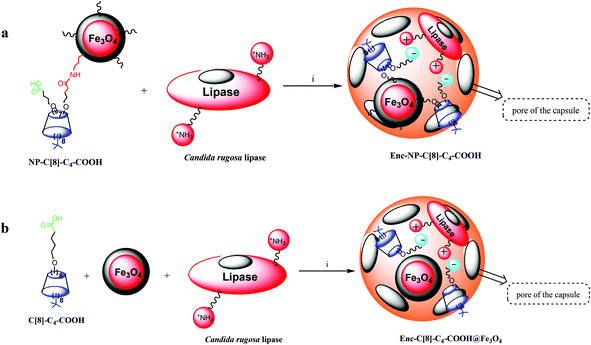 |
| Fig. 5 Preparation of encapsulated lipases. Reaction conditions for (a and b); (i) phosphate buffer (pH 7.0), polyvinyl alcohol, NaF, isopropyl alcohol, tetramethoxysilane, octyltri-methoxysilane. | |
Table 1 reveals the results of the initial attempt to associate the catalytic activity of the encapsulated lipases in the presence/absence of C[8]-COOH, Fe3O4-nanoparticles, and Enc-C[8]-C4-COOH@Fe3O4. As seen in Table 1, in terms of catalytic activity and enantioselectivity, the encapsulated lipase in the presence of C[8]-COOH (6) did not show higher affinity than the encapsulated lipase (Enc-C[8]-C4-COOH@Fe3O4). However, the encapsulated lipase with C[8]-COOH (6) was more efficient than the encapsulated lipase (Enc-Lipase) in the absence of additives (see Table 1). Moreover, in order to determine the role of the Fe3O4-nanoparticles in this reaction, the lipase was encapsulated in the presence of only Fe3O4-nanoparticles as an additive. However, the encapsulated lipase with iron oxide nanoparticles exhibited less activity (see Table 1). These findings clearly suggest that both octa COOH-substituted calix[8]arene derivatives represent complexibility toward the lipase such as host–guest, hydrogen bonding, and ionic interactions that might increase the activity and stability of the lipase.
Table 1 Enantioselective hydrolysis of racemic naproxen methyl ester and activity of the encapsulated lipases under optimum reaction conditions
Lipase |
Protein loading (mg g−1-sol gel) |
Lipase activity(Ug−1 sol gel) |
Specific activity(U mg−1 protein) |
Conversion (x, %) |
eep (%) |
E
|
Candida rugosa lipase encapsulated without calixarene or magnetite nanoparticles.
Candida rugosa lipase encapsulated with C[8]-COOH.35
Candida rugosa lipase encapsulated with C[6]-COOH.35
Candida rugosa lipase encapsulated with C[4]-COOH.35
Candida rugosa lipase encapsulated with dibenzo-18-crown-6.17
Candida rugosa lipase encapsulated with NP.9
Candida rugosa lipase encapsulated with C[8]-C4-COOH and iron oxide nanoparticles.
Candida rugosa lipase encapsulated with NP-C[8]-C4-COOH.
|
Enc-lipase
|
28.5 |
95 |
3.3 |
20 |
>98 |
137 |
Enc-C[8]-COOH
|
26.8 |
37 |
1.4 |
30 |
>98 |
150 |
Enc-C[6]-COOH
|
27.9 |
44 |
1.6 |
27 |
>98 |
141 |
Enc-C[4]COOH
|
28.5 |
28 |
1.0 |
43 |
>98 |
224 |
Enc-Dibenzo-18-crown-6
|
18.3 |
25 |
1.4 |
33 |
>98 |
193 |
Enc-NP
|
33.0 |
102 |
3.1 |
30.7 |
>98 |
170 |
Enc-C[8]-C4-COOH@Fe3O4
|
22.0 |
35 |
1.6 |
49 |
>98 |
371
|
Enc-NP-C[8]-C4-COOH
|
15.5 |
43 |
2.8 |
46 |
>98 |
265
|
Table 1 also indicates the catalytic activity results of the encapsulated lipase in the presence of NP-C[8]-C4-COOH. The encapsulated lipase (Enc-NP-C[8]-C4-COOH) provided high catalytic activity and enantioselectivity as compared to the Enc-Lipase and Enc-NP.
Effect of pH and temperature on the lipase activity
The hydrolysis of p-NPP by the encapsulated lipases was examined to assess their catalytic activity at various pHs (4.0–9.0). Finding an optimum pH for the encapsulated lipases is the optimal point, since it is well known that the conformational change of the enzyme results in a different catalytic activity when the pH of the media is changed. With that in mind, the various pHs were altered so that the encapsulated lipases would show the most efficient hydrolysis behaviour towards p-NPP. It was found that both free and encapsulated (C[8]-COOH, C[8]-C4-COOH) demonstrated high hydrolysis capability at pH 7.0, whereas the obtained efficient hydrolysis of p-NPP was observed to be at pH 6.0 for the encapsulated lipase in the presence of NP-C[8]-C4-COOH (see Fig. 6a).
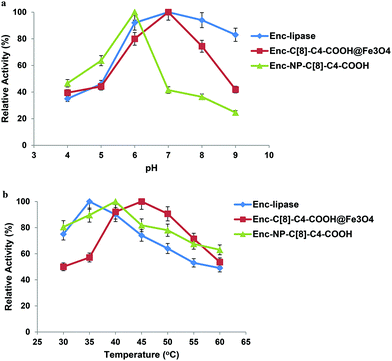 |
| Fig. 6 (a) Effect of substrate pH on residual activity of the encapsulated lipases; (b) effect of reaction temperature on the residual activity of the encapsulated lipases. Averages and standard deviations calculated for data received from three independent extraction experiments. | |
To calculate the affinity of the encapsulated lipase (Enc-Lipase) without calixarene derivatives or magnetite nanoparticles, as well as the affinity of lipase-encapsulated calixarene derivatives with temperature, the reaction was carried out under various temperatures (30–60 °C) at pH 7.0 (Fig. 6b). It was observed that encapsulated lipases with C[8]-C4-COOH or magnetite nanoparticles showed high activity at 45 °C, whereas the highest activity of encapsulated lipases without additives was at 35 °C. The highest percentage depending upon the activity of Enc-NP-C[8]-C4-COOH was at 40 °C (see Fig. 6b). Immobilization shifted the optimum temperature from 35 °C for the free lipase to around 40–45 °C, due to either conformational limitations on enzyme movement as a result of multipoint interaction between the enzyme and the support or improved substrate diffusion at a high temperature. One of the main reasons for enzyme immobilization is the expected increase in stability toward various deactivating forces, due to the limited conformational mobility of the molecules after immobilization.9,13,32
Enantioselective hydrolysis of racemic naproxen methyl ester
Variations in pH and temperature can influence the conformation of the enzyme.31 In an effort to visualise the effects of these parameters on the activity of the encapsulated lipase, we carried out the hydrolysis reaction of (R,S)-naproxen methyl ester at a pH range of 5–7 (see Fig. 7a) and at temperatures of 35 and 40 °C (see Fig. 7b).
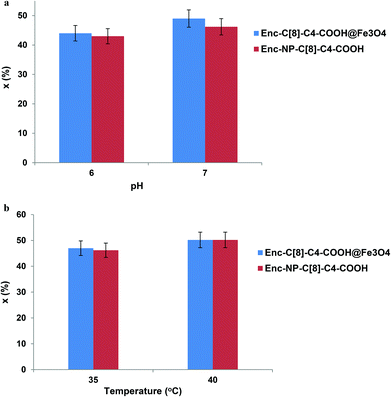 |
| Fig. 7 Effect of pH (a) and temperature (b) on the conversion (x) in the hydrolysis of racemic naproxen methyl ester. Averages and standard deviations calculated for data received from three independent extraction experiments. | |
In an earlier study, the interaction of encapsulated lipases with octa acid derivatives of calix[8]arene (6) via the sol–gel encapsulation method was employed as a catalyst for the enantioselective hydrolysis of the racemic naproxen methyl ester.26 In order to understand and expand the range of encapsulated lipases available as enantioselective catalysts, in the present study, we describe two new encapsulated lipases (Enc-NP-C[8]-C4-COOH and Enc-C[8]-C4-COOH@Fe3O4). In describing these new encapsulated lipases, we also aim to evaluate their chiral catalytic affinities.
The hydrolysis reaction results of (R,S)-naproxen methyl esters by the encapsulated lipases are shown in Table 1. After 24 h treatment of the encapsulated lipases with racemic naproxen methyl ester in aqueous buffer solution and isooctane, the lipases produced R-naproxen methyl ester and the corresponding acid (eep) >98%, the percentage of conversion (x) 49.0 for Enc-C[8]-C4-COOH@Fe3O4 and 46.0 for Enc-NP-C[8]-C4-COOH. The treatment also resulted in enantioselectivities toward naproxen methyl ester (E value) of 371 for Enc-C[8]-C4-COOH@Fe3O4 and 265 for Enc-NP-C[8]-C4-COOH, as compared to an E value of 137 for the encapsulated lipase without additives (Enc-lipase). These results show strong evidence that the immobilization of lipases with calixarene derivatives led to high stereoselectivity, high conversion, and fast recovery of the catalyst owing to the magnetite properties of the encapsulated lipases (Enc-C[8]-C4-COOH@Fe3O4 and/or Enc-NP-C[8]-C4-COOH). This is not a surprising result, considering not only the highly effective complexing agent properties of the free –COOH groups of C[8]-C4-COOH and NP-C[8]-C4-COOH by means of forming salt bridges with lysine groups of lipase, but also the host–guest interaction ability of calix[8]arene. A considerable increase in the activity and enantioselectivity of the encapsulated lipase in the presence of additives has also been observed in the literature.8,14,26,32
Increasing the recovery and reusability of the enzyme is essential for economical usage. In this sense, the encapsulated lipases with their unique magnetite properties should be paid much attention because of their easy separability with a simple magnet. Fig. 8 shows that, even after the 5th reuse cycle, the encapsulated lipases still retained 42% of their conversion ratios for Enc-C[8]-C4-COOH@Fe3O4 and 42.4% for Enc-NP-C[8]-C4-COOH.
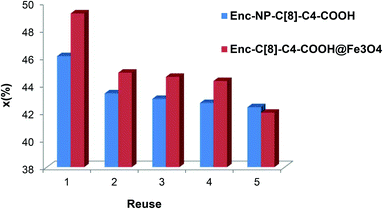 |
| Fig. 8 Reusability on the conversion (x) in the hydrolysis of racemic naproxen methyl ester. | |
Conclusion
We have synthesized a new p-tert-butylcalix[8]arene derivative (C[8]-C4-COOH) and grafted it onto iron oxide nanoparticles to afford NP-C[8]-C4-COOH. Both C[8]-C4-COOH and Fe3O4-nanoparticles were used as additives for the encapsulation of lipase. Moreover, NP-C[8]-C4-COOH was also used as an additive for the lipase encapsulation in order to form a salt bridge between lysine moieties of the enzyme and free COOH groups of calix[8]arene derivatives, as well as to provide an easy way out for the separation processes. Two new encapsulated lipases (Enc-C[8]-C4-COOH@Fe3O4 and Enc-NP-C[8]-C4-COOH) were examined for the enantioselective hydrolysis of (R,S)-naproxen methyl ester. In addition, the effects of some parameters such as pH and temperature were also investigated. It was found that the enantioselectivity of (R,S)-naproxen methyl ester improved more with Enc-C[8]-C4-COOH@Fe3O4 and Enc-NP-C[8]-C4-COOH than with the encapsulated lipase (Enc-lipase), with E values of 371 and 265, respectively. These findings demonstrate that the octa valeric acid-substituted calix[8]arene derivatives are useful supports for lipase encapsulation. Hence, an approach is opened for developing a new technique to regulate enantioselective studies. Moreover, due to the low price of sol–gel encapsulation, the excellent performance of the lipase-immobilization, and its ready recyclability, the method is industrially workable.
Experimental
Reagents
DC Alufolien Kieselgel 60 F254 (Merck) was used for TLC analysis. All chemical reagents and starting materials, and all solvents were purchased from Aldrich, Fluka and Merck. HPLC grade organic solvents were used as the mobile phase without further purification or drying. A Millipore milli-Q Plus water purification system was used to receive deionized water. Candida rugosa, Bradford reagent, bovine serum albumin (99%), and p-nitrophenyl palmitate (p-NPP) were bought from Sigma Chemical Co. (St. Louis, MO).
Apparatus
A Varian 400 MHz spectrometer was used for NMR applications. FTIR spectra were evaluated on a Perkin Elmer spectrum 100 FTIR spectrometer. A Shimadzu 160A UV-visible recording spectrophotometer was used for UV-vis spectra. Thermogravimetric analysis (TGA) was carried out with a Seteram Evolution-1750 thermogravimetric analyzer. It was performed under an argon atmosphere. Transmission electron microscopy (TEM) analysis was carried out with FEI Tecnai G2 Spirit. Melting points were determined on an EZ-Melt apparatus in a sealed capillary. An Orion 410A+ pH meter was used for the pH measurements. High-performance liquid chromatography (HPLC) (Agilent 1200 Series) was carried out using a 1200 model quaternary pump, a G1315Bmodel diode array and multiple wavelength UV-vis detector, a 1200 model standard and preparative autosampler, a G1316A model thermostated column compartment, a 1200 model vacuum degasser, and an Agilent Chemstation B.02.01-SR2 Tatch data processor. The concentrations of S- and R-enantiomers of naproxen methyl ester were measured by HPLC (Agilent 1200 Series) using a Chiralcel OD-H column at a temperature of 25 °C. In the analysis, n-hexane–2-propanol–trifluoro acetic acid (100/1/0.1, v/v/v) was used as the mobile phase at a flow rate of 1 mL min−1; and UV detection was done at 254 nm.
Synthesis
p-tert-Butylcalix[8]arene (1), Fe3O4 nanoparticles (3) and aminosilica-modified Fe3O4 nanoparticles (Fe3O4-APTES) were synthesized according to the literature.14,27,33 The syntheses of octa ester derivatives of p-tert-butylcalix[8]arene (2), 5,11,17,23,29,35,41,47-octa-tert-butyl-49,50,51,52,53,54,55,56-octahydroxycarbonylbutoxy-calix[8]arene (3), 5,11,17,23,29,35,41,47-octa-tert-butyl-49-mono-(benzotriazol-1)-oxycarbonylbutoxy-50,51,52,53,54,55,56-heptahydroxycarbonylbutoxy-calix[8]arene (C[8]-C4-COOBaz) (4), and immobilization of C[8]-C4-COOBaz onto aminosilica-modified iron oxide nanoparticles (NP-C[8]-C4-COOH) are reported for the first time.
Synthesis of 5,11,17,23,29,35,41,47-octa-tert-butyl-49,50,51,52,53,54,55,56-octaethoxycarbonylbutoxy-calix[8]arene (C[8]-C4-COOEt) (2).
A mixture of p-tert-butylcalix[8]arene (1 g, 0.771 mmol), K2CO3 (3.41 g, 24.672 mmol), NaI (1.85 g, 12.336 mmol) and 5-bromovalerate (24.672 mmol) in 90 mL of acetone was heated at 80 °C. The reaction was monitored using TLC. After 5 days, the reaction was cooled to room temperature, filtered off, and the filtrate was evaporated to dryness. The remaining solid was dissolved in 100 mL Et2O and washed with water to adjust to pH 7.0. The organic phase was dried over MgSO4 and then filtered. The filtrate was evaporated under reduced pressure. The crude residue was recrystallized from MeOH. Yield 78.2%, M.p. 165–166 °C. FTIR (ATR): 1732 cm−1(C
O). 1H NMR (CDCl3): δ 1.05 (s, 72H, But), 1.11 (t, 24H, J = 6.8 Hz, –CH3), 1.72–1.76 (m, 32H, –CH2–), 2.27 (t, 16H, J = 6.8 Hz, –CH2–CO), 3.63 (brs, 16H, ArCH2–Ar), 3.99–4.04 (m, 32H, ArO–CH2– and O–CH2–), 6.92 (s, 16H, ArH). 13C-NMR (100 MHz, CDCl3): δ (ppm) 14.21 (–CH3), 21.62 (–CH2), 29.66 (–CH2), 31.26 (–CH3 of But), 31.39 (Ar–CH2–Ar), 31.59 (–C(CH3)3), 34.10 (–CH2), 60.15 (O–CH2–CH3), 73.40 (O–CH2), 125.90 (ArC), 132.95 (ArC), 145.83 (ArC), 150.58 (ArC–O), 173.41 (C
O). Anal. Calcd for C144H208O24: C, 74.45; H, 9.02. Found (%); C, 74.51; H, 8.98.
Synthesis of 5,11,17,23,29,35,41,47-octa-tert-butyl-49,50,51,52,53,54,55,56-octahydroxycarbonylbutoxy-calix[8]arene (C[8]-C4-COOH) (3).
A solution of NaOH (15%) was added to a mixture of C[8]-C4-COOEt (0.5 g, 0.43 mmol) in 50 mL EtOH. The reaction mixture was refluxed for 17 h. Then, the volatile component was evaporated, and the remaining solid was treated with cold water (50 mL) and HCl (3 N, 100 mL). The collected product was neutralized with water, and dried in an oven. Yield 88.5%; m.p. 294–296 °C. FTIR: 1704 cm−1 (C
O, acid). 1H-NMR (DMSO-d6): δ 0.94 (s, 72H, But), 1.60–1.65 (m, 32H, –CH2–), 2.19 (t, 16H, J = 6.8 Hz, –CH2–CO), 3.36–3.61 (m, 16H, Ar–CH2–Ar), 3.93 (brs, 16H, ArO–CH2–), 6.83 (s, 16H, ArH). 13C-NMR (100 MHz, DMSO-d6): δ (ppm) 22.18 (–CH2), 29.52 (–CH2), 29.88 (–CH3), 31.51 (Ar–CH2–Ar), 34.15 (–C(CH3)3), 34.67 (–CH2), 72.82 (O–CH2), 125.61 (ArC), 133.03 (ArC), 145.28 (ArC), 153.24 (ArC–O), 175.30 (C
O). Anal. Calcd for C128H176O24: C, 73.25; H, 8.45. Found (%); C, 73.17; H, 8.48.
Synthesis of 5,11,17,23,29,35,41,47-octa-tert-butyl-49-mono-(benzotriazol-1)-oxycarbonylbutoxy-50,51,52,53,54,55,56-heptahydroxycarbonylbutoxy-calix[8]arene (C[8]-C4-COOBaz) (4).
To a solution of C[8]-C4-COOH (0.3 g, 0.286 mmol) and 1-hydroxybenzotriazol (0.046 g, 0.34 mmol) in 25 mL THF was added DCC (0.071 g, 0.34 mmol), and then allowed to be stirred at rt for 48 h. The reaction was monitored using TLC. The solvent was then evaporated, and the residue was treated with EtOAc. The filtrate was evaporated to afford the final product. Yield 92%, 1H NMR (DMSO): δ 0.94–1.03 (m, 72H, But), 1.57–1.71 (m, 34H, –CH2– and –CH2–CO), 2.16–2.19 (m, 14H, –CH2–CO), 3.29–3.58 (m, 16H, ArCH2–Ar), 3.93 (brs, 16H, ArO–CH2–), 6.84–6.99 (m, 16H, ArH), 7.39 (t, 1H, J = 8.0 Hz, ArH), 7.52 (t, 1H, J = 7.2 Hz, ArH), 7.69 (d, 1H, J = 8.8 Hz, ArH), 7.96 (d, 1H, J = 8.8 Hz, ArH). Anal. Calcd for C134H179N3O24: C, 72.63; H, 8.14; N, 1.90. Found (%); C, 72.69; H, 7.94; N, 1.92.
Preparation of NP-C[8]-C4-COOH
A mixture of C[8]-C4-COOBaz (0.25 g) and Fe3O4-APTES (0.2 g) in 40 mL THF–DMF (v/v) was stirred at rt for 4 days. Then, NP-C[8]-C4-COOH was collected using a simple magnet, and washed with THF, EtOH and H2O, respectively. The product was dried in a vacuum oven. FTIR (KBr, cm−1); 1641 (COONa), 1621 (COONH), 1542, 1468, 1413 and 1363 (C
C), 1149, 1046, 957 and 790 (Si–O), and 578 (Fe–O).
Sol–gel encapsulation of lipase with/without C[8]-C4-COOH and magnetite nanoparticles or NP-C[8]-C4-COOH
The method of Reetz was modified for sol–gel encapsulation of the lipases.1 Typically, a mixture of lipase powder (lyophilizate) such as CRL-type VII (245 mg) in phosphate buffer (1.56 mL, 50 mM) adjusted at pH 7.0 was vigorously shaken. Either NP-C[8]-C4-COOH (0.2 g) or C[8]-C4-COOH (3) (0.1 g) and Fe3O4 (0.1 g) was added to the mixture, together with 400 μL of polyvinyl alcohol (4% w/v), 200 μL of NaF solution (0.1 M) and 400 μL of isopropyl alcohol. After obtaining homogeneity, tetramethoxysilane (460 μL, 0.5 mmol) and octyltrimethoxysilane (3.2 mL, 2.5 mmol) were added and left to be shaken for 10–15 s. Then, 15 mL of isopropyl alcohol was poured onto the dried white solid. The gel was washed with water (10 mL) and isopropyl alcohol (10 mL), and then it was lyophilized to produce the encapsulated lipases.
Lipase activity and protein assay determination
p-NPP in an aqueous phosphate buffer (100 mmol sodium phosphate, pH 7.0) was used to determine the hydrolytic activities of the encapsulated lipases. A UV-visible spectrophotometer was scanned at 410 nm in order to measure the concentration of the corresponding p-nitrophenol.29,30
Protein content was defined by the method of Bradford28 using a Bio-Rad protein dye reagent concentrate. Bovine serum albumin was used as the standard.
pHs and temperatures on activity
Activity was determined between pH 4 and 9 in 50 mM phosphate buffer to see the changes in activity of free and immobilized lipases.28–30 Moreover, the thermal inactivation of the free and immobilized lipases was investigated at 30–60 °C. Both forms of enzymes were incubated in PBS (50 mM, pH 7.0) for 20 min at various temperatures and, after lowering the temperature, the remaining activity was assayed under the standard conditions and analyzed.
Thermal stability
Each encapsulated lipase (with or without additives) was stored in the buffer (50 mM, pH 7.0) at 60 °C for 2 h, in order to estimate their activity as outlined above.
Enantioselective hydrolysis of racemic naproxen methyl ester by encapsulated lipases
A reaction system in an aqueous phase/organic solvent was used for the hydrolysis reactions according to the literature procedure.8,12,14 The conversion and enantioselectivity of naproxen methyl ester by the encapsulated lipases in the presence or absence of additives such as either C[8]-C4-COOH (3) and Fe3O4, or NP-C[8]-C4-COOH were expressed as the enantiomeric ratio (E), which was calculated from the conversion (x) and the enantiomeric excess of the substrate (ees) and the product (eep) using HPLC.34
E, ees, eep, x, CR and CS stand for enantiomeric ratio for irreversible reactions, enantiomeric excess of substrate, enantiomeric excess of the product, racemate conversion, concentration of R-enantiomer and concentration of S-enantiomer, respectively.
Acknowledgements
This work was funded by the Scientific and Technological Research Council of Turkey (TUBITAK grant no.111T027 and CMST COST Action CM1005), and the Research Foundation of Selcuk University (BAP).
Notes and references
- M. T. Reetz, P. Tielmann, W. Wisenhofer, W. Konen and A. Zonta, Adv. Synth. Catal., 2003, 345, 717–728 CrossRef CAS.
- M. Chen and W. R. Roush, J. Am. Chem. Soc., 2012, 134, 12320–12320 CrossRef CAS.
- A. V. Yakovenko, V. I. Boyko, V. I. Kalchenko, L. Baldini, A. Casnati, F. Sansone and R. Ungaro, J. Org. Chem., 2007, 72, 3223–3231 CrossRef CAS PubMed.
- M. Arslan, S. Sayin and M. Yilmaz, Tetrahedron: Asymmetry, 2013, 24, 982–989 CrossRef CAS PubMed.
- T. Hartman, V. Herzig, M. Budšinsky, J. Jindřich, R. Cibulka and T. Kraus, Tetrahedron: Asymmetry, 2012, 23, 1571–1583 CrossRef CAS PubMed.
- G. Tasnádi, E. Forró and F. Fülöp, Tetrahedron: Asymmetry, 2009, 20, 1771–1777 CrossRef PubMed.
- V. Mojr, V. Herzig, M. Buděšínský, R. Cibulka and T. Kraus, Chem. Commun., 2010, 46, 7599–7601 RSC.
- E. Yilmaz, M. Sezgin and M. Yilmaz, Biocatal. Biotransform., 2009, 27, 360–366 CrossRef CAS.
- E. Yilmaz, M. Sezgin and M. Yilmaz, J. Mol. Catal. B: Enzym., 2011, 69, 35–41 CrossRef CAS PubMed.
- M. F. El-Behairy and E. Sundby, Tetrahedron: Asymmetry, 2013, 24, 285–289 CrossRef CAS PubMed.
- I. Ali, N. Lahoucine, A. Ghanem and H. Y. Aboul-Enein, Talanta, 2006, 69, 1013–1017 CrossRef CAS PubMed.
- E. Ozyilmaz and S. Sayin, Appl. Biochem. Biotechnol., 2013, 170, 1871–1884 CrossRef CAS PubMed.
- F. Kartal, M. H. A. Janssen, F. Hollmann, R. A. Sheldon and A. Kilinc, J. Mol. Catal. B: Enzym., 2011, 71, 85–89 CrossRef CAS PubMed.
- S. Sayin, E. Yilmaz and M. Yilmaz, Org. Biomol. Chem., 2011, 9, 4021–4024 CAS.
- E. B. Pereira, H. F. De Castro, F. F. De Moraes and G. M. Zanin, Appl. Biochem. Biotechnol., 2001, 93, 739–752 CrossRef.
- S. Takac and M. Bakkal, Process Biochem., 2007, 42, 1021–1027 CrossRef CAS PubMed.
- A. Uyanik, N. Sen and M. Yilmaz, Bioresour. Technol., 2011, 102, 4313–4318 CrossRef CAS PubMed.
- S. Erdemir and M. Yilmaz, J. Mol. Catal. B: Enzym., 2009, 58, 29–35 CrossRef CAS PubMed.
- P. Slavik, M. Dudic, K. Flidrova, J. Sykora, I. Cisarova, S. Böhm and P. Lhoták, Org. Lett., 2012, 14, 3628–3631 CrossRef CAS PubMed.
- S. Elcin and H. Deligöz, Tetrahedron, 2013, 69, 6832–6838 CrossRef CAS PubMed.
- A. Casnati, Chem. Commun., 2013, 49, 6827–6830 RSC.
- S. Sayin, G. U. Akkus, R. Cibulka, I. Stibor and M. Yilmaz, Helv. Chim. Acta, 2011, 94, 481–486 CrossRef CAS.
- M. A. Qazi, Ü. Ocak, M. Ocak, S. Memon and I. B. Solangi, J. Fluoresc., 2013, 23, 575–590 CrossRef CAS PubMed.
- O. O. Karakus and H. Deligöz, Anal. Lett., 2010, 43, 768–775 CrossRef CAS.
- E. Ozyilmaz and S. Sayin, Bioprocess Biosyst. Eng., 2013, 36, 1803–1806 CrossRef CAS PubMed.
- O. Sahin, S. Erdemir, A. Uyanik and M. Yilmaz, Appl. Catal., A, 2009, 369, 36–41 CrossRef CAS PubMed.
- F. Ozcan, M. Ersoz and M. Yilmaz, Mater. Sci. Eng., C, 2009, 29, 2378–2383 CrossRef CAS PubMed.
- M. M. Bradford, Anal. Biochem., 1976, 72, 248–254 CrossRef CAS.
- S. H. Chiou and W. T. Wu, Biomaterials, 2004, 25, 197–204 CrossRef CAS.
- M. Bonnet, C. Leroux, Y. Chilliard and P. Martin, Mol. Cell Probes, 2001, 15, 187–194 CrossRef CAS PubMed.
- K. Faber, Biocatalysis, 1993, 8, 91–132 CrossRef CAS.
- E. Ozyilmaz, S. Sayin, M. Arslan and M. Yilmaz, Colloids Surf., B, 2014, 113, 182–189 CrossRef CAS PubMed.
- C. D. Gutsche and L. J. Bauer, J. Am. Chem. Soc., 1985, 107, 6052–6059 CrossRef CAS.
- C. S. Chen, Y. Fujimoto, G. Girdaukas and C. J. Sih, J. Am. Chem. Soc., 1982, 104, 7294–7299 CrossRef CAS.
- E. Akoz, S. Sayin and M. Yilmaz, Appl. Biochem. Biotechnol., 2014, 172, 509–523 CrossRef CAS PubMed.
|
This journal is © The Royal Society of Chemistry 2014 |
Click here to see how this site uses Cookies. View our privacy policy here.