DOI:
10.1039/C3FO60273G
(Paper)
Food Funct., 2014,
5, 50-56
In vitro and in vivo antioxidant activities of a flavonoid isolated from celery (Apium graveolens L. var. dulce)
Received
16th July 2013
, Accepted 11th October 2013
First published on 15th November 2013
Abstract
In the present article, a flavonoid was separated and purified from celery leaf through ethanol extraction, column chromatography and crystallization. The product was identified as apiin by LC/ESI-MS, and its antioxidant activities were evaluated in vitro, including by 1,1-diphenl-2-picrylhyrazyl free radical (DPPH˙), superoxide radical (O2−˙) and hydroxyl radical (OH˙) scavenging assays. IC50 values were 68.0 μg ml−1 in the DPPH assay, 0.39 mg ml−1 in the O2−˙ assay and 48.0 μg ml−1 in the OH˙ assay. The antioxidant activities were investigated in vivo with the use of mice models. All data were measured including the contents of maleic dialdehyde (MDA) and lipofuscin (LPF), the activities of superoxide dismutase (SOD), glutathione peroxidase (GSH-Px) and catalase (CAT), and the total antioxidant capacity (TAOC), in the serum, brain, heart, liver and kidney. Results showed that apiin had a remarkable scavenging activity on MDA and LPF, promoted TAOC and significantly enhanced the activities of SOD, GSH-Px and CAT.
1. Introduction
Reactive oxygen species (ROS) generated by NADPH oxidase during oxidative phosphorylation, are normal components of healthy cells. ROS are also mediators of the first defensive actions of cells and are involved in phagocytosis, apoptosis and detoxification.1 Furthermore, ROS are toxic and can have a mutagenic effect on all types of cells via the oxidation of DNA, membrane lipids and proteins.2 Fortunately, there exist some natural scavenging systems, for instance, antioxidant enzymes and polyphenols, which can destroy ROS.3–8 Phenolic compounds promote human health through reducing oxidative phenomena and decrease the risk of several diseases.9 Flavonoids are one of the main subclasses of phenolic compounds, comprised of a wide distribution of phytochemicals consisting of two aromatic rings linked by three carbons in an oxygenated heterocycle. Studies have shown that flavonoids are associated with a low risk of these ROS related diseases and damage,10 and research has focused on the development of techniques to obtain safe and effective antioxidants from natural plants. Celery (Apium graveolens L. var. dulce) is a herbaceous biennial plant in the family Apiaceae, originating from the coasts of western and northern Europe.11 It is distinguished as a low fat food and a source of digestible carbohydrates, proven rich in bioactive compounds such as vitamins, free amino acids, minerals and a high amount of dietary fibers, in addition to non-nutrients, such as volatile oils (α-selinene, butylphalide, neocoidilde, celerin), organic acids (chlorogenic acid, caffeic acid), bergapten, niacin, inositol etc.12 Among these compounds, apigenin, one of the main bioactive components in celery, belongs to a class of flavones, existing in the form of the flavonoid, apiin. Apigenin can relax rat thoracic aorta, predominantly by means of suppressing Ca2+ influx through both voltage- and receptor-operated calcium channels, which contributes to its antihypertensive activity. Furthermore, apigenin has genotoxic and radioprotective effects on radiation-induced chromosome aberrations in human lymphocytes B. Under the protection of apigenin, the frequency of cytokinesis-block micronuclei can be decreased.13
However, in comparison to the attention on the biological activity of apigenin, there are few reports on the systematic investigation of the antioxidant activity of apiin in vitro and in vivo. In particular there are few reports on the effects of apiin on enzymatic activities in vivo, such as superoxide dismutase (SOD), catalase (CAT) and glutathione (GSH), which play an important role in the antioxidant systems of living organisms that counteract reactive species to reduce the damage they cause. Consequently, the objective of this study was to extract, separate and identify this flavonoid from celery, and then determine its antioxidant activities through various in vitro methods and in vivo models.
2. Material and methods
2.1. Materials
Leaves of Apium graveolens L. var. dulce were purchased from a local supermarket. 1,1-Diphenyl-2-picrylhydrazyl was purchased from Sigma-Aldrich, China (Shanghai, China). Rutin, sodium carboxymethyl cellulose (Na-CMC), and pyrogallol acid were purchased from Beijing Chemical Reagents Company (Beijing, China). Other materials used were safranine T, ethylene diamine tetraacetic acid (EDTA), trishydroxymethylaminomethane (Tris), and silica gel (75–150 μm), obtained from Shanghai Chemical Reagent Co., Ltd. (Shanghai, China). Assay kits for MDA, LPF, SOD, GSH-Px, CAT and TAOC were obtained from Nanjing Jiancheng Institute of Bioengineering (Nanjing, China). All solutions were freshly prepared with distilled water. Stock solutions of test extracts and compounds (0.5 mg ml−1) were prepared in anhydrous ethanol. Appropriate blanks were used for the individual assays.
2.2. Preparation and separation of the plant extracts
The preparation of plant extracts was performed according to the method of Kähkönen,14 with some modifications. The leaves of celery were dried at 60 °C, powdered and extracted twice by 70% (v/v) ethanol solution (dried powder
:
ethanol solution was 1/15 (w/w)) at 80 °C for 4 h. After filtration, the filtrate was concentrated under vacuum by rotary evaporation to give the sample S1, ready for further extraction. 5 ml S1 was subjected to RP-HPLC on an Ultimate 3000 C18 semi-prep column (10 mm × 150 mm; DIONEX, USA), and eluted with a gradient mobile phase (ethyl acetate
:
methanol (90
:
10) → ethyl acetate
:
methanol (50
:
50) → methanol) within 50 minutes at a flow rate of 36 ml h−1, while being monitored at 215 nm. Fractions were collected automatically before being checked by silica gel thin-layer chromatography (TLC). The TLCs were developed in ethyl acetate
:
methanol (90
:
10) and the thin-layer was visualised under UV 365 nm after spraying with a solution of AlCl3 in ethanol. The fractions with Rf = 0.4 were combined, dried and recrystallized from ethanol at 4 °C.
2.3. Identification of flavonoid by LC/ESI-MS
LC/ESI-MS was used for flavonoid detection and identification15,16 with a reversed-phase BDS Hypersil C18 (3 μm particle size; 150 × 2.1 mm, i.d.) column. The solvent system was an acetonitrile–water mixture (A) and formic acid (B), in the following gradient: 0 min, 20% A; 10 min, 25% A; 15 min, 100% A. Elution was performed at a solvent flow rate of 0.2 ml min−1. Detection was accomplished with a Thermo Finnigan Deca XP MAX LCQ ion trap mass spectrometer equipped with an ESI interface (San Jose, CA, USA) (source voltage 3.5 kV; capillary temperature 320 °C). The mass spectrometer was operated in negative-ion mode, with a scan range from m/z 100–800 (scan rate 1.2 scans s−1).
2.4. Determination of antioxidant activity in vitro
2.4.1. DPPH scavenging assay.
The scavenging activity with respect to the free radical 1,1-diphenyl-2-picrylhydrazyl (DPPH), was determined by a modified method according to Wu17 and Luo.18 Briefly, 1 ml ethanol solution of DPPH (100 μM) was added to 1 ml extract in different concentrations. The samples were incubated at room temperature in the dark for 30 min and the optical absorbance was measured at 517 nm. The control sample was 1 ml of 100 μM DPPH mixed with 1 ml of ethanol. The percent scavenging ratio (%) was calculated by (1 − As/Ac) × 100 (where As refers to the absorbance of the extract and Ac refers to the absorbance of the control). Rutin was used as a positive control. Triplicate experiments were carried out and a dose response curve was plotted to determine the IC50, which is defined as the concentration required to obtain a scavenging capacity of 50%. A lower IC50 value corresponds to a greater antioxidant activity.
2.4.2. Superoxide radical-scavenging assay.
In this experiment, the superoxide radical was generated by the autoxidation of pyrogallol acid (PA) and the scavenging radical activity was determined by the method described by Marklund and Marklund.19 Pyrogallol acid (PA) is readily auto-oxidized at pH 8.2, generating superoxide radicals and an intermediate product which exhibits a strong absorbance at 320 nm. At the beginning of the PA auto-oxidization, the observed absorbance is linear with respect to time. However, when the antioxidant inhibits the superoxide radical, the generation velocity of the intermediate product is slowed down. The activity was calculated from the tempered slope of the resulting absorbance–time curve. The reaction was carried out in a tube with 2.8 ml Tris–HCl buffer (200 mM, pH 8.2). The mixture was incubated at 25 °C for 10 min and 0.1 ml extract solution in varying concentrations was added before preheating. The reaction was started by the addition of 0.1 ml PA solution (3 mM, preheated to 25 °C) into the tube and the reaction process was measured against distilled water at 320 nm. The absorbance was recorded every 30 s during the first 4 min. The slope (ΔA min−1) of the time response curve represented the value of the oxidation velocity (V). The control sample was made using 0.1 ml distilled water instead of extract solution. The percent inhibition activity (%) was calculated by (1 − Vs/Vc) × 100 (where Vs refers to the oxidation velocity in the sample containing extract, and Vc refers to oxidation velocity in the control sample). Rutin was used as a positive control.
2.4.3. Hydroxyl radical-scavenging assay.
The hydroxyl radical scavenging activity was determined according to the method of Zhong,20 with some modifications. Hydroxyl radicals, generated by the reaction of a Fe(II) complex with H2O2 in the presence of acid, can easily cross cell membranes, and readily react with biomolecules including carbohydrates, proteins, lipids, and DNA in cells, causing tissue damage or cell death. Hydroxyl radical scavengers have the ability to quench hydroxyl radicals that can lead to fading in the colour of safranine T. The absorbance of safranine T was detected during a fixed reaction period at 520 nm. The hydroxyl radicals were generated by the Fenton reaction model system and the scavenging activity was determined as follows. The reaction was performed in a mixture of 0.5 ml Na2HPO4–NaH2PO4 (200 mM, pH 7.4), 0.1 ml safranine T solution in ethanol (520 μg ml−1), 0.5 ml EDTANa2–Fe2+ (10 mM), 3.5 ml extract in various concentrations and 0.4 ml H2O2 (6%, V/V). The mixtures were incubated at 40 °C for 30 min and the optical absorbance was measured at 520 nm relative to a corresponding blank. The control sample was made up from 0.9 ml distilled water instead of EDTANa2–Fe2+ and H2O2, and another 3.5 ml distilled water in place of the extract solution. The percent inhibition activity (%) was calculated by (As − Ao)/(Ac − Ao) × 100 (where As refers to the absorbance of the sample containing extract, Ac refers to the absorbance of the control sample, and Ao refers to the absorbance of the original sample). Rutin was used as a positive control.
2.5. Determination of antioxidant activity in vivo
2.5.1. Experimentation on animals.
The antioxidant activity in vivo was determined according to the method of Zhang,21 with some modifications. Male ICR mice (8 weeks old, 29.9 ± 1.3 g) were purchased from Sino-British Sippr/B&K Lab Animal Ltd. (Shanghai, China). The experiment was carried out under the “Guidelines and regulations for the Care and Use of Laboratory Animal” of the Science and Technology Commission of the Shanghai Municipality, China. The mice were randomly divided into three groups of ten mice each. Group 1 (control) was only given suspending agent (0.4 ml 0.5% Na-CMC) by gavage, while groups 2 and 3 were given apiin and rutin at 50 mg kg−1 dissolved in 0.4 ml 0.5% Na-CMC by gavage, respectively. Administration was performed over 28 consecutive days. After overnight fasting, blood samples were collected by retro orbital puncture and processed to obtain serum, at which point the mice were killed by decapitation. Four organs (brain, heart, liver and kidney) were excised from the animals, and tissue homogenates were processed for biochemical analysis.
2.5.2. Biochemical analysis.
The biochemical assays were carried out according to the instructions of kits purchased from Sino-British Sippr/B&K Lab Animal Ltd. (Shanghai, China). Serum samples were obtained by centrifuging the whole blood at 2000 rpm at 4 °C for 10 min. For biochemical assays, the organ homogenates were prepared in 0.1 g ml−1 wet weight of PBS solution (50 mM, pH 7.4). Samples were centrifuged at 3000 rpm at 4 °C for 10 min, and the supernatants were then subjected to measurement of MDA, SOD, GSH-Px, CAT and TAOC levels by spectrophotometric methods, while LPF determination was performed with the use of a GENios Pro Scanning Autoreader (TECAN, Switzerland). Lipid peroxidation was estimated in terms of Thiobarbituric Acid Reactive Species (TBARS), using MDA as a standard. The MDA level was quantified with 2-thiobarbituric acid and expressed as nmol ml−1 or nmol mg−1 protein. LPF was evaluated by fluorescence at an excitation wavelength of 365 nm and an emission wavelength of 435 nm, and was expressed as kRFU ml−1 or kRFU mg−1 protein. SOD activity was analyzed by the xanthine/xanthine oxidase method; GSH-Px activity was detected with 5,5′-dithiobis-p-nitrobenzoic acid; CAT was detected with hydrogen peroxide and ammonium molybdate; TAOC was measured by a ferric reducing/antioxidant activity assay. The above four biochemical parameters were expressed as U ml−1 or U mg−1 protein. Protein content was assayed according to Bradford using bovine serum albumin as the standard.
2.6. Statistical analysis
The experimental results are expressed as the mean ± SD of parallel measurements. The significance of differences between sample means was calculated by the Independent-Samples t test using SPSS 12.0. A p value of <0.05 was regarded as a significant difference and p < 0.01 was regarded as a very significant difference.
3. Results and discussion
3.1. LC/MS analysis of flavonoid
Negative ion electrospray LC/MS analysis of the celery flavonoid is shown in Fig. 1. The Chromatogram obtained from the LC/ESI/MS/MS experiment for the ion at m/z 563.2 gave a single peak at a retention time of 4.52 min (Fig. 1A), and the corresponding MS/MS spectrum showed a fragment ion at 269.2 (Fig. 1B), which represents the fragmentation of apigenin. The mass difference between the molecular ions and the aglycone ions was 294 Da, as expected for the loss of the apiosylglucosyl derivative. The results indicated that the purified celery flavonoid was apigenin-7-apisoylglucoside, namely apiin.
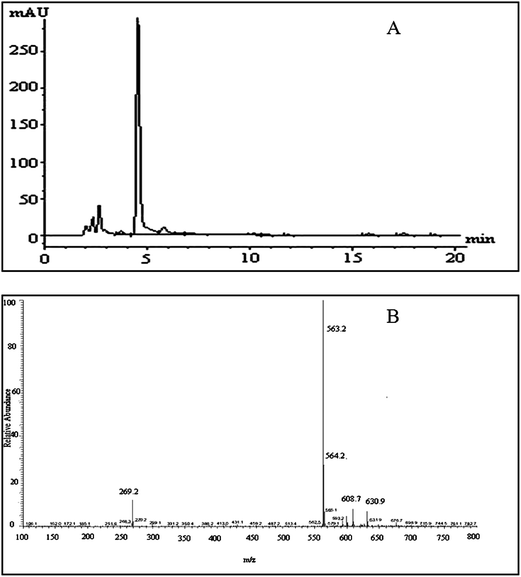 |
| Fig. 1 LC/MS analysis of the celery flavonoid, apiin. (A) HPLC profile of the purified celery flavonoid; the peak was identified as apiin. (B) ESI/MS/MS spectrum of the purified celery flavonoid. | |
3.2. Antioxidant activity in vitro
3.2.1. DPPH-scavenging activities.
DPPH is characterized as a stable free radical, owing to the delocalization of the spare electron over the whole molecule, resulting in a deep violet color at 520 nm.22 The scavenging activities of the apiin towards DPPH compared with those towards rutin are shown in Fig. 2, where it can be seen that the DPPH scavenging activity of apiin is dose dependent. Furthermore, the DPPH scavenging activity of apiin (IC50 68.0 μg ml−1) was less than that of rutin (IC50 45.6 μg ml−1), which has been proved to be a potent flavonoid exhibiting highly efficient physiological activity.23 Additionally, the extracted apiin showed a stronger scavenging activity than that of vitamin E according to Wu and Ng,24 who reported the IC50 of vitamin E to be 172.21 μg ml−1, suggesting that the extracted apiin exhibited remarkable free radical scavenging activity.
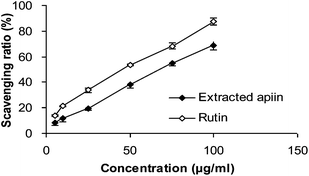 |
| Fig. 2 DPPH radical-scavenging activities of apiin, with rutin being used as a control. Data were presented as the percentage of DPPH radical scavenging, mean ± SD (n = 3). | |
3.2.2. Superoxide radical-scavenging activities.
The superoxide radical, arising either through metabolic processes or from oxygen activation by physical irradiation, is considered as the primary ROS. Superoxide radicals can further interact with other molecules to generate secondary ROS (e.g., hydroxyl radicals, hydrogen peroxide and singlet oxygen), either directly or more usually through enzyme or metal catalyzed processes.25 It can be seen from the superoxide radical-scavenging activities of apiin shown in Fig. 3 that the scavenging rate of apiin correlated well with increasing concentration. Under the same conditions, the IC50 of rutin was 0.19 mg ml−1, which indicates that the superoxide radical-scavenging activity of rutin was a little higher than that of apiin with an IC50 of 0.39 mg ml−1. The excellent superoxide radical-scavenging activity of rutin may be due to its greater amount of hydroxyl substituents,26 more specifically its 4′-OH and 7′-OH, which are not present in apiin. However, the extracted apiin demonstrated a stronger superoxide radical-scavenging activity than that of vitamin E according to Wu and Ng,24 who reported the IC50 of vitamin E to be 0.42 mg ml−1. Therefore, apiin can be considered as a good scavenger of superoxide radicals.
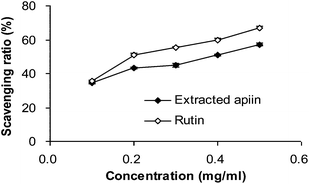 |
| Fig. 3 Superoxide radical-scavenging activities of apiin, with rutin being used as a control. Data were presented as the percentage of superoxide radical scavenging, mean ± SD (n = 3). | |
3.2.3. Hydroxyl radical-scavenging activities.
The hydroxyl radical, known as the most reactive radical, can attack and damage almost every bio-macromolecule in living cells. The best characterized biological damage caused by the hydroxyl radical is its capacity to stimulate lipid peroxidation, which occurs when hydroxyl radicals are generated close to membranes, attacking the fatty acid side chains of the membrane phospholipids.25 The results of the hydroxyl radical-scavenging activities are illustrated in Fig. 4. Apiin exhibited hydroxyl radical-scavenging activity in a concentration dependant manner, in the range of 5–100 μg ml−1. In this assay, although apiin acted as a powerful radical scavenger, the increase of scavenging activity gradually slowed down when the concentration was increased from 75 μg ml−1 to 100 μg ml−1. The same result was also found in the assay for rutin. As well as quenching the hydroxyl radical, the flavonoid can also simultaneously reduce Fe3+ into Fe2+, and Fe2+ can further react with H2O2 to form OH˙ and Fe3+, which means that the flavonoid can also promote the formation of hydroxyl radicals. Therefore, in low concentration, the flavonoid was able to quench the hydroxyl radicals, however at high concentration, the flavonoid would be promoting the formation of hydroxyl radicals, thus explaining why the plots of the lines in Fig. 4 became flat at high concentrations of extract.
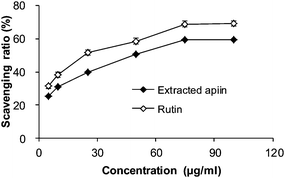 |
| Fig. 4 Hydroxyl radical-scavenging activities of apiin, and rutin was used as a control. Data were presented as the percentage of hydroxyl radical scavenging, mean ± SD (n = 3). | |
3.3. Antioxidant activity in vivo
The antioxidant properties of apiin were further confirmed with the use of in vivo assays. Brain tissue contains high concentrations of polyunsaturated fatty acids, catecholamines and oxidative metabolites, which are targets of reactive oxygen species. However, there are few antioxidant enzymes and nonenzymatic endogenous antioxidants in brain tissue, which results in the brain being under tremendous oxidative stress and aggravated neuropathic apoptosis. Meanwhile, it is known that polyunsaturated fatty acids easily form peroxides in the presence of oxygen and that this induces the production of free radicals, which attack membrane phospholipids and initiate lipid peroxidation. This peroxidative effect on membrane lipids and the low density of lipoproteins are directly related to the pathogenesis of atherosclerosis. As this condition worsens, coronary disease is induced and when ischemic reperfusion injury occurs, more radicals are produced. Hence, this can be considered as a vicious spiral which can cause significant damage to the heart.27 Furthermore, the liver is the biggest endocrine organ, involved in food digestion and detoxification. Many injurants, including medicaments and chemicals, are transformed by the liver to be non-toxic, and a great deal of radicals are produced at the same time. When the amount of free radicals exceeds the ability of endogenous antioxidants, injury will definitely occur and the function of the liver will be affected.28 The kidney is one of the primary battlefields with respect to the production and removal of free radicals. The glomerulus and its cells (epithelial cells, interstitial cells and Sertoli's cells) are capable of producing radicals. In cases of Bright's disease (acute or chronic nephritis), neutrophils, macrophages, plasma cells and eosinophils can cause enhanced oxidative stress.29 Thus, the kidney is also an organ capable of mass producing free radicals and needs more antioxidants. Therefore, the serum, brain, heart, liver and kidney of mice were employed to evaluate the antioxidant properties of the extracted apiin. Six biochemical parameters were utilized in the in vivo experiments, which were the concentrations of maleic dialdehyde (MDA) and lipofuscin (LPF), the activities of superoxide dismutase (SOD), glutathione peroxidase (GSH-Px) and catalase (CAT), and the total antioxidant capacity (TAOC).
MDA is one of the most frequently used indicators for lipid peroxidation, and it can also be an indicator of cell membrane injury, though aldehydes are a highly toxic molecule and should be considered as more than just a marker of lipid peroxidation.30Fig. 5A shows the comparison of MDA content of the serum, brain, heart, liver and kidney of the three different groups, after the administration of the extracted apiin and rutin, and it can be seen that the MDA content was generally decreased. MDA in the mice's liver was reduced significantly by over 24% compared with the control (p < 0.05). However, the decrease of MDA in the kidney was not as obvious, at less than 10% compared with the control. Furthermore, the ability of apiin to inhibit the generation of MDA was basically equal to that of rutin, which has been proven to have a strong antioxidant activity. The results of the present study have demonstrated that apiin extracted from celery can inhibit the generation of MDA in serum and brain, heart, liver and kidney tissue. LPF is the product of the peroxidation of unsaturated fatty acids, and may be symptomatic of membrane damage, in addition to damage to mitochondria and lysosomes. Pathological accumulation of LPF is associated with Alzheimer's disease, Parkinson's disease, certain lysosomal diseases, acromegaly, denervation atrophy, lipid myopathy and chronic obstructive pulmonary disease.31 As shown in Fig. 5B, the strongest inhibition of LPF generation by apiin from celery appeared in the brain (25%), followed by the serum (20%), but no significant reduction was observed in the heart, liver or kidney.
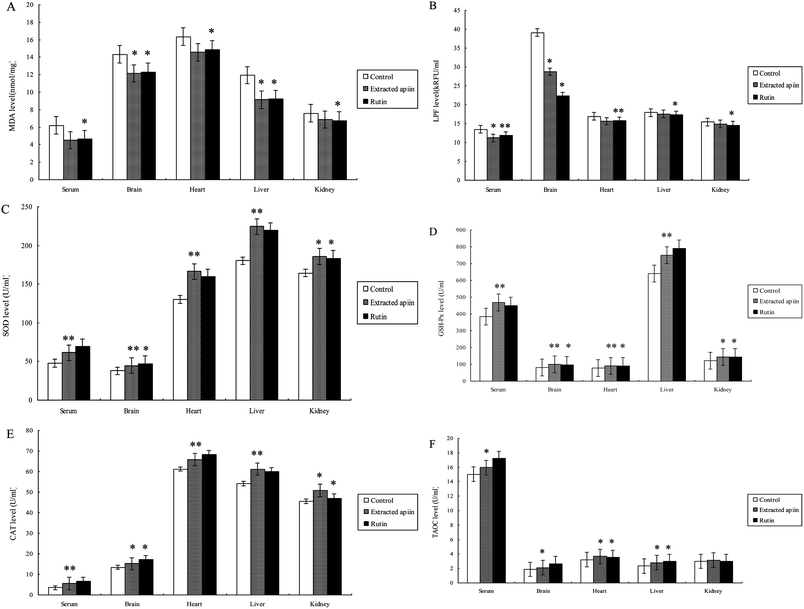 |
| Fig. 5 Comparison of (A) MDA and (B) LPF content, and the activities of (C) SOD, (D) GSH-Px, (E) CAT and (F) TAOC in the serum, brain, heart, liver and kidney of mice administered with the extracted apiin and rutin; suspending agent (0.4 ml 0.5% Na-CMC) was used as a control. Values are mean ± SD (n = 3). *p < 0.05, **p < 0.01 compared with the control. | |
Superoxide dismutase (SOD) catalyzes the dismutation of superoxide into oxygen and hydrogen peroxide. SOD decreases the generation of reactive oxygen species and oxidative stress and thus restrains endothelial activation, indicating the modulation of factors which govern adhesion molecule expression and leukocyte–endothelial interactions. As such, it is an important antioxidant defense, present on nearly all cells that are exposed to oxygen.32 Catalase (CAT) is a common enzyme found in nearly all living organisms, and it can catalyze the decomposition of hydrogen peroxide into water and oxygen. Catalase has one of the highest turnover numbers of all enzymes. One molecule of catalase can convert millions of molecules of hydrogen peroxide into water and oxygen per second. Catalase can also oxidize different toxins, such as formaldehyde, formic acid, phenols, and alcohols.33,34 GSH-Px is the general name of an enzyme family with peroxidase activity, whose main biological role is to protect the host organism from oxidative damage. The biochemical function of GSH-Px is to reduce lipid hydroperoxides to their corresponding alcohols, and to reduce free hydrogen peroxide to water.35 Thus, SOD, GSH-Px and CAT are the most important antioxidant enzymes to inhibit free radical formation, and are usually used as biomarkers to indicate ROS production.
As can be seen from the results shown in Fig. 5C–E, the activities of SOD, CAT and GSH-Px were remarkably improved in the serum, brain, heart, liver and kidney after administration of the extracted apiin. Compared with the control, the activities of SOD, GSH-Px and CAT were found to be very significantly (p < 0.01) increased in the serum (26%, 19% and 57%), brain (32%, 27% and 14%), heart (23%, 17% and 8%) liver (14%, 16% and 17%) and kidney (15%, 17% and 11%). All of the results demonstrated that the apiin extracted from celery was capable of enhancing the activities of SOD, CAT and GSH-Px, consequently representing protection against oxidative tissue damage by apiin. The enhanced activities of the antioxidant enzymes may provide an effective defense from the damaging effects of free radicals. As reported, the enhanced activities of the antioxidant enzymes were partially due to the increased mRNA expression of these enzymes.36
The total antioxidant activities (TAOC) of apiin and rutin are shown in Fig. 5F. The observed increase of TAOC promoted by apiin was 6% (p < 0.05) in serum, and about 13%, 16% and 17% in the brain, heart and liver, respectively, but no significant improvement was observed in the kidney. In addition, apiin showed a lower TAOC relative to that of rutin.
The results of the treatment of mice with apiin showed that the apiin extracted from celery can assist in the prevention of a brain oxidative state, and simultaneously promote the activity of antioxidant enzymes to provide additional protection for the heart, liver and kidney. It has clearly been demonstrated that apiin has significant antioxidant activity against various antioxidant systems in vivo.
4. Conclusions
In conclusion, the present study has demonstrated that apiin extracted from Apium graveolens L. var. dulce possesses free radical scavenging activities as well as excellent antioxidant activity in ICR mice, by protecting several important organs from oxidative stress. These antioxidant activities could have contributed, at least partially, to effects underpinning certain traditional claims of the therapeutic benefits of celery. In view of the potential use of celery in the health food industry, its therapeutic benefits and bioactive compounds behoove further investigations.
Acknowledgements
This work was supported by “the Fundamental Research Funds for the Central Universities”, and “National Major Science and Technology Projects of China (no. 2012ZX09304009)”.
References
- R. I. Salganik, J. Am. Coll. Nutr., 2001, 20, 464–472 CrossRef.
- N. L. Lerma, J. Peinado and R. A. Peinado, J. Funct. Foods, 2013, 5, 914–922 CrossRef PubMed.
- M. Y. Kim, P. Seguin, J. K. Ahn, J. J. Kim, S. C. Chun, E. H. Kim, S. H. Seo, E. Y. Kang, S. L. Kim, Y. J. Park, H. M. Ro and I. M. Chung, J. Agric. Food Chem., 2008, 56, 7265–7270 CrossRef CAS PubMed.
- Y. T. Liu, J. Sun, Z. Y. Luo, S. Q. Rao, Y. J. Su, R. R. Xu and Y. J. Yang, Food Chem. Toxicol., 2012, 50, 1238–1244 CrossRef CAS PubMed.
- I. Palacios, M. Lozano, C. Moro, M. D'Arrigo, M. A. Rostagno, J. A. Martínez, A. García-Lafuente, E. Guillamón and A. Villares, Food Chem., 2011, 128, 674–678 CrossRef CAS PubMed.
- F. S. Reis, A. Martins, L. Barros and I. C. F. R. Ferreira, Food Chem. Toxicol., 2012, 50, 1201–1207 CrossRef CAS PubMed.
- C. Sarikurkcu, B. Tepe, D. K. Semiz and M. H. Solak, Food Chem. Toxicol., 2010, 48, 1230–1233 CrossRef CAS PubMed.
- S. Y. Tsai, S. J. Huang, S. H. Lo, T. P. Wu, P. Y. Lian and J. L. Mau, Food Chem., 2009, 113, 578–584 CrossRef CAS PubMed.
- G. Mousavinejad, Z. Emam-Djomeh, K. Rezaei and M. H. H. Khodaparast, Food Chem., 2009, 115, 1274–1278 CrossRef CAS PubMed.
- L. S. Wang and G. D. Stoner, Cancer Lett., 2008, 269, 281–290 CrossRef CAS PubMed.
-
Oxford dictionary of plant sciences, ed. M. Allaby, Oxford University Press, Oxford, 1998, pp. 124–127 Search PubMed.
- J. Tang, Y. Zhang, T. G. Hartman, R. T. Rosen and C. T. Ho, J. Agric. Food Chem., 1990, 38, 1937–1940 CrossRef CAS.
- K. N. Rithidech, M. Tungjai and E. B. Whorton, Mycolres, 2005, 585, 96–104 CAS.
- M. P. Kähkönen, A. Hopia, H. J. Vuorela, J. P. Rauha, K. Pihlaja, T. S. Kujala and M. Heinonen, J. Agric. Food Chem., 1999, 47, 3954–3962 CrossRef PubMed.
- L. Lin, S. Lu and J. M. Harnly, J. Agric. Food Chem., 2007, 55, 1321–1326 CrossRef CAS PubMed.
- L. Lin, S. Mukhopadhyay, R. J. Robbins and J. M. Harnly, J. Food Compos. Anal., 2007, 20, 361–369 CrossRef CAS PubMed.
- J. H. Wu, Y. T. Tung, S. Y. Wang, L. F. Shyur, Y. H. Kuo and S. T. Chang, J. Agric. Food Chem., 2005, 53, 5917–5921 CrossRef CAS PubMed.
- X. D. Luo, M. J. Basile and E. J. Kennelly, J. Agric. Food Chem., 2002, 50, 1379–1382 CrossRef CAS PubMed.
- S. Marklund and G. Marklund, Eur. J. Biochem., 1974, 47, 469–474 CrossRef CAS.
- X. K. Zhong, X. Jin, F. Y. Lai, Q. S. Lin and J. G. Jiang, Carbohydr. Polym., 2010, 82, 722–727 CrossRef CAS PubMed.
- Q. Zhang, N. Li, G. Zhou, X. Lu, Z. Xu and Z. Li, Pharmacol Res., 2003, 48, 151–155 CrossRef CAS.
- P. Molyneux, Songklanakarin J. Sci. Technol., 2004, 26, 211–219 CAS.
- C. L. Casa, C. Lastra, V. Motilva and M. Calero, J. Ethnopharmacol., 2000, 71, 45–53 CrossRef.
- S. J. Wu and L. T. Ng, LWT–Food Sci. Technol., 2008, 41, 323–330 CrossRef CAS PubMed.
- M. Valko, D. Leibfritz, J. Moncol, M. T. D. Cronin, M. Mazur and J. Telser, Int. J. Biochem. Cell Biol., 2007, 39, 44–84 CrossRef CAS PubMed.
- J. Kitajima, T. Ishikawa and M. Satoh, Phytochemistry, 2003, 64, 1003–1011 CrossRef CAS.
- V. Calabresse, D. B. Kimball, G. Scapagnini and D. A. Butterfield, In Vivo, 2004, 18, 245–268 Search PubMed.
- N. Gharbi, M. Pressac, M. Hadchouel, H. Szwarc, S. R. Wilson and F. Moussa, Nano Lett., 2005, 5, 2578–2585 CrossRef CAS PubMed.
- E. Casalino, G. Calzaretti, C. Sblano and C. Landriscina, Toxicology, 2002, 179, 37–50 CrossRef CAS.
- H. Esterbauer, R. J. Schaur and H. Zollner, Free Radical Biol. Med., 1991, 11, 81–128 CrossRef CAS.
- J. Allaire, F. Maltais, P. LeBlanc, P. M. Simard, F. Whittom, J. F. Doyon, C. Simard and J. Jobin, MuscleNerve, 2002, 25, 383–389 CrossRef PubMed.
- J. M. McCord and I. Fridovich, Free Radical Biol. Med., 1988, 5, 363–369 CrossRef CAS.
- K. N. Rithidech, M. Tungjai and E. B. Whorton, Mutat. Res., Genet. Toxicol. Environ. Mutagen., 2005, 585, 96–104 CrossRef CAS PubMed.
- P. Chelikani, I. Fita and P. C. Loewen, Cell. Mol. Life Sci., 2004, 61, 192–208 CrossRef CAS PubMed.
- Q. Ran, H. Liang, Y. Ikeno, W. Qi, A. P. Tomas, L. J. Roberts, N. Wolf, H. VanRemmen and A. Richardson, J. Gerontol., Ser. A, 2007, 62, 932–942 CrossRef PubMed.
- W. Zhong, T. Yan, R. Lim and L. W. Oberley, Free Radical Biol. Med., 1999, 27, 1334–1345 CrossRef CAS.
|
This journal is © The Royal Society of Chemistry 2014 |