DOI:
10.1039/C4CS00164H
(Tutorial Review)
Chem. Soc. Rev., 2014,
43, 6881-6893
The physical properties of supramolecular peptide assemblies: from building block association to technological applications†
Received
11th May 2014
First published on 6th August 2014
Abstract
Bio-inspired nano-materials can be formed by the ordered assembly of elementary building blocks using recognition modules and structural elements. Among the biological sources, peptides and proteins are of special interest due to their role as major structural elements in all living systems, ranging from bacteria to humans in a continuum of magnitudes, from the nano-scale to the macro-scale. Peptides, as short as dipeptides, contain all the molecular information needed to form well-ordered structures at the nano-scale. Here, in light of the significant advancements in the field of peptide nanostructures in the last few years, we provide an updated overview of this subject. The use of these nanostructures was indeed recently demonstrated in various fields including the design of molecular motors based on nanostructure complexation with a metal–organic framework, the delivery of therapeutic agents, the development of energy storage devices and the fabrication of piezoelectric-based sensors.
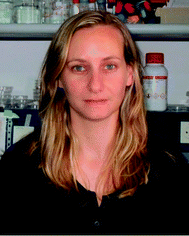
Lihi Adler-Abramovich
| Dr Lihi Adler-Abramovich studied biology at Tel Aviv University. She received both her MSc (summa cum laude) and her PhD (2010) from the Tel Aviv University at the Department of Molecular Microbiology and Biotechnology. For her PhD studies she received several prizes including the Colton Foundation Scholarship and the Marian Gertner Institute for Medical Nanosystems Scholarship. Her research interests include self-assembly of short peptides into ordered nanostructures and their utilization, as well as new paradigms for metabolite aggregation diseases. |
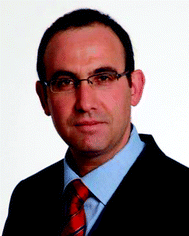
Ehud Gazit
| Prof. Ehud Gazit FRSC is the incumbent of the Chair of Biotechnology of Neurodegenerative Diseases at Tel Aviv University. He received his BSc (summa cum laude) after completing his studies at the Special Program for Outstanding Students of Tel Aviv University and his PhD (with highest distinction) from the Weizmann Institute of Science. He has been a faculty member at Tel Aviv University since 2000, after completing his postdoctoral studies at Massachusetts Institute of Technology (MIT). Gazit has received numerous awards including the Landau Research Award, the Dan David Scholarship Award, and the Herstin Award for a leading scientist under the age of 44, in 2009. |
1. Introduction
Nanostructures are ordered clusters or assemblies at the nano-scale that can be composed of inorganic or organic constituents. At those nanoscopic dimensions, materials may acquire distinctive physical, chemical, and biological properties, and may adopt functions that are remarkably different from those observed at the macro-scale. There is special interest in nanostructures formed by the process of molecular self-association, in which simple building blocks are organized by non-covalent interactions to form ordered supramolecular assemblies. A bottom-up self-assembly process is distinctly different from fabricating nanostructures that are formed as one cluster, such as metallic (e.g., gold nanoparticles) or carbon nanostructures. Self-assembly allows one to manipulate architectures as well as to control the deposition of the structures in stepwise processes. The physical properties of assemblies can be readily tuned by fine adjustment of the assembly process by physical and chemical means. Moreover, self-assembly allows the co-assembly of two or more types of building blocks, resulting in increasingly structurally complex nano-assemblies that may have physical and chemical properties that are distinct from those of the original mono-structures.1–3
Organic nanotechnology is clearly a new front in the field of molecular self-assembly of new structures and composite families at the nano-scale. The organic nanotechnology revolution has many similarities as compared to the effect of polymer chemistry development on material science in the 20th century. As with organic polymers, the organic building blocks offer diverse chemical alternatives with fine-tuning capabilities associated with the physical and chemical properties of the resulting structures. The formed nano-assemblies could be regarded as supramolecular polymers for which covalent bonds between building blocks are replaced by non-covalent interactions.4 These include hydrogen bonds, aromatic interactions, and van der Waals interactions, which provide an extensive degree of flexibility in the spatial organization of the elementary building blocks. Often, the resulting supramolecular polymers behave as typical polymers, featuring a classical phase separation phenomenon. Moreover, recently, combining more than one elementary unit was also explored in organic nanotechnology, resulting in the equivalence of supramolecular co-polymers1–3 (Fig. 1). However, self-assembled nanostructures provide a significant advantage compared with polymeric assemblies, because the deposition of structures is much simpler and the resulting physical dimensions of the assemblies can be controlled by modulating the assembly process. This was directly demonstrated by precisely controlling the peptide nanotube length using physical vapour deposition methods.5
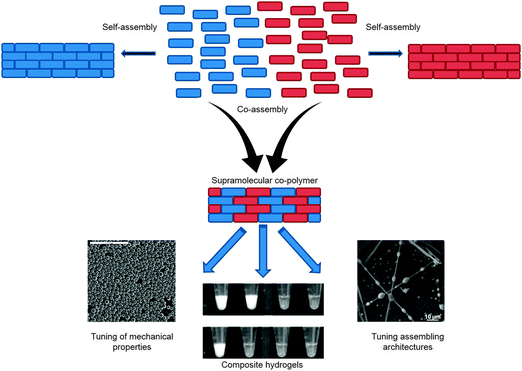 |
| Fig. 1 Supramolecular copolymers. Schematic illustration of two building blocks co-assembled into supramolecular co-polymers, for modulating the ultra-structures and its physical properties with tunable mechanical properties, composite hydrogels, and tunable assembly architectures. Reproduced with permission from ref. 14 Copyright 2010, Wiley-VCH and from ref. 3 Copyright 2012, American Chemical Society, and from ref. 2 Copyright 2012, American Chemical Society. | |
In this review, we focus on the importance of proteins in various biological systems including the amyloidal state of proteins as a basic structural motif. We provide an updated review on the self-assembly of very short peptides into ordered nanostructures, in particular, the assembly of diphenylalanine, the core recognition motif of Alzheimer's β-amyloid.6 In recent years, this building block has gained significant interest in the scientific community7,8 and the original peptide and many analogues have been synthesized, characterized and utilized in various technological applications. We will present an account of the extended family of dipeptide analogues and their self-organization properties. Moreover, we describe the utilization of the diphenylalanine peptide and its derivative-based nanostructures in various fields, including energy storage devices, displays and light-emitting devices, piezoelectric components, super-hydrophobic surfaces for self-cleaning applications, composite reinforcement, scaffolds for inorganic ultra-structures, metal–organic frameworks, ultra-sensitive sensors, 3D hydrogel scaffolds for tissue engineering, and for drug delivery agents.
2. Proteins as the dominant elements in biological systems
Among the key building blocks of organic nanotechnology, proteins and polypeptides serve as very intriguing options. Proteins function as the major building blocks of organic tissues and as key molecular scaffolds of the entire biological world. These properties are universal from the macro-scale (e.g., nail, hair, silk, and scaffolds for inorganic materials in bone and dental structures) down to the micro- and nano-scale. Examples of nano-scale protein elements include cytoskeletal filaments, microtubules, and actin. Microtubules determine the positions of membrane-enclosed organelles and direct intracellular transport, and the self-assembled actin filaments control the shape of the cell surface and are necessary for whole-cell locomotion. These cytoskeletal assemblies are found in all forms of life from the simplest bacteria to highly differentiated human cells. This is in marked contrast to nucleic acids, which were shown to possess various activities, including enzymatic ones, but do not serve as structural elements in any biological system. However, nucleic acids were extensively studied for nanotechnological applications owing to their precise and systematic recognition patterns that allow the formation of 2D and 3D objects.
Some proteins have superior mechanical properties such as extracellular spider silk fibers, which are highly adapted to meet the physiological needs of the spider. The enhanced mechanical performance of the spider web relies on the geometry of the web and on the integration of its material properties and structure. The mechanical properties are ultimately derived from the particular molecular structure of the silk, which features a composite of semi-amorphous protein and β-sheet nanocrystals. As noted above, other ubiquitous structural materials are also protein-based. This includes materials that range from the softer materials, like feathers, fur, and human hair, to more rigid materials, like beaks, claws, hooves, and horns.
3. The self-assembly of short peptide motifs
Self-assembled protein- and peptide-based nanostructures were found to be utilized in various technological applications and are envisioned to serve as key building blocks in future nanotechnological devices. Such assemblies offer the advantage of chemical diversity, since they are derived from a large structural range of building blocks, which not only include the 20 coded amino acids, but also extensive variations of non-coded and synthetic amino acids. This includes a wide-ranging repertoire of amino acid derivatives that are available as protected building blocks for chemical synthesis. Furthermore, amino acid motifs could enable the binding of metals and other inorganic entities to biological structures. Such organic–inorganic interactions include the high-affinity binding of nickel and cobalt to histidine stretches in proteins, and the affinity of gold to the thiol group of cysteine. Protein and peptide structures can also be decorated by the coupling of functional and protective groups. Moreover, proteins and peptides are biocompatible and possess the ability to be biodegradable. Another major advantage of peptide-based nanostructures is their spontaneous formation through the process of self-organization, based on efficient molecular recognition modules.
Pioneering work, some of it initiated more than 20 years ago, is related to the use of linear and cyclic peptide building blocks to form ordered nanostructures. In 1993, Ghadiri and co-workers were the first to describe hollow peptide nanotubes based on alternating D- and L-amino acids. Later on, Zhang and co-workers designed a number of linear, surfactant-like and charge-complementary peptide building blocks that were shown to efficiently self-assemble into various well-ordered nanostructures. Matsui and co-workers presented yet another group of linear peptide building blocks, the bolaamphiphile peptides that include two amide head groups connected by a hydrocarbon tail group, resulting in two hydrophilic heads that are conjugated through a hydrophobic linker. Another very intriguing amphiphilic building block family was developed by Stupp and co-workers. These units are based on the conjugation of a functional hydrophilic peptide chain head with a long hydrophobic alkyl tail. These peptide amphiphiles can present various functional biological peptide motifs including the RGD peptide and other cellular signal motifs. For an extensive review on these pioneering studies see a 2007 Tutorial Review published in this journal.8
4. Aromatic peptide nanostructures and their various structural morphologies
The smallest self-assembled peptide sequence identified is the diphenylalanine (FF). This aromatic dipeptide is derived from the core recognition motif of Alzheimer's disease β-amyloid polypeptide and is self-assembled into discrete elongated tubular assemblies (Fig. 2a).6 Subsequently, additional diphenylalanine peptide derivatives were also shown to self-assemble, forming ordered aromatic dipeptide nano-structures [ADNS], including tubes, spheres, plates, and hydrogels (Table 1).9,10 Using single-crystal X-ray diffraction studies, Görbitz found that the phenyl rings in the FF peptide generate a striking three-dimensional aromatic stacking arrangement that serves as glue between the peptide hydrogen-bonded cylinders' main chains and promotes fiber formation.11 A later study demonstrated that even a single phenylalanine amino acid can form ordered fibrillar assemblies with a distinct electron diffraction pattern. Molecular dynamics simulations suggest tight packing of the aromatic moieties, which could stabilize these supramolecular fibrils.12 These findings correlate with the importance of aromatic residues in the accelerated formation and stabilization of ordered amyloid fibrils. The phenylalanine nano-structures may play an important role in neurological damage that is observed in non-treated phenylketonuria patients.12 The ADNS system's simplicity, its facile molecular self-assembly process, as well as its inherent water solubility and dispersity have drawn significant attention in recent years, motivating several research groups to characterize and utilize the ADNS in various applications that will be discussed herein.
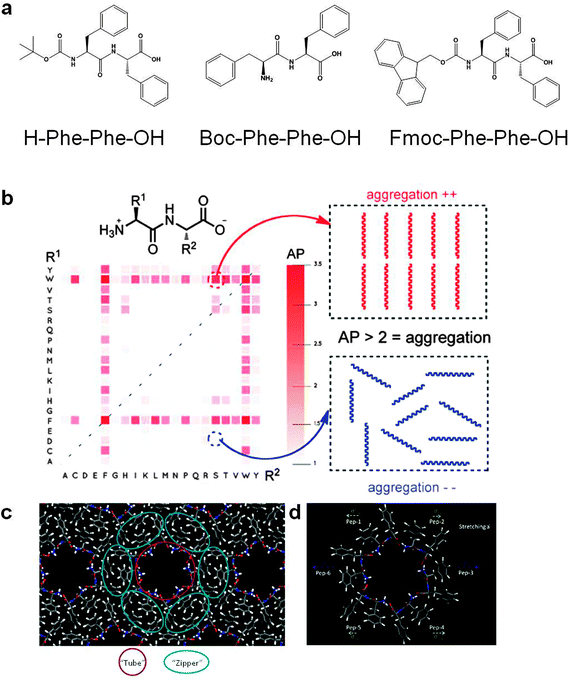 |
| Fig. 2 Aromatic dipeptide structures. (a) The molecular structure of FF, Boc-FF and Fmoc-FF peptides. (b) Virtual screening for dipeptide aggregation. Two-dimensional grids indicating the aggregation propensity score for dipeptides. Horizontal and vertical axes show the amino acid one-letter codes for the first (N-terminus) and second (C-terminus) amino acid, respectively.19 (c) Schematic partition of the diphenylalanine-based molecular solid into repeating building blocks consisting of a backbone-based “tube” surrounded by six “zipper” units consisting of two diphenylalanines each.22 (d) Schematic illustration of center-of-mass movements for each peptide unit upon stretching the lattice parameter along the x-direction.22 Reproduced with permission from ref. 19 Copyright 2011, American Chemical Society and from ref. 22 Copyright 2014, American Chemical Society. | |
Table 1 The aromatic peptide nanostructure family comprises diphenylalanine peptide and its derivatives. These peptides self-assemble into ordered structures at the nano-scale, including tubes, spheres, plates, and hydrogels
Compound name |
Assemblies structured |
Additional information |
Ref. |
H-Phe-Phe-OH (FF) |
Tubes, spheres, quantum-dots |
Young modulus = 19–30 GPa, β-sheet |
6
|
H-Phe-Phe-NH2 |
Nanotubes |
|
10, 15
|
Ac-Phe-Phe-NH2 |
Nanotubes |
|
10
|
H-Phg-Phg-OH |
Nanospheres |
|
13
|
H-Cys-Phe-Phe-OH |
Nanospheres |
|
13
|
Boc-Phe-Phe-OH (Boc-FF) |
Tubes, spheres |
Young modulus = 140–275 GPa |
10, 14, 34
|
Cbz-Phe-Phe-OH |
Amyloid-like structures |
|
10
|
Cyclo-Phe-Phe |
Tubular structures |
α-Helix or β-turn |
5, 10, 29
|
Fmoc-Phe-Phe-OH |
Fibrous-hydrogel |
G′ = 2 × 104 Pa, β-sheet |
26, 27
|
Fmoc-Phe-Gly-OH |
Fibrous-hydrogel |
|
3
|
Fmoc-2-Nal-OH |
Fibrous-hydrogel |
β-Sheet |
3
|
Fmoc-Gly-Phe-OH |
Microtubes |
|
3
|
Fmoc-Phe-Arg-Gly-Asp-OH |
Fibrous-hydrogel |
β-Sheet |
3
|
Fmoc-Arg-Gly-Asp-Phe-OH |
Fibrous-hydrogel |
β-Sheet |
3
|
Fmoc-Arg-Gly-Asp-OH |
Nanotubes |
|
3
|
Fmoc-Phe-Ser(tBu) |
Spheres |
|
3
|
Fmoc-Phe-Pro-OH |
Spheres |
|
3
|
di-D-1-napthylalanine |
Tubular structures |
|
9
|
di-D-2-napthylalanine |
Tubular structures |
Point stiffness ∼1.25 GPa |
1, 9
|
H-(p-fluoro-Phe)-(p-fluoro-Phe)-OH |
Tubular structures |
|
9
|
H-(pentafluoro-Phe)-(petafluoro-Phe)-OH |
Tubular structures |
|
9
|
H-(p-iodo-Phe)-(p-iodo-Phe)-OH |
Fibrillar structures |
|
9
|
H-(p-nitro-Phe)-(p-nitro-Phe)-OH |
Fibrillar structures and spheres |
9 |
|
H-(4-phenyl-Phe)-(4-phenyl-Phe)-OH |
Squared plates |
|
9
|
H-Phe-Phe-Phe-OH |
Nanoplates |
β-Sheet |
30
|
Nano-spherical structures can self-assemble from various members of the ADNS family, including the non-natural dipeptide, diphenylglycine, which is a simple analogue of FF, and by the Cys-Phe-Phe peptide.13 Moreover, the analogue protected by the acid-labile tert-butyl dicarbonate group, Boc-Phe-Phe-OH (Boc-FF), can alternatively assemble into either tubes or spheres. Although FF can only form mixed populations of tubes and spheres, Boc-FF can assemble into a distinctive population of tubes in aqueous solution or into a homogenous population of spheres in the presence of ethanol, displaying a notably high yield of spheres.14 Li and co-workers presented a reversible shape transition between self-assembled dipeptide nanotubes and vesicle-like structures upon a change in the cationic dipeptide (H-Phe-Phe-NH2) concentrations; the intermediate state of the transition was presented as necklace-like structures.15 The tuning of pH, ionic strength, concentration and other physical parameters was demonstrated to affect the morphology of formed FF assemblies on various surfaces emphasizing the possibility to fine tune the ultrastructural features.16 More recently, the architectural tuning was also demonstrated by co-assembly of the FF peptide, and the Boc-FF analogue resulted in the construction of beaded strings, in which spherical assemblies are connected by elongated elements.2
Tubular structures can be assembled from the non-charged Ac-Phe-Phe-NH2 aromatic dipeptide analogue, in which the N-terminal amine is acetylated and the C-terminal carboxyl is amidated, as well as by the assembly of other amine-modified analogues, thus indicating the important role of the aromatic residue and π–π interactions in the formation and stabilization of the nanostructures.10 Furthermore, the FF peptide was conjugated to several saccharides through S-linked glycosylation, including different monosaccharide, disaccharide, and sialic acid residues. These glycopeptides adopted various structures, implying that glycosylation could be a useful tool for manipulating the self-assembly process. Moreover, the solubility of these analogues was found to be much higher as compared with the original diphenylalanine building block, which could pave the way for new applications based on much higher concentrations of these nanostructures in an aqueous environment.17 Later, it was found that covalent attachment of a single mannose residue to FF leads to the retention of tubular structures, whereas the conjugation of two mannose units linked through a lysine residue results in a dramatic morphological change from tubular to spherical structures. However, a similar switch to spherical structures could be achieved by introducing a thiol residue in the mono-mannosylated FF dipeptide.18
These experimental studies of short aromatic peptides are also consistent with theoretical analysis. A comprehensive unbiased virtual screening of all 400 (20 × 20) possible natural dipeptide sequence combinations was conducted and the ability of the various dipeptides to self-assemble was determined.19 It is evident from the results (Fig. 2b) that the aromatic dipeptides have the highest propensity for the formation of supramolecular assemblies. The highest tendency for self-association was observed in phenylalanine and tryptophan containing dipeptides and, to a lower extent, with those dipeptides that included tyrosine.19
In the context of the remarkable ability of the aromatic dipeptides to self-assemble, it should be noted that while peptides in general are considered to be expensive materials, this is not the case in regard to these building blocks. The dipeptide-based materials offer a unique platform from the industrial point of view. The solution-phase synthesis of this family of aromatic dipeptides is facile and its scale-up should be straightforward. A peptide very similar to diphenylalanine is N-(L-α-aspartyl)-L-phenylalanine methyl ester (Aspartame®). This artificial sweetener is being synthesized at the multi-ton scale in edible quality and at a very low-cost (a few cents per gram). The diphenylalanine synthesis is even simpler, as no protection or tunability is needed, due to the chemically inert nature of phenylalanine side chains. Practically, the chemical complexity and synthesis efforts needed for the production of the diphenylalanine family of dipeptides are lower than that of many highly used organic chemical ingredients in various consumer products at commonplace commodities.
5. Notable physical properties of dipeptide nanostructures
5.1 Mechanical properties
Several previous studies reported the mechanical strength of the FF peptide nanotubes. Direct atomic force microscopy (AFM) measurements have indicated an average point stiffness of 160 Nm−1 and a calculated Young's modulus of about 20 GPa for nanotubular peptide assemblies.14 A similar Young's modulus value (27 ± 4 GPa) was obtained in an independent study by using a bending beam model.20 Based on their mechanical properties, FF peptide nanotubes were utilized as nanofillers for composite materials with epoxy resin as the matrix. This resulted in an increase of about 70% in shear strength and a 450% increase in peel strength as compared with unmodified epoxy, while preserving the thermal and elongation properties similar to the original resin. These effects exceed the reinforcement effect of several known inorganic nanofillers, making the peptide nanotubes [PNT] excellent nanofillers for composite materials.21 Moreover, indentation-type experiments conducted by AFM using a diamond-tip cantilever resulted in a remarkable metallic-like point stiffness of up to 885 Nm−1 and a calculated Young's modulus of up to 275 GPa for aromatic dipeptide nanospheres self-assembled by the Boc-FF peptide.14 It can be envisioned that new material reinforcement could be achieved by combining the peptide nanospheres with peptide nanotubes. Aligned peptide nanotubes could increase the bending rigidity, whereas the nanospheres could increase the pressing rigidity, similar to the role of aligned metal bars and crushed stones in reinforced concrete at the macro-scale. The mechanical properties of the nanostructure can also be tuned using two peptides of differing stereochemistry: the L-form of FF peptide and the D-form of dinapthylalanine peptide, which co-assemble to form nanotubular structures. Elevating the level of dinapthylalanine peptide in the peptide mixture decreases the nanostructure's stiffness.1 A density function theory (DFT) study was used to evaluate the basis for the mechanical rigidity of the nanotubes suggesting that despite the porous nature of the crystal lattice, there is an array of rigid nanotube backbones with interpenetrating “zipper-like” aromatic interlocks that result in stiffness and robustness (Fig. 2c and d).22
5.2 Thermal and chemical stability
As described before, self-assembled protein and peptide nanostructures are envisioned to serve as important building blocks in future nanotechnological applications. Yet the inherent thermal and chemical instability of many protein structures raises questions regarding the compatibility of incorporating peptide assemblies using common lithographic techniques, as well as the long-term durability of such devices. In contrast to this concern, FF peptide nanotubes exhibit notable thermal stability, with substantial structural stability of the tubes under wet heating conditions up to 120 °C and dry heating up to 150 °C.23 The understanding of the structural stability of the nanotubes upon dry heating was further advanced using AFM, and their degradation products were identified utilizing time-of-flight secondary ion mass spectrometry. When the nanotubes were heated to 150 °C and above, the nanotube structure degraded through the release of phenylalanine building blocks.
In addition, the stability of peptide nanospheres under extreme chemical conditions ranging from acidic (10% TFA) to alkaline (1 M NaOH) was demonstrated. The nanotubes exhibited stability and retained their ultrastructure upon exposure to organic solvents such as ethanol, methanol, isopropanol, acetone, and acetonitrile.23
5.3 Optical and electronic quantum confinement properties
It was recently shown that the FF-based nanotubes, Boc-FF-based nanospheres, and fluorenylmethoxycarbonyl (Fmoc)-FF-based hydrogel all display unique optical properties that will be discussed herein. The packing of the elementary building blocks that are assembled to form FF-nanotubes or Boc-FF-nanospheres was shown to have the optical properties of zero dimensional quantum dots (QD). However, FF-cyclization during vapour deposition at high temperatures leads to alteration of their nanoscale packing, consequently generating 2D quantum-well structures. In fact, the vertically aligned FF peptide nanotubes, deposited through physical vapour deposition, exhibited quantum confinement effects. The quantum-confined nanostructures exhibit step-like optical absorption, which is typical of quantum-wells. In addition, nanotube arrays display strong photoluminescence in the blue region along with UV absorbance of the exciton.5,24,25
As noted before, the Boc-FF peptide nanospheres exhibit optical absorbance and photoluminescence. Based on these observations, the quantum dots' radius was calculated to be ∼1 nm. The basic optical properties and the calculated dimensions of QD suggest the presence of nanometer-sized crystalline regions within the supramolecular Boc-FF spheres. The spheres themselves could range from a few tens of nm to the micron-scale. This information provides insights into the molecular packing of the spheres. Previously, the addition of the Fmoc moiety, which is widely used as a protecting group in peptide synthesis, to diphenylalanine peptide resulted in biocompatible macroscopic hydrogel formation, with nano-scale order.26,27 Furthermore, Fmoc-FF hydrogel networks exhibit gradual variation of their quantum confinement in comparison with the monomeric state. Low sub-gel concentrations show optical signatures indicating the formation of QD structures. For high monomer concentrations, which are assembled into hydrogel networks, stepwise optical absorbance with a pronounced exciton peak at the “red edge” of the spectrum was observed. Such optical properties were consistent with the formation of quantum-well structures.24,25
It was further revealed that the FF building blocks can assemble into QD-like structures at the nano-scale with pronounced exciton effects, which may enable these new forms of organic QDs to be utilized. The first stage of forming either the peptide QD or the peptide nanotubes from the FF building blocks involves dissolving the FF powder in an organic solvent, hexafluoro-2-propanol (HFIP), which allows the FF building blocks to remain in their monomeric state. For the formation of the peptide QDs or nanotubes, the HFIP solution was further diluted in methanol or H2O, respectively. The peptide QDs could also be formed by dissolving the FF peptide directly in methanol. It has been observed that the assembly process of dots to tubes and the disassembly process of tubes to dots are reversible.24,25
5.4 Piezoelectric properties of FF-peptide nanotubes
Piezoelectricity is the ability of non-centrosymmetric crystals to produce mechanical stress–strain under electric fields or charge under mechanical stress. It was recently shown that FF peptide nanotubes exhibit high polarization along the longitudinal axis, accompanied by a strong piezoelectric effect. A comparison with commercially used piezoelectric lithium niobate (LiNbO3) and lateral signal calibration yielded sufficiently high effective piezoelectric coefficient values of at least 60 pm V−1.24 Later, FF peptide tubes of different diameters and lengths were used as piezoelectrically driven micromechanical resonators. The tubes were grown from the solution and assembled on a rigid support. The conducting atomic force microscope tip was then used to both excite vibrations and to study resonance behavior.28 It was established that the self-assembled FF peptide microtubes can be used as piezoelectric resonators with the potential to be employed in sensing applications and actuating functions in micro- and nano-electromechanical systems. Sharp piezoelectric resonance in the MHz range with a quality factor of >100 was demonstrated. These parameters can be further improved by scaling down the size of the devices down to a few μm and even to sub-μm ranges.28
6. Understanding the molecular structure of the peptide nanostructures using X-ray crystallography and molecular dynamics simulations
In 2006, Görbitz set up a structural model for peptide nanotubes that is based on the single crystal structure of diphenylalanine, as obtained by fast evaporation of an aqueous solution of the peptide at 80 °C, and X-ray powder diffraction of the peptide nanotubes as obtained by a solvent-switch.11 The X-ray diffraction of the diphenylalanine nanotubes was consistent with a simulated diffractogram of the single crystal structure. The proposed model is based on arbitrarily chosen peptide nanotubes of a 110 nm outer diameter and a 50 nm inner one. The model suggests that aromatic groups generate a three dimensional aromatic stack that serves as a ‘glue’ between hydrogen-bond cylinders of the main chain of the peptides. Furthermore, the laminated construction gives exceptional strength to such a low-density porous material. The narrow peptide channels formed are hydrophilic, and may host guest molecules. Yet, the exact nature of the inner surface is not known and may be either a mixed hydrophobic/hydrophilic surface, as suggested in the model, or entirely hydrophobic.11 In recent years, the use of molecular dynamics (MD) simulations has been another important tool for studying the molecular mechanism underlying self-assembly. Aromatic peptides are excellent models for computational studies due to the their small size, which requires significantly less computational power, thus enabling all-atom calculations and extended dynamics. Indeed, further molecular insights were gained regarding the FF peptide, its cyclic form and triphenylalanine peptide (FFF) assembly into an ordered structure using implicit-solvent and replica-exchange molecular dynamics simulations.29,30 In the simulations, the peptides form aggregates, which often contain open or ring-like peptide networks, as well as elementary and network-containing structures with β-sheet characteristics. The networks are stabilized by polar and non-polar interactions, and by the surrounding aggregates. In particular, the charged termini of adjacent peptides are involved in hydrogen-bonding interactions and their aromatic side chains form “T-shaped” contacts, similar to the three-dimensional FF crystal structure.11 These interactions may assist in the FF and FFF self-assembly at the early stages, and may also stabilize the mature nanostructures. The FFF peptide has higher network propensities and increased aggregation stability with respect to the FF peptide, which can be interpreted energetically.30
Molecular dynamics simulations using an explicit solvent model were combined with experiments that study the FF peptide self-assembly process in aqueous and organic (methanol) environments. The self-assembly propensity of FF in water is evident, whereas in methanol a very weak self-assembly tendency is observed. The structural properties of FF assemblies in the two different solvents were compared to the dialanine peptide (AA) system.31 The simulation predictions showed very good qualitative agreement with the experimental data and indicated that phenyl rings are the sources for the stronger attraction, which is observed in FF aqueous solutions. This confirms the critical role postulated and experimentally observed for the phenylalanine residues in amyloid self-assembly. On the other hand, phenyl rings do not seem to affect the structure in methanol solutions.31 Further insights into the structural properties of the peptide nanotubes, beyond the crystallography information, came from dispersion-corrected DFT which uses the Tkatchenko–Scheffler scheme with and without dispersive corrections to expose the importance of van der Waals interactions in the stabilization of the assemblies. The DFT results clearly indicated that dispersion interactions are essential to the stiffness of the peptide nanotubes. It was found that despite the porous nature of the nanotubes and crystals, there is ordering into arrays of rigid backbones with interpenetrating “zipper-like” aromatic interlocks, each consisting of two diphenylalanines. Each tubular cavity is surrounded by six such “zipper” units (Fig. 2c).22
7. Spatial and temporal organization of the aromatic dipeptide nanotubes
Controlling the spatial organization of objects at the nano-scale is a key challenge in enabling their technological utilization. Therefore, much effort was dedicated to develop methodologies for the vertical and horizontal alignment of the aromatic dipeptide nanotubes (Fig. 3). One of these approaches was the fabrication of a vertically aligned nanoforest formation by evaporation-initiated unidirectional growth of a dense array of peptide tubes from a monomeric HFIP solution (Fig. 3a).32 In addition, horizontal alignment of the tubes was achieved through non-covalent coating of the tubes with a ferrofluid and the application of an external magnetic field.32 Applying a very strong, 12 Tesla, magnetic field on the peptide nanotubes also resulted in an ordered alignment without the need for additional magnetic particles.33 Inkjet technology was also applied for the patterning of peptide nanostructures on non-biological surfaces. The tubes were used as “ink” and were patterned on transparent foil and indium-tin-oxide (ITO)-coated plastic surfaces by a modified commercial inkjet printer.34 Moreover, a stable 2D-ordered film was shown to be formed by dissociation of the peptide nanotubes in N-methyl-2-pyrrolidone (NMP) solution and recrystallization of the tubes under strong non-equilibrium conditions. These centimeter-sized peptide nanotube-based films are composed of densely arranged flat spherulite films that were further used in the embedding of silver wires.35 It was demonstrated that the FF peptide can form individually dispersed rigid nanowires in the carbon disulfide (CS2) organic solvent. Owing to the rigidity and high aspect ratio of the nanowires, they can be organized into a colloidal liquid crystalline phase. The dispersion of the liquid crystalline peptide nanowires allowed one to control the macroscopic alignment of the nanowires by applying an electric field. The deposition of a drop of liquid crystalline nanowires followed by drying under an electric field yielded a well-aligned morphology of nanowires over a broad area (Fig. 3b).36 Later, dielectrophoresis was used to integrate the FF peptide nanotubes into a microchip and to manipulate the location of the peptide nanotubes in a controlled manner. The conductivity of the immobilized peptide nanotubes was studied by I–V characterization, for both single nanotube and bundles.37
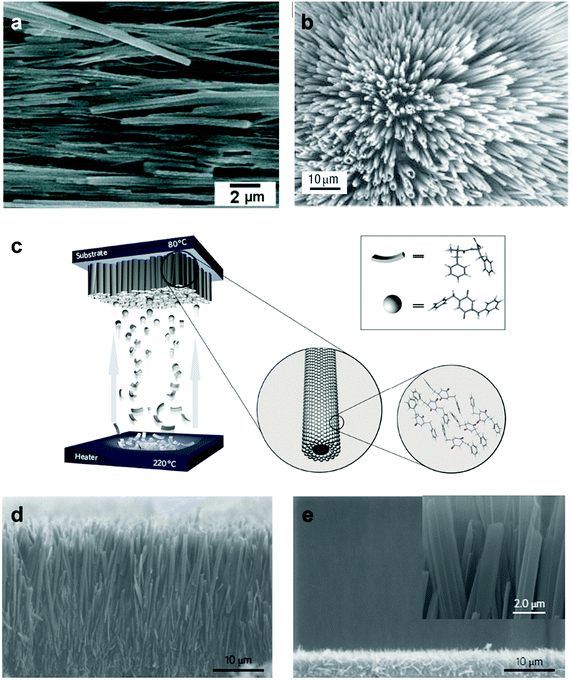 |
| Fig. 3 Alignment of the aromatic dipeptide nanotubes. (a) SEM image of vertically aligned diphenylalanine-based nanotubes self-assembled into a peptide nanoforest from HFIP solution.32 (b) Electric field induced alignment of diphenylalanine peptide nanowires.36 (c) Scheme of the physical vapour deposition [PVD] technique. During evaporation, the diphenylalanine peptide, which is heated to 220 °C, attained a cyclic structure (Cyclo–Phe–Phe peptide) and then assembled on a substrate to form ordered, vertically aligned nanotubes. (d, e) SEM image of the array side-view of vertically aligned PVD nanotubes, demonstrating the elongated micrometer tubes. The length of the tubes could be tuned with a thickness of 40 μm or 5 μm, respectively d, e. The inset shows a high-resolution SEM image of the nanotubes.5 Reproduced with permission from ref. 32 Copyright 2006, Nature Publishing Group and from ref. 36 Copyright 2007, John Wiley and Sons, and from ref. 5 Copyright 2009, Nature Publishing Group. | |
A significant limitation in the processes of peptide nano-assemblies growth and coating is the control of the physical dimensions of the formed structures. One attempt to overcome this challenge is controlling the process by the use of physical vapour deposition (PVD). This method is based on the volatility of such small aromatic building blocks upon heating and the application of vacuum.5 The ability to apply the PVD method for controlling the growth of peptide assemblies is a demonstration of the inherent advantages of small aromatic building blocks that, unlike other building blocks, can be readily evaporated under vacuum at temperatures lower than 300 °C. This controlled assembly process, in which the physical parameters could be easily tuned, allows a very precise control over the length of the formed assemblies. It was demonstrated that a uniform nanotube layer of either 4 μm or 40 μm “forest” could be achieved just by modulating the vapour deposition procedures (Fig. 3c–e).5 These vertically aligned arrays of peptide nanotubes were further used to develop high-surface-area electrodes for energy storage applications, highly hydrophobic self-cleaning surfaces, and microfluidic chips,5 and they exhibited blue luminescence based on quantum confinement.24
Ryi and Park reported the solid-phase growth of nanostructures from amorphous thin film of FF peptide. The amorphous thin peptide film was prepared by placing a drop of FF peptide dissolved in HFIP onto a solid surface in the complete absence of water vapour, followed by drying. From this amorphous thin film, they could grow aligned peptide nano-rods by applying high humidity or high thermal energy. Later, they used aniline vapour to improve the method for preparing vertically aligned peptide nanowires from FF-amorphous film. The vertically well-aligned peptide nanowires have a high aspect ratio and a long persistence length of more than 10 mm. The peptide nanowires were thermally stable up to 200 °C and a micro-pattern of peptide nanowires was formed by combining a simple soft-lithographic technique and the high-temperature aniline vapour-aging process.7
Another very promising direction is the use of supramolecular co-polymers in which the addition of capped building blocks at various ratios and settings could be utilized to modulate the assembly process. This relates to a new and exciting phase in the development of supramolecular polymer science, in which concepts that were related to conventional covalent polymers such as alternating, statistical and block copolymers could be applied to supramolecular copolymers.
Finally, the FF peptide assembly into an ordered nanostructure is extremely rapid upon dilution in water. Therefore, there is also a need for temporal control of the assembly process. An approach that allows one to precisely control the nano-assembly formation by an external signal was presented. For that purpose, a self-immolative dendrimer system containing three diphenylalanine end-groups and a trigger that is activated by PGA was designed. Although the diphenylalanine peptide building blocks cannot form tubular nanostructures in the dendritic form, these molecules readily self-assemble upon triggered release. Enzymatic cleavage by the PGA enzyme begins a cascade that results in the formation of well-ordered nanostructures.38
8. Utilization of peptide nanotubes in various applications
The minimal size and the simple synthesis of the short peptide building blocks as well as the efficient and reproducible assembly procedures and unique physical properties had attracted many research groups to develop various applications utilizing the aromatic dipeptide system (Fig. 4).
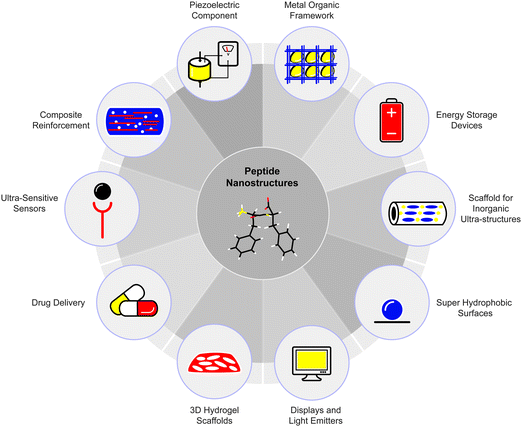 |
| Fig. 4 Various applications based on the peptide nanostructure. The aromatic dipeptide nanostructure can be utilized in many applications in various fields, including energy storage devices, displays and light-emitting devices, piezoelectric components, super hydrophobic surfaces for self-cleaning applications, composite reinforcement, scaffolds for inorganic ultra-structures, metal–organic frameworks, ultra-sensitive sensors, 3D hydrogel scaffolds for tissue engineering, and drug delivery agents. | |
8.1 Integration of peptide nanotubes into metal–organic frameworks and polymer electrolytes
The self-assembly ability of diphenylalanine peptide was utilized to develop autonomous motor systems by its integration with a metal–organic framework (MOF). MOFs are crystalline compounds consisting of metal ions and bridging organic ligands that have recently emerged as an important family of nano-scale porous materials, due to their unique structural and functional properties. The driving mechanism underlying peptide-incorporating MOF movement on the water's surface consists of two steps. The first step is the slow release of the FF peptides through the ordered pores of the MOF by the addition of EDTA, causing a controlled partial destruction of the MOF. The second step is the accumulation and self-assembly of FF peptides at the water/MOF interface, which result in a large surface tension difference around the MOF, thus providing the motion, because the released FF peptide assembly near the MOF creates a hydrophobic domain. The MOF motor moves in the direction of the higher surface tension side (Fig. 5).39
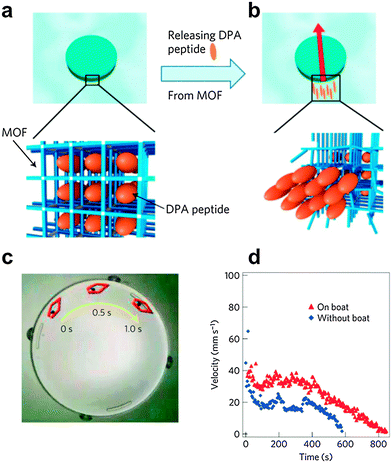 |
| Fig. 5 Diphenylalanine-metal–organic framework motor (MOF) powered by self-assembly of diphenylalanine peptides at the water interface. (a) Illustration of the mechanism of diphenylalanine-MOF motion. MOF incorporates diphenylalanine peptides in a well-ordered alignment in the nanoscale pores. (b) After releasing diphenylalanine peptides, the re-assembly of diphenylalanine peptides creates a hydrophobic domain at the end of the MOF particle. Because this domain lowers the surface tension of the MOF on the release side, the MOF particle moves in the direction of the red arrow as a result of the surface tension gradient via the Marangoni effect. (c) An overlaid light microscope image composed of three images taken every 0.5 seconds of released diphenylalanine peptides at the MOF/water interface. (d) Comparison of the velocity and lifetime of movement between the diphenylalanine-MOF particle and the boat incorporating the diphenylalanine-MOF particle.39 Reproduced with permission from ref. 39 Copyright 2012, Nature Publishing Group. | |
Furthermore, peptide nanotubes coated with magnetite nanoparticles that were added to polymer electrolyte (PE) improved its conductivity.40 The FF peptide nanotubes coated with ferrofluid, containing magnetite nanoparticles of about 5 nm, were added to LiTf:P(EO)3 polymer electrolyte. As was mentioned before, coating nanotubes with ferrofluid was found to be very useful in aligning the tubes in magnetic fields.32 Indeed, casting PE under an inhomogeneous magnetic field with the addition of only 1% FF-coated peptide nanotubes increased the total PE conductivity at room temperature by two orders of magnitude, as compared with neat PE. The magnetically oriented peptide nanotube-modified PE cast, under a magnetic field, is thermally stable, retaining its original properties even after 1 month of storage at 70 °C. As opposed to other fillers, incorporating peptide nanotubes into neat PE eliminates the formation of large spherulites. A highly homogeneous, fine-grained morphology with well-defined round-shaped crystallites develops in this composite PE under an applied magnetic field. The XRD patterns validate that the applied magnetic field influences the crystallinity of the PE-containing peptide nanotubes more strongly than it influences the neat films.40
8.2 Three-dimensional hydrogel scaffolds based on peptide nanotubes
As aforementioned, Fmoc-protected amino acids and dipeptides form hydrogels. Moreover, the end-terminal analogue of the diphenylalanine peptide, Fmoc-FF, has been shown to form hydrogels with order at the nano-scale and with notable mechanical properties.26,27 Hydrogels are of great interest as a class of materials for tissue engineering and regeneration, since they offer 3D scaffolds to support the growth of cultured cells. Fmoc-FF hydrogel can support cell growth and release of small molecules in a controllable manner, and it exhibits remarkable physical properties. The Fmoc-FF hydrogel storage modulus, G′, is higher than 2 × 104 Pa, whereas other peptide hydrogels have a G′ value of 50 Pa at a frequency of 1 Hz.26 Recently, this study was expanded by using additional members of the aromatic dipeptide family, with a new set of Fmoc-peptides, which included both natural and non-natural aromatic amino acids. One product of the peptide building blocks' assembly resulted in the formation of a hydrogel that presents a bioactive ligand, the cell-adhesive arginine-glycine-aspartate (RGD) motif at the fiber surface; therefore, it mimics certain essential features of the extracellular matrix.3,41 The nanofibrous hydrogel presented by Zhou et al. is a mixture of two aromatic short peptide derivatives: Fmoc-FF and Fmoc-RGD. The appearance of the RGD moieties on the surfaces of the fibers is tunable and was found to promote adhesion of encapsulated dermal fibroblasts through specific RGD–integrin binding, with subsequent cell spreading and proliferation.41 These scaffolds may offer an economical approach for fabricating 3D-culture scaffolds with other bioactive ligands for in vitro tissue regeneration.
Moreover, QDs were incorporated in 3D fibrous organogel, a scaffold which is based on the FF peptide. To prepare the organogels, FF dipeptide is first dissolved in HFIP and then the organic solvent is added to enable gel formation. The gelation process can be observed exclusively in chloroform or in aromatic solvents, such as toluene or xylene. The FF gelation occurred in the presence of QD solution and the gels display photoluminescence from the embedded QDs. A comparison between the emission spectra of QDs in gels and those of free QDs reveals that the emission maxima of the QDs in fibrous networks are slightly blue-shifted, indicating the attachment of the QDs on fibrils, but they maintain the original photoluminescence colors.42
8.3 Peptide nanostructure-based ultra-sensitive sensors
Another application of peptide nanostructures, which exploits their nanometric dimensions and their ability to organize peptide arrays, was the formation of sensitive electrochemical sensors based on an enzymatic reaction. The peptide nanotubes served as part of an electrochemical biosensor platform, and improved the electrochemical measurement. Moreover, a biosensor based on the covalent immobilization of modified peptide nanotubes to gold electrodes resulted in an improvement of the enzymatic molecular recognition properties and higher electrochemical sensitivity of glucose and ethanol detection.7 Later, in an extended study, a sensor based on several members of the aromatic dipeptide nanostructured family was designed and fabricated. Cyclic voltammetric and time-based amperometric techniques demonstrated that the integration of dinaphthylalanine peptide nanotubes or Boc-FF peptide nanospheres into biosensor electrodes increased the sensitivity of the electrochemical measurements.43 Notably, electrodes coated with nano-forest, a vertically aligned peptide nanotube array, showed the highest phenol detection sensitivity, 17 times that of a bare electrode. The remarkable effect of the nanostructure's deposition on the cyclic voltammetry parameters is assumed to be related to the increase in the functional electrode surface.43
In addition, utilizing both FF peptide nanotubes and carbon nanotubes (CNT) on the same amperometric enzyme-based biosensor results in highly sensitive phenol detection. The complementary properties of both PNT and CNT contributed to the synergistically elevated sensitive detection. The peptide nanotubes significantly increased the active surface area of the electrode, and together with the CNT contribution provided a faster electron transfer rate.43
Yuan et al. used cyclic voltammetry (CV) and chronoamperometry on electrodes modified with multiwalled carbon nanotubes coated with diphenylalanine peptide nanotubes (FF–MWCNT). The modified electrodes exhibited a fivefold higher, direct and unmediated response to β-reduced coenzyme nicotinamide adenine dinucleotide (NADH) than that of the bare electrode. Furthermore, an ethanol biosensor prepared by crosslinking ethanol dehydrogenase (ADH), bovine serum albumin, and FF–MWCNT deposited onto the electrode exhibited high sensitivity.44
Recently, a graphene electrode was modified with a new conjugate of peptide nanotubes and folic acid for the selective detection of human cervical cancer cells over-expressing folate receptors. Modification of the graphene electrode with peptide nanotube–folic acid led to an increase in the measured current. The human cervical cancer cells were bound to the modified electrode through the interaction of the folic acid–folate receptor. Cyclic voltammograms in the presence of [Fe(CN)6], as a redox species, demonstrated that the binding of human cervical cancer cells' folate receptor to peptide nanotube-folic acid modified electrodes lowered the electron transfer. This resulted in a decrease in the measured current upon cell binding. The peptide nanotube–folic acid electrode specifically recognized the folate receptors. This suggests future applications in early-stage diagnosis of diseases where cells over-express folate receptors, such as in cancer or leishmaniasis disease.45
Park and co-workers reported on a facile route for developing a biosensing platform based on peptide hydrogel, by co-encapsulation of enzymes as bio-receptors, and QDs as fluorescent reporters, during the self-assembly process.46 The sensor could efficiently detect the concentration of target analytes through the enzymatic conversion of the analytes to photoluminescence quenching agents that simultaneously reduced the photoluminescence intensity of QDs embedded within the hydrogel network. QDs strongly adhered along the peptide nanofibers, making the hydrogel highly photoluminescent, whereas enzyme bioreceptors were well-retained within the nanofiber network of the hydrogel formed by Fmoc-FF peptide. The photoluminescent peptide hydrogel was successfully applied for environmental monitoring, by utilizing the enzymatic detection of target analytes, such as glucose and toxic phenolic compounds.46
Later, Park and co-workers also employed photoluminescent peptide nanotubes for detecting organophosphate, which inhibits the activity of acetylcholine esterase in the brain, leading to the termination of synaptic transmission in the central nervous system.47 FF nanotube photoluminescence incorporated with lanthanide complexes was readily and selectively quenched upon exposure to organophosphate, especially paraoxon, by rapid photoluminescence quenching and nitro-group compounds (nitromethane, 4-nitrophenol, and nitrobenzene). The selective response is attributed to the role of FF nanotubes as a robust host matrix for lanthanide complexes. This sensing mechanism occurs through the inhibition of cascaded energy transfer from FF nanotubes to lanthanide ions.47
8.4 Peptide nanotubes for energy storage applications
The study of electrode modification with peptide nanotube arrays and the remarkable increase in the functional surface area, as observed by electrochemical analysis, led to studying the use of the peptide nanostructures in energy storage applications. The vertically aligned arrays of peptide nanotubes, made by the vapour deposition method, were used to develop electrostatic ultracapacitors based on peptide-nanotube-modified carbon electrodes.5 The formation of a FF peptide nanotube array strongly increases the functional surface area of the ultracapacitor electrode and significantly increases its capacitance. Peptide nanotube-modified ultracapacitor electrodes were compared to standard carbon electrodes and carbon nanotube-coated carbon electrodes. The peptide nanotube-modified electrode current response was found to be 30 times higher than the unmodified carbon electrode and 4 times higher than carbon-nanotube-modified electrodes. This estimation shows that peptide-nanotube-modification of carbon electrodes leads to significant growth in the electrical double-layer capacitance density.5
Peptide nanowires, prepared by treating amorphous diphenylalanine film with aniline vapour at an elevated temperature were readily hybridized with Co3O4 by simple reduction of Co+2 ions with NaBH4 into Co and further oxidation in an aqueous solution. The potential application of diphenylalanine–cobalt oxide hybrid as a negative electrode for Li-ion batteries was explored by constructing Swagelok-type cells with hybrid nanowires as a working electrode and examining their charge–discharge behavior.48 In comparison to pristine peptide nanowires, the peptide–Co3O4 hybrid nanowires exhibited a higher specific capacity of approximately 280 mA h g−1 at the first discharge cycle, and then it was saturated to 80 mA h g−1. This indicates that the electrochemical activity of Co3O4 was utilized in the hybrid nanowires, effectively enhancing their specific capacity as electrodes for Li-ion batteries.48
8.5 Highly hydrophobic surfaces based on peptide nanotubes
Another direction for the use of the peptide nanotube arrays was the formation of highly hydrophobic surfaces by controlled self-assembly on a glass surface.5 Preparation of highly hydrophobic surfaces is based on combining surface micro-nanotexturing, similar to a lotus-leaf type structure, and low surface energy materials.
The nanostructural coating was tailored using the vapour deposition technique; the self-organization of vertically aligned peptide nanotubes resulted in the formation of a hydrophobic surface. Fine control of the peptide-modified surface hydrophobicity can be achieved by varying the number of phenylalanine residues in the peptide building block. A glass surface was modified using vapour deposition with tri-, tetra-, and penta-phenylalanine peptides, which resulted in elevation of the water contact angle as a function of the peptide's length.5 Moreover, a vertically aligned peptide nanowire film, prepared by the self-assembly of diphenylalanine upon exposure to aniline or fluorinated aniline vapour at high temperatures, also exhibited superhydrophobic properties. The superhydrophobicity was derived from the nanoscale roughness and the decreased surface free energy by incorporating pentafluoroaniline molecules into the peptide nanowires.49 The highly hydrophobic coating may be used for self-cleaning-surfaces, smart-windows, and solar cells. These molecules also possess a key element for tailoring built-in structures for microfluidic devices.5,49
8.6 Peptide nanostructures as drug delivery agents
An indication of the potential use of the FF nanotube system in gene and drug delivery was provided by Li and co-workers. They reported that a cationic dipeptide, H-Phe-Phe-NH2, undergoes self-assembly into nanotubes at a physiological pH and that these cationic dipeptide nanotubes (CDPNTs) can also be rearranged to form vesicles upon dilution.15 This morphological switch can be reversible upon a change in the peptide concentration. Moreover, the self-assembled positively charged nanotubes were electrostatically bound to fluorescently labeled ssDNA and could enter cells readily, most likely following conversion into vesicles. The fluorescently labeled ssDNA accumulates in the cytoplasm of the cells after internalization.15 As mentioned before, Fmoc-FF peptide forms hydrogel; however, this peptide can also assemble into hydrogel nanoparticles (HNPs), which represent a promising alternative to current drug delivery carriers.50 Fmoc-FF based HNPs were formulated via a modified inverse-emulsion method using vitamin E-TPGS as an emulsion stabilizer and high-speed homogenization. The HNPs exhibited two distinct populations with an average size of 21.5 ± 1.3 and 225.9 ± 0.8 nm. HNPs served for encapsulation of doxorubicin (Dox) and 5-flourouracil (5-Fu) and exhibited release kinetics of the drugs, depending on their chemical structure, molecular weight, and hydrophobicity.50
9. Prospects for the future
The establishment of a large and diverse collection of organic bio-inspired building blocks for molecular self-assembly is one of the most important tasks for the nanotechnology field in the 21st century. The next steps involve integrating such structures into more complex assemblies as composite materials and combining more than one building block as supramolecular co-polymers. The far-reaching prospects include assemblies that not only possess passive unique chemical and physical properties, but also active and functional ones, which are self-healing, responsive, and catalytic.
Acknowledgements
We thank the support by grants from the Israeli National Nanotechnology Initiative and Helmsley Charitable Trust for a focal technology area on nanomedicines for Personalized Theranostics. We thank Gali Talor-Bilen for graphical design and thank the members of the Gazit laboratory for helpful discussion.
References
- V. L. Sedman, X. Chen, S. Allen, C. J. Roberts, V. V. Korolkov and S. J. B. Tendler, J. Microsc., 2013, 249, 165–172 CrossRef CAS PubMed
.
- S. Yuran, Y. Razvag and M. Reches, ACS Nano, 2012, 6, 9559–9566 CrossRef CAS PubMed
.
- R. Orbach, I. Mironi-Harpaz, L. Adler-Abramovich, E. Mossou, E. P. Mitchell, V. T. Forsyth, E. Gazit and D. Seliktar, Langmuir, 2012, 28, 2015–2022 CrossRef CAS PubMed
.
- T. Aida, E. W. Meijer and S. I. Stupp, Science, 2012, 335, 813–817 CrossRef CAS PubMed
.
- L. Adler-Abramovich, D. Aronov, P. Beker, M. Yevnin, S. Stempler, L. Buzhansky, G. Rosenman and E. Gazit, Nat. Nanotechnol., 2009, 4, 849–854 CrossRef CAS PubMed
.
- M. Reches and E. Gazit, Science, 2003, 300, 625–627 CrossRef CAS PubMed
.
- X. Yan, P. Zhu and J. Li, Chem. Soc. Rev., 2010, 39, 1877–1890 RSC
.
- E. Gazit, Chem. Soc. Rev., 2007, 36, 1263–1269 RSC
.
- M. Reches and E. Gazit, Phys. Biol., 2006, 3, S10–S19 CrossRef CAS PubMed
.
- M. Reches and E. Gazit, Isr. J. Chem., 2005, 45, 363–371 CrossRef CAS
.
- C. H. Görbitz, Chem. Commun., 2006, 2332–2334 RSC
.
- L. Adler-Abramovich, L. Vaks, O. Carny, D. Trudler, A. Magno, A. Caflisch, D. Frenkel and E. Gazit, Nat. Chem. Biol., 2012, 8, 701–706 CrossRef CAS PubMed
.
- M. Reches and E. Gazit, Nano Lett., 2004, 4, 581–585 CrossRef CAS
.
- L. Adler-Abramovich, N. Kol, I. Yanai, D. Barlam, R. Z. Shneck, E. Gazit and I. Rousso, Angew. Chem., Int. Ed., 2010, 49, 9939–9942 CrossRef CAS PubMed
.
- X. Yan, Q. He, K. Wang, L. Duan, Y. Cui and J. Li, Angew. Chem., Int. Ed., 2007, 46, 2431–2434 CrossRef CAS PubMed
.
- P. Kumaraswamy, R. Lakshmanan, S. Sethuraman and U. M. Krishnan, Soft Matter, 2011, 7, 2744–2754 RSC
.
- R. Roytman, L. Adler-Abramovich, K. S. A. Kumar, T.-C. Kuan, C.-C. Lin, E. Gazit and A. Brik, Org. Biomol. Chem., 2011, 9, 5755–5761 CAS
.
- N. Gour, A. K. Barman and S. Verma, J. Pept. Sci., 2012, 18, 405–412 CrossRef CAS PubMed
.
- P. W. J. M. Frederix, R. V. Ulijn, N. T. Hunt and T. Tuttle, J. Phys. Chem. Lett., 2011, 2, 2380–2384 CAS
.
- L. Niu, X. Chen, S. Allen and S. J. B. Tendler, Langmuir, 2007, 23, 7443–7446 CrossRef CAS PubMed
.
- N. Even, L. Adler-Abramovich, L. Buzhansky, H. Dodiuk and E. Gazit, Small, 2011, 7, 1007–1011 CrossRef CAS PubMed
.
- I. Azuri, L. Adler-Abramovich, E. Gazit, O. Hod and L. Kronik, J. Am. Chem. Soc., 2013, 136, 963–969 CrossRef PubMed
.
- L. Adler-Abramovich, M. Reches, V. L. Sedman, S. Allen, S. J. Tendler and E. Gazit, Langmuir, 2006, 22, 1313–1320 CrossRef CAS PubMed
.
- A. Handelman, P. Beker, N. Amdursky and G. Rosenman, Phys. Chem. Chem. Phys., 2012, 14, 6391–6408 RSC
.
- C. A. E. Hauser and S. Zhang, Nature, 2010, 468, 516–517 CrossRef CAS PubMed
.
- A. Mahler, M. Reches, M. Rechter, S. Cohen and E. Gazit, Adv. Mater., 2006, 18, 1365–1370 CrossRef CAS PubMed
.
- V. Jayawarna, M. Ali, T. A. Jowitt, A. F. Miller, A. Saiani, J. E. Gough and R. V. Ulijn, Adv. Mater., 2006, 18, 611–614 CrossRef CAS PubMed
.
- E. D. Bosne, A. Heredia, S. Kopyl, D. V. Karpinsky, A. G. Pinto and A. L. Kholkin, Appl. Phys. Lett., 2013, 102, 073504 CrossRef PubMed
.
- J. Jeon and M. S. Shell, J. Phys. Chem. B, 2014, 118, 6644–6652 CrossRef CAS PubMed
.
- P. Tamamis, L. Adler-Abramovich, M. Reches, K. Marshall, P. Sikorski, L. Serpell, E. Gazit and G. Archontis, Biophys. J., 2009, 96, 5020–5029 CrossRef CAS PubMed
.
- A. N. Rissanou, E. Georgilis, E. Kasotakis, A. Mitraki and V. Harmandaris, J. Phys. Chem. B, 2013, 117, 3962–3975 CrossRef CAS PubMed
.
- M. Reches and E. Gazit, Nat. Nanotechnol., 2006, 1, 195–200 CrossRef CAS PubMed
.
- R. J. A. Hill, V. L. Sedman, S. Allen, P. Williams, M. Paoli, L. Adler-Abramovich, E. Gazit, L. Eaves and S. J. B. Tendle, Adv. Mater., 2007, 19, 4474–4479 CrossRef CAS PubMed
.
- L. Adler-Abramovich and E. Gazit, J. Pept. Sci., 2008, 14, 217–223 CrossRef CAS PubMed
.
- N. Hendler, N. Sidelman, M. Reches, G. Gazit, Y. Rosenberg and S. Richter, Adv. Mater., 2007, 19, 1485–1488 CrossRef CAS PubMed
.
- T. H. Han, J. Kim, J. S. Park, C. B. Park, H. Ihee and S. O. Kim, Adv. Mater., 2007, 19, 3924–3927 CrossRef CAS PubMed
.
- J. Castillo, S. Tanzi, M. Dimaki and W. Svendsen, Electrophoresis, 2008, 29, 5026–5032 CrossRef CAS PubMed
.
- L. Adler-Abramovich, R. Perry, A. Sagi, E. Gazit and D. Shabat, ChemBioChem, 2007, 8, 859–862 CrossRef CAS PubMed
.
- Y. Ikezoe, G. Washino, T. Uemura, S. Kitagawa and H. Matsui, Nat. Mater., 2012, 11, 1081–1085 CAS
.
- K. Goldshtein, D. Golodnitsky, E. Peled, L. Adler-Abramovich, E. Gazit, S. Khatun, P. Stallworth and S. Greenbaum, Solid State Ionics, 2012, 220, 39–46 CrossRef CAS PubMed
.
- M. Zhou, A. M. Smith, A. K. Das, N. W. Hodson, R. F. Collins, R. V. Ulijn and J. E. Gough, Biomaterials, 2009, 30, 2523–2530 CrossRef CAS PubMed
.
- X. Yan, Y. Cui, Q. He, K. Wang and J. Li, Chem. Mater., 2008, 20, 1522–1526 CrossRef CAS
.
- L. Adler-Abramovich, M. Badihi-Mossberg, E. Gazit and J. Rishpon, Small, 2010, 6, 825–831 CrossRef CAS PubMed
.
- J. Yuan, J. Chen, X. Wu, K. Fang and L. Niu, J. Electroanal. Chem., 2011, 656, 120–124 CrossRef CAS PubMed
.
- J. J. Castillo, W. E. Svendsen, N. Rozlosnik, P. Escobar, F. Martinez and J. Castillo-Leon, Analyst, 2013, 138, 1026–1031 RSC
.
- J. H. Kim, S. Y. Lim, D. H. Nam, J. Ryu, S. H. Ku and C. B. Park, Biosens. Bioelectron., 2011, 26, 1860–1865 CrossRef CAS PubMed
.
- J. H. Kim, J. Ryu and C. B. Park, Small, 2011, 7, 718–722 CrossRef CAS PubMed
.
- J. Ryu, S.-W. Kim, K. Kang and C. B. Park, ACS Nano, 2009, 4, 159–164 CrossRef PubMed
.
- J. S. Lee, J. Ryu and C. B. Park, Soft Matter, 2009, 5, 2717–2720 RSC
.
- R. Ischakov, L. Adler-Abramovich, L. Buzhansky, T. Shekhter and E. Gazit, Bioorg. Med. Chem., 2013, 21, 3517–3522 CrossRef CAS PubMed
.
Footnote |
† This publication is dedicated to the memory of Dr Priyadarshini Kumaraswamy, a promising and talented young scientist who tragically passed away on 30.6.14 in a road accident at the age of 27. |
|
This journal is © The Royal Society of Chemistry 2014 |