DOI:
10.1039/C3CE41942H
(Paper)
CrystEngComm, 2014,
16, 2056-2060
Characterization of tetra-n-butylphosphonium bromide semiclathrate hydrate by crystal structure analysis†
Received
25th September 2013
, Accepted 20th December 2013
First published on 24th December 2013
Abstract
We report the crystal structure analysis of the semiclathrate hydrate of tetra-n-butylphosphonium bromide (TBPB), which is a candidate material for refrigeration and gas-capture technologies. Refinement of the single crystal X-ray diffraction measurements revealed that the found structure of the TBPB hydrate has an orthorhombic structure, with the space group Pmma, and unit cell parameters a = 21.065(5), b = 12.657(3) and c = 11.992(3) Å. The chemical formula is TBPB·38H2O. The TBP ion is accommodated in a combined cage that consists of two tetrakaidecahedra and two pentakaidecahedra. The structure features three dodecahedral cages for each TBPB molecule that may accommodate small gas molecules (e.g., CH4, CO2 and N2). The structure determined in this work is compared in detail with that of a similar hydrate, tetra-n-butylammonium bromide (TBAB) hydrate. In contrast to the TBAB hydrates, the most stable structure of the TBPB hydrate is not tetragonal but orthorhombic. Since C–P has a longer bond length than C–N, the TBP ion was packed tightly by the combined cage, having complex disorder. The relative comparison of the atom positions showed that the difference in the bond lengths of the two cations is counteracted by the displacement of water molecules in the TBPB hydrate lattice.
Introduction
Semiclathrate hydrates are guest–host crystalline compounds that share many similarities with clathrate hydrates and are composed of water (host) and hydrophobic substances (guest), e.g., hydrocarbons. In clathrate hydrate structures, hydrogen-bonded water molecules form a cage-like network in which the guest molecule is accommodated. Compounds that induce semiclathrate hydrate formation also form a part of the cage-lattice that is surrounded by the water molecules.1,2 Thus, semiclathrate forming compounds can be regarded as both guest and host components. Salts containing the tetra-n-butylammonium (TBA) or phosphonium (TBP) cation have a strong tendency to form semiclathrates because the cations fit into the hydrate cage excellently.1–3
Recently, hydrates of TBP and TBA salts have been proposed as promising materials for applications in refrigeration technologies.4–9 The melting temperatures of these semiclathrate hydrates can be tuned from around 270 to 300 K by selection of an appropriate counterion. The thermophysical properties of these materials may satisfy the temperature requirements for air conditioning and offer improvements to the coefficient of performance (COP) in refrigeration applications.5 There are many candidate counter anions for TBP+ and TBA+ that may form suitable salts,10–12 however, the relationship between the counter anion and melting temperature is not well understood. It is likely related to the guest–host molecular (or ion) interactions, and crystallographic study is necessary to clarify the structural chemistry.
The TBP and TBA salt hydrates are also of interest for gas separation technologies. The semiclathrate structure contains vacant cages that may be occupied by gas molecules. The semiclathrate hydrate of tetra-n-butylammonium bromide (TBAB) has been suggested for use in the separation of CH4, N2 and CO2 gases.13–15 Tetra-n-butylphosphonium bromide (TBPB) hydrate has also been tested for hydrogen-gas storage applications.16,17 The performance of such separation and storage technologies based on semiclathrate hydrates depends on the gas-storage capacity of each molecule, i.e., fractionation between the target gases. Some phase equilibrium models have been developed for semiclathrate hydrates incorporating gaseous molecules.18,19 Many of these models are based on assumptions about the structure including the cage size because of the lack of structural data. To develop a more precise model, the analysis of the crystal structure is required. Two different stoichiometries (TBPB·38H2O and TBPB·32H2O) have been reported and their melting points are estimated to be almost equal to within ~0.1 K.3 This implies the possibility that two hydrates having congruent compositions form from an aqueous TBPB solution. There is a need to examine the most stable crystal structure through crystal structure analysis.
In this study, we report the first detailed crystal structure analysis of the TBPB semiclathrate hydrate. The TBPB·38H2O hydrate had an orthorhombic structure with a unit cell size of a = 21.065(5), b = 12.657(3) and c = 11.992(3) Å and the space group Pmma. The refined structure model is compared in detail with that of the previously reported TBAB hydrate.
Experimental
Materials
The reagents used in this study were deionized and distilled water and tetra-n-butylphosphonium bromide (TBPB) (98 mass%, Sigma-Aldrich Co. LLC.). A 30.0 mass% (2.224 mol%) of aqueous TBPB solution was gravimetrically prepared. The uncertainty for TBPB concentration in the aqueous solution was estimated to be ±2.8 mass% (±0.016 mol%) with a 95% coverage.
Single crystal X-ray diffraction measurement
The single crystal was grown in the apparatus illustrated in Fig. 1. Aqueous TBPB solution was injected into a glass vial with an outer diameter of 28 mm and a height of 95 mm. The bath temperature was maintained at 280.6 K. As shown in Fig. 2, this temperature gives ~1 K of subcooling and enables growth of mm-sized single crystals.20,21 Using this setup, the crystals with the highest melting temperature were most likely to form. The single crystals obtained in this study had a polygonal columnar shape as shown in Fig. 3. After the crystals grew to mm-size, they were removed from the solution. The sample was maintained below ~260 K for handling and transfer. The single crystal sample was cut out from a large column and mounted on an X-ray diffractometer (Bruker AXS CCD). The diffraction data were collected at 100 K. The crystal structure was solved and refined using the SHELXTL program.22 All atoms except for hydrogen were refined with anisotropic thermal parameters. Two of four butyl chains of the TBP ion were generated by symmetry operations, and site occupancies for the atoms contained in the four butyl chains were divided equally. Hydrogen atoms of the TBP ion were placed using the program and those of water were located using a difference Fourier map. The crystal-structure refinement results are summarized in Table 1. The crystal structure was visualized using VESTA.23
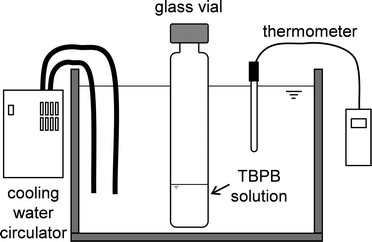 |
| Fig. 1 Apparatus for single crystal formation. | |
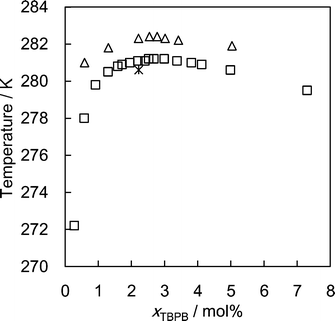 |
| Fig. 2 Formation conditions of the TBPB hydrates. △, Suginaka et al.;20 □, Mayoufi et al.;21 *, formation conditions for single crystals in this work. | |
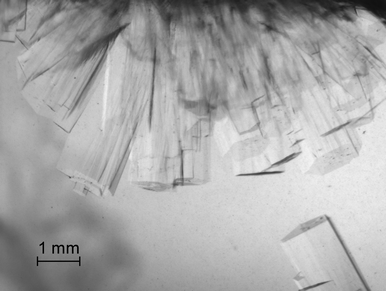 |
| Fig. 3 Single crystals of the TBPB hydrate formed in this study. | |
Table 1 Summary of crystal structure analysis for the TBPB hydrate
Empirical formula |
C32H224Br2P2O76 |
Formula weight, g mol−1 |
1023.94 |
Temperature, K |
100(3) |
Wavelength, Å |
0.71070 |
Crystal system, space group |
Orthorhombic, Pmma |
Unit cell dimensions, Å |
a = 21.065(5), b = 12.657(3), c = 11.992(3) |
Volume, Å3 |
3197.3(14) |
Z, calculated density, g cm−3 |
2, 1.054 |
Absorption coefficient, μ |
0.744 |
F(000) |
1124 |
Crystal size, mm |
0.3 × 0.2 × 0.1 |
θ range for data collection |
1.61, 27.50 |
Index ranges |
−27 < h < 27 |
|
−16 < k < 14 |
|
−15 < l < 13 |
Reflections collected/unique |
16 672/3912 |
Completeness to 2θ |
0.988 |
Refinement method |
F
2 against all reflections |
Data/restraints/parameters |
365/144/365 |
Goodness-of-fit on F2 |
1.119 |
Final R indices [I > 2Σ(I)] |
0.0646 |
R indices (all data) |
0.0715 |
Largest diff. peak and hole |
−0.80, 1.35 |
CCDC 960764 contains the supplementary CIF crystallographic data for the presently found crystal structure of the TBPB hydrate.
Results and discussion
The newly determined structure of the TBPB hydrate corresponds to Jeffrey's type-IV structure,1i.e., an orthorhombic structure with the space group Pmma, and a = 21.065(5), b = 12.657(3) and c = 11.992(3) Å. The chemical formula of the crystal was determined to be TBPB·38H2O. Fig. 4 illustrates the unit cell of the TBPB hydrate. The TBP ion is accommodated in a combined cage that consists of 2T·2P shaped voids, where T and P denote tetrakaidecahedra and pentakaidecahedra. The disorder of the TBP cation appeared through symmetry operations. The phosphonium atom and butyl chains have four possible positions. The Br− ion is located over or under the P atom in Fig. 4, replacing a water molecule in the hydrate-cage framework. There are three available D cages for each TBPB, and the structural formula can be written as TBPB·38H2O·3D. The orthorhombic lattice determined in this study is the same as that of the previously reported TBAB hydrate.10,24 In contrast to the TBAB hydrates, there is no shoulder-like shape on the phase diagram of the TBPB + water system,20,21,25 and the TBPB hydrate is considered to normally have an orthorhombic phase. The difference in polymorphism may account for the different gas capacities of the TBAB and TBPB hydrates.16,17 Based on Jeffrey's structures,1,2 the tetragonal TBAB hydrate, for which the refined structure remains unreported, is expected to have 10 D cages and 164 water molecules. The orthorhombic structure of the TBAB hydrate24 and TBPB hydrate in this study had 6 D cages and 76 water molecules. The ratio of water molecules to D cages in the unit cell is lower in the orthorhombic structure than in the tetragonal one, which provides the former structure with the superior gas capacity.
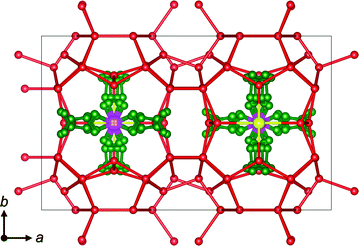 |
| Fig. 4 Unit cell of the presently found TBPB hydrate crystal. Atom types are denoted as follows: green: carbon; red: oxygen; pink: phosphorus; yellow: bromine. Hydrogen atoms are omitted for clarity. | |
The three D cages per TBPB are not all identical. One of them is highly distorted, and the other two are identical and a relatively regular dodecahedron. Such cage distortion is also observed in the previous report for the TBAB hydrate.24 It is caused by a slightly large size of the cation and a longer bond length of Br–O than that of O–O. We roughly calculated the volumes for the regular and distorted cages, and they are almost the same, i.e., 156 and 155 Å3, respectively. This fact indicates that the distorted cage is squeezed by the cation and anion, displacing the positions of water molecules but keeping its regular cage volume.
A relative comparison of the atom positions between the TBPB and the TBAB hydrates is shown in Fig. 5. In this figure, the c-axis lengths are drawn to be the same size. In comparison with the TBA ion of which butyl chains have two possible positions, the butyl chains of the TBP ion showed more complex disorder, i.e., four positions, due to the tighter packing by the combined cage. This results from the difference in the bond length between C–P and C–N which are 1.73 Å and 1.53 Å, respectively. Since this TBP cation pushes the D cage from both sides more strongly than the TBA ion, the D cage shows larger distortion in the TBPB hydrate structure. In this comparison, it is confirmed that the difference in bond length between C–P and C–N is opposed by stronger displacement of water molecules of the distorted D cage in the TBPB hydrate. It is also noteworthy that the relatively regular D cages are not affected by the cations.
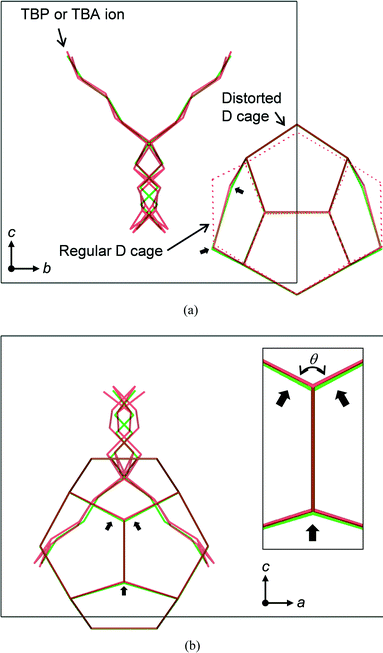 |
| Fig. 5 Superimposed pictures of the distorted D cages in the TBPB and TBAB hydrates shown with the TBP and TBA ions. The red and green lines denote the TBPB and TBAB hydrates, respectively. The thick arrows show the displacement direction of the water molecules. The c-axes are drawn to be the same size. (a) A viewpoint from the a-axis. The relatively regular D cage of the TBPB hydrate is together shown by the dotted lines for visualization of the cage distortion. (b) A viewpoint from the b-axis. An enlarged figure of the displaced water lattice is shown on the right side. The θ indicates the hydrogen-bond angles for the water molecules in the TBPB and TBAB hydrates which are 119.5° and 118.4°, respectively. | |
The roughly calculated volumes of the regular and distorted D cages for the TBAB hydrate were 161 and 153 Å3, respectively. In Fig. 5(b), the bond angles, θ, in the distorted cage lattice were slightly different. The values were 119.5° and 118.4° in the TBPB and TBAB hydrates, respectively. This fact showed that the hydrogen bonds can stretch in this small magnitude without changing the hydrate structure.
Conclusions
In this study, we report the complete crystal structure analysis of the TBPB hydrate. The hydrate corresponds to Jeffrey's type-IV structure, that is an orthorhombic structure, differing from the most stable tetragonal TBAB hydrate. This structural preference may account for the relatively high gas capacity of the TBPB hydrate due to the difference in the ratio of water molecules to D cages which can be occupied by the guest gas. The two types of D cages, i.e., relatively regular and distorted cages, are found, and they have similar volumes. This implies that the two types of cages have a different preference for small gas molecules. We compared the TBPB and TBAB hydrates based on their structures, which are the same hydrate structure but have different cations. The 0.2 Å difference in the bond length between C–P of the TBP ion and C–N of the TBA ion is compensated for by the displacement of water molecules rather than by the expansion of the unit cell volume. This may be the key factor for the stabilization capability of the TBP salts on the semiclathrate structure, the hydrates of which have slightly lower melting temperatures than the TBA salt hydrates. For the gas capacities, the two types of D cages in the TBPB and TBAB hydrate structures have almost the same volumes. However, the distorted D cage in the TBPB hydrate is squeezed more strongly than that in the TBAB hydrate, which may provide a different preference for a guest gas molecule, not in volume but in shape. This fact implies that these materials have distinct separation capabilities for specific molecules that have similar sizes.
Acknowledgements
This study was subsidized in part by the Keio University Global Center of Excellence Program “Center for Education and Research of Symbiotic, Safe and Secure System Design”. SM thanks the Japan Society for the Promotion of Science (JSPS) for support provided through the program “Grant-in-Aid for JSPS Fellows of the Ministry of Education, Culture, Sports, Science, and Technology (MEXT)” (Grant no. 23-56572). We also thank Dr. Midori Gotoh for valuable discussion about the single crystal X-ray diffraction measurements and structure refinement. SM thanks Dr. John A. Ripmeester for instruction of semiclathrate-hydrate structures.
Notes and references
-
G. A. Jeffrey, in Inclusion Compounds, ed. J. L. Atwood, J. E. D. Davies and D. D. MacNicol, Academic Press, London, 1984, vol. 1, ch. 5 Search PubMed.
-
D. W. Davidson, in Water. A Comprehensive Treatise, ed. F. Franks, Plenum Press, New York, NY, 1973 Search PubMed.
- Y. A. Dyadin and K. A. Udachin, J. Struct. Chem., 1987, 28, 75 CrossRef CAS.
- H. Ogoshi and S. Takao, JFE Technical Report, 2004, 3, 1 CAS.
- K. Sato, H. Tokutomi and R. Ohmura, Fluid Phase Equilib., 2013, 337, 115 CrossRef CAS PubMed.
- M. Darbouret, M. Cournil and J.-M. Herri, Int. J. Refrig., 2005, 28, 663 CrossRef CAS PubMed.
- P. Clain, A. Delahaye, L. Fournaison, N. Mayoufi, D. Dalmazzone and W. Fürst, Chem. Eng. J., 2012, 193–194, 112 CrossRef CAS PubMed.
- W. Lin, D. Dalmazzone, W. Fürst, A. Delahaye, L. Fournaison and P. Clain, J. Chem. Thermodyn., 2013, 61, 132 CrossRef CAS PubMed.
- P. Zhang, N. Ye, H. Zhu and X. Xiao, J. Chem. Eng. Data, 2013, 58, 1781 CrossRef CAS.
- K. A. Udachin and J. A. Ripmeester, Angew. Chem., Int. Ed., 1999, 38, 1983 CrossRef CAS.
- T. Rodionova, V. Komarov, J. Lipkowski and N. Kuratieva, New J. Chem., 2010, 34, 432 RSC.
- H. Sakamoto, K. Sato, K. Shiraiwa, S. Takeya, M. Nakajima and R. Ohmura, RSC Adv., 2011, 1, 315 RSC.
- D. Zhong and P. Englezos, Energy Fuels, 2012, 26, 2098 CrossRef CAS.
- N. H. Duc, F. Chauvy and J.-M. Herri, Energy Convers. Manage., 2007, 48, 1313 CrossRef CAS PubMed.
- L.-L. Shi, D.-Q. Liang and D.-L. Li, J. Chem. Eng. Data, 2013, 58, 2125 CrossRef CAS.
- J. Deschamps and D. Dalmazzone, J. Chem. Eng. Data, 2010, 55, 3395 CrossRef CAS.
- Y. Fujisawa, T. Tsuda, S. Hashimoto, T. Sugahara and K. Ohgaki, Chem. Eng. Sci., 2012, 68, 660 CrossRef CAS PubMed.
- P. Paricaud, J. Phys. Chem. B, 2011, 115, 288 CrossRef CAS PubMed.
-
M. Kwaterski and J.-M. Herri, in Proceedings of the 7th International Conference on Gas Hydrates (ICGH 2011), Edinburgh, Scotland, United Kingdom, July 17–21, 2011 Search PubMed.
- T. Suginaka, H. Sakamoto, K. Iino, S. Takeya, M. Nakajima and R. Ohmura, Fluid Phase Equilib., 2012, 317, 25 CrossRef CAS PubMed.
- N. Mayoufi, D. Dalmazzone, A. Delahaye, P. Clain, L. Fournaison and W. Fürst, J. Chem. Eng. Data, 2011, 56, 2987 CrossRef CAS.
- G. M. Sheldrick, Acta Crystallogr., Sect. A: Found. Crystallogr., 1990, 46, 467 CrossRef.
- K. Momma and F. Izumi, J. Appl. Crystallogr., 2011, 44, 1272 CrossRef CAS.
- W. Shimada, M. Shiro, H. Kondo, S. Takeya, H. Oyama, T. Ebinuma and H. Narita, Acta Crystallogr., Sect. C: Cryst. Struct. Commun., 2005, 61, o65 Search PubMed.
- H. Oyama, W. Shimada, T. Ebinuma, Y. Kamata, S. Takeya, T. Uchida, J. Nagao and H. Narita, Fluid Phase Equilib., 2005, 234, 131 CrossRef CAS PubMed.
|
This journal is © The Royal Society of Chemistry 2014 |