Molecular self-assembly at nanometer scale modulated surfaces: trimesic acid on Ag(111), Cu(111) and Ag/Cu(111)†
Received
2nd April 2014
, Accepted 23rd April 2014
First published on 24th April 2014
Abstract
The balance between molecule–molecule and molecule–surface interactions is a determining factor in the creation of well-ordered organic networks formed by self-assembly on crystalline metal surfaces. We have used a scanning tunneling microscope under ultrahigh vacuum conditions to study the molecular self-assembly of trimesic acid on a surface that is modulated on a comparable nanometer scale as the size of the molecules. This is made of one layer of silver grown on a Cu(111) surface where it forms a periodic reconstruction. It is shown that the self-assembly of trimesic acid at room temperature, where intermolecular interactions are taking place via hydrogen bonds, is strongly disturbed due to the modulated substrate and the spatially varying potential imposed on the molecules. Annealing to 350 K partly deprotonates the molecules and changes the intermolecular interactions to stronger ionic hydrogen bonds. This reduces the influence of the modulated substrate and allows the molecules to self-assemble into long-range ordered networks on the surface. Comparisons are made to self-assembly on the flat surfaces of Ag(111) and Cu(111), where we always find well-ordered molecular networks.
Introduction
Molecular interactions at surfaces play an important role in many fields such as molecular electronics, heterogeneous catalysis, biocompatibility or sensor technology. Understanding the interaction between molecules and surfaces as well as the inter-molecular interactions on the surface is therefore crucial. For many purposes it is of advantage to assemble molecules in periodic networks covering the whole surface. This can be achieved by using organic molecules, which are allowed to self-assemble on the surface.1–5 The properties can be tailored by choosing the right functionality of the molecules. Naturally the physical shape of the molecules and the directionality of the bonds involved will influence the overall geometry of the molecular networks. A delicate balance between molecule–surface bonding, inter-molecular interactions and kinetic parameters like temperature and deposition rate will decide on the shape of the formed network. In this article we will focus on the role of the substrate6–11 by using crystalline metal surfaces that are either flat or given a nanometer scale modulation beforehand. For metal growth, it is well-known that the symmetry of the surface strongly influences the geometry of the structures grown on top.12 But organic molecules typically have a length scale (nanometer) which is much larger than the atomic periodicity of low-index metal surfaces (few Å). Therefore we have used a surface which has been given a nanoscale periodic modulation and investigate its influence on the molecular interactions and the self-assembly.
Trimesic acid (TMA) consists of a phenyl ring with three carboxylic acid groups. It has been studied on crystalline surfaces like Cu(100), Ag(111) and Au(111)13–16 under ultrahigh vacuum conditions, where it interacts via hydrogen bonding and forms well-organized structures by self-assembly.17 TMA forms an open honey-comb phase at room temperature on the noble metal surfaces Ag(111) and Au(111). A rich variety of structures are observed upon increasing coverage on Au(111)16 and on Ag(111) the TMA honey-comb phase is transformed into a close-packed phase upon annealing to 420 K.14,15 This is associated with a deprotonation of one carboxylic acid group of the TMA that allows interaction via stronger ionic hydrogen bonds between the negatively charged oxygen of the acid group and a neighbouring TMA.15,18,19 Studies on Cu(100) showed that the ordered domains are significantly smaller due to the stronger molecule–surface interaction on the more reactive Cu(100) surface.13 Also here a range of structures are observed depending on coverage and it was studied how the deprotonation depends on coverage and annealing temperature.20 Some of the TMA molecules are bonded to the surface in an upright standing configuration on Cu(100).13 These molecules appear in a round shape in the scanning tunneling microscope images while the flat lying TMA molecules are imaged in a triangular shape, as seen on Ag(111) and Au(111)13–16 and in the work presented here.
The modulated substrate we use here consists of one monolayer of Ag grown on the surface of Cu(111). Due to a lattice mismatch between Ag and Cu, this forms the well-known (9 × 9) reconstruction, with a periodicity of 2.4 nm and a corrugation on the order of 0.25 Å.21–24 These kind of weak reconstructions and Moiré patterned surface systems have been seen for many materials.25–30 We will compare the self-assembly of TMA on this modulated surface with the flat surfaces of Ag(111) and Cu(111). Changing the balance between molecule–molecule and molecule–surface interaction is shown to have a drastic effect on the self-assembly: we will demonstrate that the molecular self-assembly of TMA at room temperature is strongly disturbed due to the modulated substrate as long as TMA is interacting via fairly weak hydrogen bonding, whereas annealing allows a stronger intermolecular interaction via ionic hydrogen bonds that leads to a new well-ordered phase with shape and symmetry steered by the substrate.
Experimental
All experiments have been performed under Ultra High Vacuum (UHV) conditions (base pressure of 1 × 10−10 mbar) using a scanning tunneling microscope (STM). The metal samples have been cleaned by sputtering and subsequent annealing to 800 K. TMA molecules have been deposited by sublimation from a Knudsen cell and silver metal films have been prepared using an e-beam evaporator. All coverages in monolayers (ML) below are given in terms of how large an area fraction the molecules cover. All STM images have been taken with the sample at room temperature (RT).
Results and discussion
TMA on Ag(111) and Cu(111)
TMA on Ag(111) has previously been studied at RT and 420 K.14,15Fig. 1 shows a typical STM image of TMA on Ag(111) prepared and scanned at RT. We observe that more than 90% of the covered surface is filled with TMA in the honey-comb phase. Annealing the surface to 420 K leads to the formation of a close-packed phase. The transformation occurs through in-plane compression and deprotonation of the carboxylic acid functional groups.15 We find a density of 0.9 Molecules per nm2 (M per nm2) in the honey-comb phase and 1.44 M per nm2 in the close-packed phase.
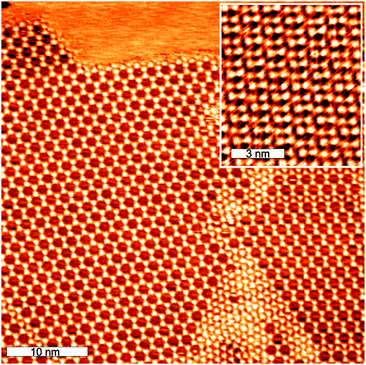 |
| Fig. 1 TMA on Ag(111) at RT. The inset shows the close-packed phase after annealing to 420 K. | |
Next we present the self-assembly of TMA on Cu(111). Fig. 2a shows the STM image of 0.7 ML TMA deposited at room temperature on Cu(111). The TMA molecules are always imaged in a triangular form and are therefore flat lying on the surface at all surface temperatures we investigated. We observe a high degree of order with mostly honey-comb structures with the same bonding distance and periodicity as on Ag(111) and a density of 0.9 M per nm2. Some parts (around 20%), of the surface are covered with closer packed structures with a density of 1.4 M per nm2 (Fig. 2b). It should be noted that this phase is different from the close-packed phase we observe after annealing as presented in the following.
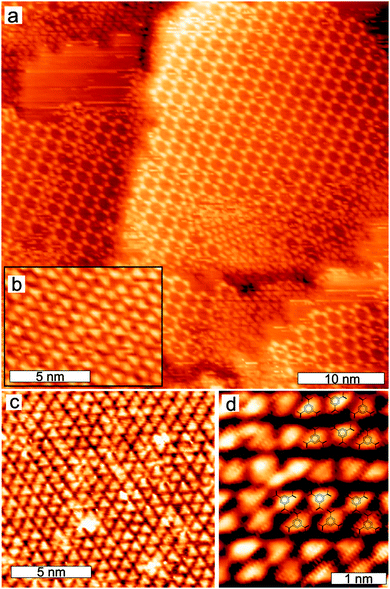 |
| Fig. 2 (a) STM image of TMA adsorbed on Cu(111) at room temperature. We observe domains with honey-comb, close-packed and empty Cu(111) areas. (b) Close-up image of the “low-density” compact structure at room temperature. (c) STM image of the “high-density” phase of TMA on Cu(111) adsorbed at room temperature followed by annealing to 325 K. All TMA molecules are imaged triangular and are therefore flat-lying. (d) High-resolution image of the TMA/Cu(111) after annealing to 325 K illustrating the two kinds of row-formations: the upper 3 rows are single-row structures and the lower 4 rows are two pairs of double-row structures. Molecular models of TMA are superimposed. | |
Upon annealing to higher temperatures the honey-comb phase disappeared and we only observed a high-density close-packed phase. This transformation happens already at 325 K. This is in contrast to Ag(111), where it is necessary to anneal to 420 K in order to transform all molecules into close-packed assemblies.15Fig. 2c and d shows two kinds of arrangements after annealing: single-row and double-row close-packed formations. We find 40% in single- and 60% in double-rows. Both the single- and double-row structures are packed with a density of 2.0 M per nm2. In the ESI† we show detailed STM images of both structures including molecular models. This is 43% higher than the close-packed phase observed after deposition (Fig. 2b) and 40% higher than the density of the close-packed phase on Ag(111). We speculate that this is due to, that the TMA molecules have lost more than one proton on the more reactive Cu surface allowing a closer interaction mediated by an increased number of ionic hydrogen bonds compared to Ag(111), where only one of the three carboxylic acid groups are deprotonated.15 We furthermore note, that the annealing to 325 K allows TMA molecules to descend–ascend steps between terraces, since we observed some terraces to be empty of molecules and neighbouring terraces to be filled with molecules. Further annealing (up to 420 K was tried) did not change the structures.
TMA on modulated Ag/Cu(111)
The modulated substrate was prepared by evaporating 1 ML of Ag on the Cu(111) surface at room temperature. The periodicity of the (9 × 9) Ag/Cu(111) layer is 2.4 nm.21–24Fig. 3a shows an STM image of sub-monolayer amounts of TMA deposited on Ag/Cu(111) at room temperature. We observe an intermixed phase consisting of regular honey-comb units, honey-comb units that are filled with a TMA molecule inside and patches with closer packed TMA molecules. The most important observation is the lack of long-range order. In the upper part of the image the modulated Ag/Cu(111) substrate is visible. Fig. 3b shows a high-resolution STM image, where it can be seen that the filled honey-comb structures have the same size as the unfilled. Furthermore, the distance between adjacent honey-comb units does not depend on the filling. Comparing sizes and inter honey-comb distances we measure the same values on Ag/Cu(111) as on Cu(111) and Ag(111). The center-filling TMA molecule is placed exactly in the middle of a honey-comb and it is interacting via hydrogen bonds between each of the 3 carboxyl groups and the 3 closest TMA's as illustrated in the model picture in Fig. 3c. These center-filled honey-comb structures are an example of host–guest architectures that play an important role in surface chemistry e.g. and has been observed previously for other systems, for example TMA on graphite.31 Due to the larger honey-comb size of TMA on graphite the center-filling TMA was believed to be placed acentric.31
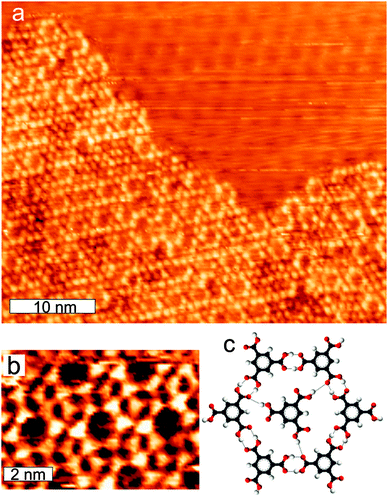 |
| Fig. 3 (a) STM image of TMA adsorbed at room temperature on 1 ML Ag/Cu(111). The modulated substrate is visible in the upper right part of the image. (b) Close-up STM image showing an area with honey-comb structures, where some of the units are center-filled with a TMA molecule. (c) An illustration of a center-filled honey-comb hexagon. The dotted lines indicate the presumed H-bonding. | |
Annealing the sample to 350 K led to a compression and formation of a well-ordered close-packed phase, where two TMA molecules pair up as it was also seen on Ag(111). This is shown in Fig 4a, where the TMA pairs are observed on the right hand side and the Ag/Cu(111) substrate on the left hand side of the image. We believe that a deprotonation has taken place, like on Ag(111).15 Interestingly our studies showed that the transformation to the close-packed phase already can happen at lower annealing temperatures compared with the Ag(111) surface, where temperatures of 420 K are required to fully convert the TMA molecules into a close-packed structure.15 We believe that Ag/Cu(111) has a higher chemical reactivity that facilitates the necessary deprotonation at a lower temperature. A small fraction, around 20%, of the TMA molecules are not paired up, as seen in the middle of Fig. 4a (the coverage ratios of the phases shown in Fig. 4a are not representative). We note that further annealing (up to 420 K was investigated) did not change the structures.
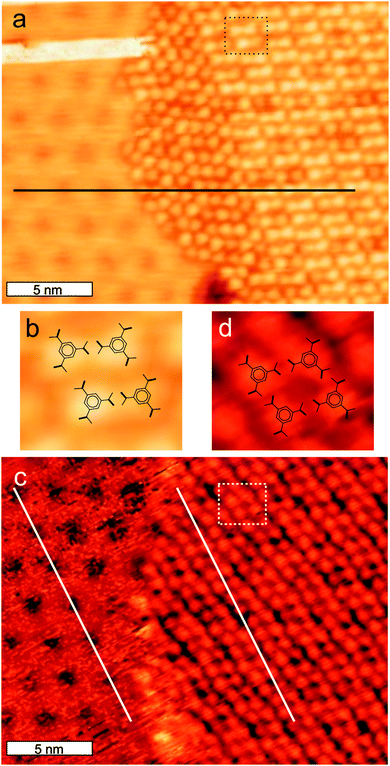 |
| Fig. 4 (a) TMA on 1 ML Ag/Cu(111) after annealing to 350 K. The TMA pairs follow the high symmetry orientation of the substrate indicated by the black line. (b) close-up of the area shown by the black dotted rectangle in a. Models of TMA molecules are superimposed. (c) TMA on 3 ML Ag/Cu(111) after annealing to 350 K. There is no correlation between the TMA network and the substrate orientation indicated by the white lines. In both images the modulated (9 × 9) Ag/Cu(111) is visible on the left side. (d) close-up of the area shown by the white dotted rectangle in c. Models of TMA molecules are superimposed. | |
Whereas deposition of TMA at room temperature led to a disordered phase we now observe perfect order. The ordered arrangement after annealing is in full agreement with the nature of the intermolecular bonding. TMA in the close-packed arrangement interact via ionic hydrogen bonds that are much stronger than the hydrogen bonds formed between intact TMA molecules upon RT deposition.15,18,19 The change in bonding mechanism and binding energy thus alters the balance between molecule–molecule and molecule–surface interaction: at RT deposition the molecule–surface interaction dominates and disturbs the ordering of the self-assembly, whereas annealing and the associated deprotonation leads to an increased intermolecular interaction that reduces the influence from the surface. The modulation the substrate imposes is apparently not strong enough to disorder the assembly of the deprotonated TMA molecules. When we look at the geometry we find that the close-packed phase on Ag/Cu(111) is to some extent similar to the one observed on Ag(111). It experiences a comparable packing density but it is important to note that the symmetry and orientation of the molecular network is now partly steered by the substrate. We find that the long axis of the TMA pairs (horizontal direction in Fig. 4a) is aligned along the Ag/Cu(111) Moiré.
We also investigated the self-assembly of TMA on multilayered Ag on Cu(111). The modulation of the substrate induced by the reconstruction is still present, but the apparent corrugation gradually decreases from 0.25 Å at 1 ML to 0.15 Å at 3 ML Ag as we add more layers of silver. Note that these numbers will depend on how sharp the STM tip is. Sub-monolayer amounts of TMA deposited on 3 ML Ag/Cu(111) at RT forms dominantly honey-comb structures like on Ag(111) and annealing to 350 K leads again to the close-packed structure consisting only of TMA pairs (Fig. 4c), but there is no correlation between the substrate and the orientation of the TMA molecules. Although the modulation is still present on 3 ML Ag/Cu(111) it appears to be too small to change the self-assembly as it was observed on 1 ML Ag, where the modulation induced disorder in the molecular structure at room temperature and forced the TMA network to partly follow the modulation upon annealing.
Conclusions
We have investigated the self-assembly of TMA on the flat surface of Cu(111) and the modulated surface of Ag/Cu(111). Comparisons to previous results on flat Ag(111) were made. TMA deposited at room temperature on Cu(111) self-assembles into the same open honey-comb networks as on Ag(111). A mild annealing to 325 K leads to a close-packed ordered phase that is distinct from Ag(111) in terms of a higher packing density and a different molecular arrangement. Room temperature adsorption of TMA on the modulated Ag/Cu(111) surface results in a molecular arrangement without long range order since the modulation of the surface disturbs the ordering of the TMA. Annealing the surface to 350 K transforms the molecular adlayer into a highly ordered close-packed phase that is partly steered by the substrate symmetry. We rationalize that TMA in the close-packed arrangement interact via ionic hydrogen bonds that are much stronger than the hydrogen bonds formed between intact TMA molecules upon RT deposition. This recovers the long range order. We have thus demonstrated how it is possible to switch the balance between molecule–molecule vs. molecule–surface interaction and the associated effects on the self-assembly.
References
- T. Yokoyama, S. Yokoyama, T. Kamikado, Y. Okuno and S. Mashiko, Nature, 2001, 413, 619 CrossRef CAS PubMed.
- J.-M. Lehn, Proc. Natl. Acad. Sci. U. S. A., 2002, 99, 4763 CrossRef CAS PubMed.
- J. V. Barth, G. Costantini and K. Kern, Nature, 2005, 437, 671 CrossRef CAS PubMed.
-
J. V. Barth, Annu. Rev. Phys. Chem., Annual Reviews, Palo Alto, 2007, vol. 58, p. 375 Search PubMed.
- E. Umbach, K. Glöckler and M. Sokolowski, Surf. Sci., 1998, 402–404, 20 CrossRef CAS.
- R. Fink, D. Gador, U. Stahl, Y. Zou and E. Umbach, Phys. Rev. B: Condens. Matter Mater. Phys., 1999, 60, 2818 CrossRef CAS.
- T. Suzuki, T. Lutz, D. Payer, N. Lin, S. L. Tait, G. Costantini and K. Kern, Phys. Chem. Chem. Phys., 2009, 11, 6498 RSC.
- S. Lukas, S. Vollmer, G. Witte and C. Wöll, J. Chem. Phys., 2001, 114, 10123 CrossRef CAS PubMed.
- N. Lin, A. Langner, S. L. Tait, C. Rajadurai, M. Ruben and K. Kern, Chem. Commun., 2007, 4860 RSC.
- J. Mao, H. Zhang, Y. Jiang, Y. Pan, M. Gao, W. Xiao and H. J. Gao, J. Am. Chem. Soc., 2009, 131, 14136 CrossRef CAS PubMed.
- H. Zhou, L. Zhang, J. Mao, G. Li, Y. Zhang, Y. Wang, S. Du, W. Hofer and H.-J. Gao, Nano Res., 2013, 6, 131 CrossRef CAS PubMed.
- H. Brune, Surf. Sci. Rep., 1998, 31, 125 CrossRef.
- A. Dmitriev, N. Lin, J. Weckesser, J. V. Barth and K. Kern, J. Phys. Chem. B, 2002, 106, 6907 CrossRef CAS.
- N. Lin, D. Payer, A. Dmitriev, T. Strunskus, C. Wöll, J. V. Barth and K. Kern, Angew. Chem., Int. Ed., 2005, 44, 1488 CrossRef CAS PubMed.
- D. Payer, A. Comisso, A. Dmitriev, T. Strunskus, N. Lin, C. Wöll, A. DeVita, J. V. Barth and K. Kern, Chem. – Eur. J., 2007, 13, 3900 CrossRef CAS PubMed.
- Y. Ye, W. Sun, Y. Wang, X. Shao, X. Xu, F. Cheng, J. Li and K. Wu, J. Phys. Chem. C, 2007, 111, 10138 CAS.
-
F. Cicoira, C. Santato and F. Rosei, in Stm and Afm Studies on (bio)molecular systems: Unravelling the nanoworld, ed. P. Samori, Springer-Verlag Berlin, Berlin, 2008, vol. 285, p. 203 Search PubMed.
- D. Braga, A. Angeloni, E. Tagliavini and F. Grepioni, J. Chem. Soc., Dalton Trans., 1998, 1961 RSC.
- M. Meot-Ner, Chem. Rev., 2005, 105, 213 CrossRef CAS.
- L. Kanninen, N. Jokinen, H. Ali-Loytty, P. Jussila, K. Lahtonen, M. Hirsimaki, M. Valden, M. Kuzmin, R. Parna and E. Nommiste, Surf. Sci., 2011, 605, 1968 CrossRef CAS PubMed.
- E. Bauer, Surf. Sci., 1967, 7, 351 CrossRef CAS.
- K. A. R. Mitchell, D. P. Woodruff and G. W. Vernon, Surf. Sci., 1974, 46, 418 CrossRef CAS.
- B. Aufray, M. Göthelid, J.-M. Gay, C. Mottet, E. Landemark, G. Falkenberg, L. Lottermoser, L. Seehofer and R. L. Johnson, Microsc., Microanal., Microstruct., 1997, 8, 167 CrossRef CAS.
- A. Bendounan, H. Cercellier, Y. Fagot-Revurat, B. Kierren, V. Y. Yurov and D. Malterre, Phys. Rev. B: Condens. Matter Mater. Phys., 2003, 67, 165412 CrossRef.
- U. Müller, D. Carnal, H. Siegenthaler, E. Schmidt, W. J. Lorenz, W. Obretenov, U. Schmidt, G. Staikov and E. Budevski, Phys. Rev. B: Condens. Matter Mater. Phys., 1992, 46, 12899 CrossRef.
- N. Nilius, E. D. L. Rienks, H.-P. Rust and H.-J. Freund, Phys. Rev. Lett., 2005, 95, 066101 CrossRef.
- M. Pivetta, F. Patthey, M. Stengel, A. Baldereschi and W.-D. Schneider, Phys. Rev. B: Condens. Matter Mater. Phys., 2005, 72, 115404 CrossRef.
- A. T. N'Diaye, S. Bleikamp, P. J. Feibelman and T. Michely, Phys. Rev. Lett., 2006, 97, 215501 CrossRef.
- J. Bork, P. Wahl, L. Diekhöner and K. Kern, New J. Phys., 2009, 11, 113051 CrossRef.
- J. Bork, J. Onsgaard and L. Diekhöner, J. Phys.: Condens. Matter, 2010, 22, 135005 CrossRef PubMed.
- S. Griessl, M. Lackinger, M. Edelwirth, M. Hietschold and W. M. Heckl, Single Mol., 2002, 3, 25 CrossRef CAS.
Footnote |
† Electronic supplementary information (ESI) available: Additional STM images and molecular models of TMA on Cu(111) after annealing. See DOI: 10.1039/c4cp01429d |
|
This journal is © the Owner Societies 2014 |