DOI:
10.1039/C3TC31075B
(Communication)
J. Mater. Chem. C, 2013,
1, 5619-5623
Micro-focused X-ray diffraction characterization of high-quality [6,6]-phenyl-C61-butyric acid methyl ester single crystals without solvent impurities†
Received 5th June 2013, Accepted 27th June 2013
First published on 3rd July 2013
Abstract
We report the preparation of high-quality, solvent-free [6,6]-phenyl-C61-butyric acid methyl ester (PCBM) large single crystals (size up to 0.5 mm) by slow drying of a chlorobenzene solution at room temperature. The monoclinic structure containing four PCBM molecules per unit cell was successfully solved (R-factor = 0.0512) via micro-focused X-ray diffraction and employed as a reliable experimental model for further molecular dynamics simulations. We find that the first peak of the simulated fullerene–fullerene radial distribution function is centred at 10.05 Å, giving a nearest neighbour coordination number of 7.0. The work reported herein provides the structural basis for a fundamental understanding of charge transport in this important functional material that is particularly relevant to organic solar cells.
1 Introduction
Achieving large, high-quality, and solvent-free crystals of application-relevant organic semiconductors1,2 is crucial to affording ever more detailed insights into the fundamental charge and exciton physics underpinning the technology of photovoltaic diodes (PVDs), field-effect transistors (FETs), and light-emitting diodes (LEDs).3–5 However, this is often far from trivial, not only for high molecular weight materials/polymers such as poly(3-hexylthiophene-2,5-diyl) (P3HT) due to chain entanglement and entropic factors, but also for smaller molecular weight materials such as [6,6]-phenyl-C61-butyric acid methyl ester (PCBM), owing to inclusion of solvent molecules and/or other impurities. PCBM is a particularly relevant example because it is one of the most popular choices as the electron acceptor in organic solar cells adopting a type-II heterojunction design. Such an architecture is necessary to favour exciton splitting against a substantial exciton binding energy (0.2−1 eV or so),6 in either bilayer7 or bulk heterojunction (BHJ) organic solar cells in which the formation of an interpenetrated electron donor–acceptor network has the potential to provide large interface areas and domains that are as close as currently possible to the exciton diffusion range, thereby optimising charge generation.8,9 Interestingly both amorphous and microcrystalline domains have also been identified in BHJs.10In general, it is thus crucial to understand the physics of charge transport in PCBM in order to optimise this type of solar cells. Despite the large number of papers investigating the morphological and electronic properties of PCBM in solid blends with electron-donor materials,11–19 a clear understanding of its aggregation behaviour in such a complex system is yet to emerge. Preparation and characterisation of solvent-free crystals have never previously been reported, with the exception of a very recent publication of which we became aware in the final stages of preparation of this manuscript.20
Previously, Rispens et al. have proved that the solvent choice plays a critical role in the PCBM crystallization behaviour.21 In particular, when drop-cast from ortho-dichlorobenzene (oDCB) PCBM was found to form red-brown platelet-shaped crystals with a monoclinic unit cell (a = 13.76, b = 16.63, c = 19.08 Å, and β = 105.3°), whereas casting from chlorobenzene (CB) leads to the formation of reddish triangle-shaped crystals with a triclinic unit cell (a = 13.83, b = 15.29, c = 19.25 Å, α = 80.3°, β = 78.6°, and γ = 80.41°). However, regardless of the solvent choice, these authors have noticed solvent inclusion inside the crystals. Furthermore, the substrate nature seems to affect the crystallization pathways undertaken by PCBM. In this context, Dabirian et al. prepared crystals via dip-coating deposition on three different substrates, observing the formation of crystals with different shapes, namely, hexagonal crystals on silanized SiOx, amorphous aggregates on graphite and snowflake-like crystals on Au.22 More recently Zheng and Han have prepared hexagonal crystals incorporating solvent molecules via liquid–liquid interfacial precipitation.23 In addition to experimental investigations, various groups have carried out modelling studies to gain insight into the charge transport features and the structure–property relationship in PCBM24,25, C6026 and related fullerene derivatives.27 For this purpose, a reliable and well-defined structure of solvent-free PCBM is required.
Here we report the preparation of high-quality, solvent-free PCBM crystals obtained via solvent casting deposition from a chlorobenzene solution, and the XRD characterization via the I24 Microfocus Macromolecular Crystallography (MX) beamline at the Diamond Light Source. Our findings will serve as a guide for further experimental and theoretical investigations on PCBM electronic properties.
2 Methods
2.1 Experimental
PCBM was purchased from Aldrich (purity >99%) and used without further purification. The PCBM solution with a concentration of 20 mg ml−1 was obtained by dissolving an appropriate amount of PCBM in chlorobenzene (CB) at room temperature. To ensure the complete dissolution of PCBM, the solution was kept stirring overnight at room temperature (295–298 K) in the dark. We obtained PCBM single crystals by placing a droplet (∼100 μL) of the PCBM solution on 15 mm diameter fused silica glass disks at room temperature and allowing the solvent to evaporate. The samples were kept in a capped Petri dish during the deposition and dried under vacuum (∼10−2 mbar) overnight to remove the residual solvent. The vacuum treatment was carried out at room temperature. All the preparation procedures were carried out in a nitrogen glove-box to prevent degradation of the material.XRD measurements were performed on beamline I24 at the Diamond Light Source. The experiment was carried out using an X-ray beam size of 10 μm × 10 μm with a wavelength of 0.62 Å. The sample was held at a temperature of 100 K. The data were processed using CrysAlis Pro,28 the structure was solved with SHELXS-9729 and refined by means of full-matrix least squares in SHELXL-97.29
2.2 Computation
Molecular dynamics (MD) simulations were carried out for a 3 × 3 × 3 supercell (108 PCBM molecules) using the experimental crystal structure as initial coordinates. Two simulations were run for 100 and 300 K applying periodic boundary conditions. The systems were equilibrated in the NPT ensemble at 1 bar for about 4–11 ns using a Langevin piston with anisotropic pressure rescaling. Thereafter, simulations were carried out in the NVT ensemble at the respective equilibrium densities. Radial distributions and coordination numbers were averaged over 1000 frames taken from a 10 ns MD trajectory in the NVT ensemble. Parameters for bonded and non-bonded interactions were chosen as in ref. 25i.e. OPLS parameter from ref. 30 with C60 bond lengths taken from ref. 31. The only exception is the Lennard-Jones distance parameter for the carbon atoms of the C60 cages, which we increased to σ = 3.832 Å from 3.550 Å in the OPLS force field. The value was adjusted so as to reproduce the experimental density of fcc-C60 at room temperature.32 The integration time step for the MD simulation was 1 fs. The MD simulations were carried out with the NAMD package.333 Results and discussion
Fig. 1 presents the optical microscopy images of the PCBM crystals produced via slow evaporation of the solvent. The best quality crystals were obtained by drop-casting a 20 mg ml−1 CB solution on fused silica glass. To obtain a solvent saturated atmosphere and slow down the solvent evaporation dynamics, the samples were placed in a capped Petri dish and kept overnight. Such a deposition technique, with a reduced solvent evaporation rate, enabled the formation of large PCBM crystalline aggregates with a lateral size of up to 500 μm. The preparation procedure involved the optimization of several parameters including solvent, PCBM concentration and substrate.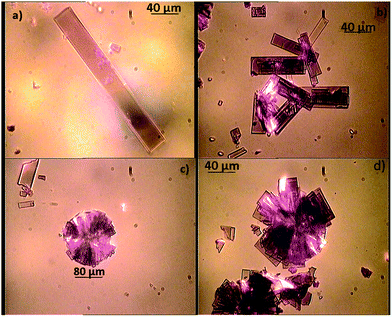 |
| Fig. 1 Optical microscopy images of PCBM crystal solvent cast from CB. | |
The images display the presence of high-quality, rhomboidal-shaped crystals, with sizes ranging from ∼10 μm to 260 μm. In particular, Fig. 1a shows a single PCBM crystal (with a top surface ∼260 × 30 μm2). Some crystals were also found to stack on top of one another (Fig. 1b) or to merge with neighbouring crystals to form round-shaped crystalline aggregates with a radius in the range of ∼40 to 120 μm (Fig. 1c and d). This rectangular prism crystal habit for PCBM seems to be unprecedented, as Rispens21 and Dabirian22 have found different shapes for PCBM single crystals. This might be related to the different substrate used in our experiment and, hence, confirms the important role played by the substrate nature in affecting the PCBM crystallization behaviour.
The XRD micro-focus characterization revealed that PCBM assembles into a monoclinic unit cell containing four PCBM molecules (Table 1 and Fig. 2a and b). Remarkably, this structure does not show any solvent inclusion, in contrast to what was found by Rispens21 and Zheng.23 This is corroborated by the considerably smaller unit cell volume reported here as compared to that reported by Rispens (3708.70 Å3vs. 3984.9 Å3 for the triclinic and 4210.8 Å3 for the monoclinic respectively, although this may partly be due to contraction of the crystals at 100 K, the measurement temperature). In addition, a van der Waals sphere representation (Fig. 2b) clearly indicates that there is not enough space to accommodate a solvent molecule inside the unit cell. Good agreement between the calculated crystallographic model and the experimental XRD data is demonstrated by the relatively low value of the R-factor (5.12%).
Table 1 XRD data collected at the microfocus beamline
Empirical formula | Crystal system | Space group | Unit cell dimension | Volume | Z | Density | Final R indices |
---|
C72H14O2 | Monoclinic | P2(1)/n | Experimental, 100 K, a = 13.47 Å, α = 90°, b = 15.1 Å, β = 106.90°, c = 19.01 Å, γ = 90° | 3708.70 Å3 | 4 | 1.631 g cm−3 | R1= 0.0512, wR2= 0.1503 |
MD, 100 K, a = 13.25, α = 90°, b = 14.77, β = 106.23, c = 19.48, γ = 90° | 3660.35 Å3 | 1.653 g cm−3 |
MD, 300 K, a = 13.29, α = 90°, b = 14.93, β = 106.33, c = 19.48, γ = 90° | 3709.28 Å3 | 1.631 g cm−3 |
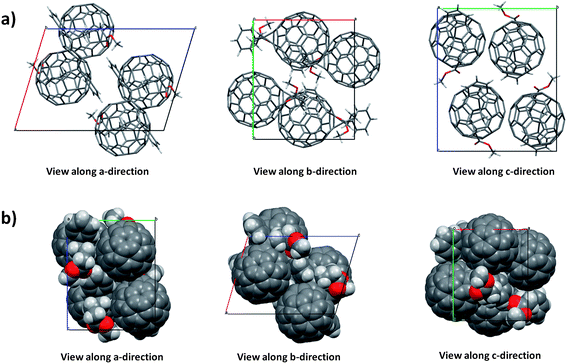 |
| Fig. 2 (a) Monoclinic crystal packing of PCBM along a, b and c directions and (b) van der Waals sphere representation. | |
Classical molecular dynamics simulations of the solvent-free monoclinic PCBM crystal have been carried out for the temperature at which the X-ray structure was solved (100 K) and for room temperature (300 K), see the Methods section for simulation details. The unit cell parameters obtained are summarized in Table 1. We find that the molecular model predicts the equilibrium density at 1 bar and 100 K reasonably well, overestimating the experimental value by only 1.3%. From the finite difference of the simulated volumes at 100 and 300 K we estimate a thermal expansion coefficient of 6.7 × 10−5 K−1, which is similar to the experimental value for C60, 6.2 × 10−5 K−1.32
The thermal fluctuations of the fullerene molecules are characterized in more detail by computing the centre-to-centre radial distribution functions of the C60 cages, shown in Fig. 3. The first peak is centred at 10.05 ± 0.10 Å (100 K) and ranges from 9.55 to 10.55 Å, matching closely the shortest distances in the crystal structure (9.56–10.85 Å, indicated by dashed spikes). The second peak exhibits a shoulder at 12.75 Å and a maximum at 13.25 Å matching again closely the discrete distances in the crystal structure. When the temperature is increased to 300 K the position of the first maximum remains virtually unchanged, and the shoulder at larger distances merges into a single broad peak centred at 12.95 Å. Thus, at room temperature the average nearest neighbour distance in monoclinic PCBM, 10.05 Å, is within statistical errors identical to the distance in fcc-C60 (10.02 Å using a lattice constant of 14.17 Å at 300 K (ref. 32)).
Coordination numbers (CN) were obtained by spherical integration of the radial distributions, shown as insets in Fig. 3. Integration up to the first minimum at 11.0 Å gives a CN of 7.0 and further integration up to the second minimum at 13.8 Å gives a CN of 12.0. As can be seen, the CNs are rather insensitive to temperature in the range of 100–300 K. We note that the nearest neighbour CN (7.0) is higher than previously reported values for monoclinic PCBM which contained solvent impurities (PCBM
:
ODCB = 1
:
1, CN = 6), but similar to the one reported for triclinic PCBM, where the PCBM to solvent ratio was higher (PCBM
:
CB = 2
:
1, CN = 7).21,34
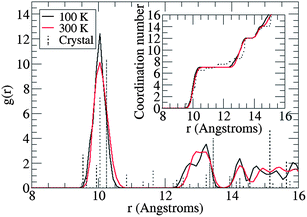 |
| Fig. 3 Radial distribution function of solvent-free monoclinic PCBM as obtained from classical molecular dynamics simulation at 100 and 300 K. The spectra of discrete distances in the X-ray structure are shown as dashed spikes. The distance r is the separation between the centres of mass of the C60 cages of PCBM. Coordination numbers are shown in the inset. | |
4 Conclusion
We have reported the preparation and micro-focused XRD structural characterisation of large and high-quality crystals of PCBM. These provide a much needed experimental validation for computational models that build on such structural information for analysis and prediction of photophysical and charge transport properties in this class of materials. The results are highly relevant to applications such as solar cells, field-effect transistors, and more generally for organic and plastic electronics. While in the final stages of preparation of this manuscript we became aware of similar results obtained by Casalegno and collaborators,20 who also obtained solvent-free crystals but with a slightly different procedure, that involved the use of a different solvent and heating the crystals, instead of simply exposing them to vacuum. Such a concomitant and independent finding that it is indeed possible to prepare solvent-free crystals disproves the long-held and discouraging belief that it is impossible to obtain solvent-free crystals of PCBM. In turn, it fully validates the selection of this material as both an experimental and computational model for charge transport and photophysical studies. Our good agreement between experimental and computed structures, as evidenced by radial distributions and thermal expansion coefficient, suggests that the present atomistic model is especially well-suited for future investigations of electron transport in this important material.Acknowledgements
GMP is supported by an IMPACT PhD studentship co-sponsored by UCL and ISIS- Neutron and Muon Facility (Science and Technology Facilities Council). JS is supported by an IMPACT PhD studentship co-sponsored by University College London and the Department of Physics and Astronomy. JB acknowledges the Royal Society for a University Research Fellowship. The molecular simulations were carried out at the high-performance computing facility HECToR via the Materials Chemistry Consortium, which is funded by the Engineering and Physical Sciences Research Council, EP/F067496. We also thank Professor A. Briseño for helpful discussions.References
- B. Fraboni, C. Femoni, I. Mencarelli, L. Setti, R. Di Pietro, A. Cavallini and A. Fraleoni-Morgera, Adv. Mater., 2009, 21, 1835–1838 CrossRef CAS
. - A. Fraleoni-Morgera, L. Benevoli and B. Fraboni, J. Cryst. Growth, 2010, 312, 3466–3472 CrossRef CAS
. - V. Podzorov, MRS Bull., 2013, 38, 15–27 CrossRef
. - A. L. Briseno, S. C. Mannsfeld, M. M. Ling, S. Liu, R. J. Tseng, C. Reese, M. E. Roberts, Y. Yang, F. Wudl and Z. Bao, Nature, 2006, 444, 913–917 CrossRef CAS
. - A. A. Virkar, S. Mannsfeld, Z. Bao and N. Stingelin, Adv. Mater., 2010, 22, 3857–3875 CrossRef CAS
. - J. L. Brédas, J. Cornil and A. J. Heeger, Adv. Mater., 1996, 8, 447–452 CrossRef
. - A. L. Ayzner, C. J. Tassone, S. H. Tolbert and B. J. Schwartz, J. Phys. Chem. C, 2009, 113, 20050–20060 CAS
. - G. Yu and A. J. Heeger, J. Appl. Phys., 1995, 78, 4510–4515 CrossRef CAS
. - J. J. M. Halls, C. A. Walsh, N. C. Greenham, E. A. Marseglia, R. H. Friend, S. C. Moratti and A. B. Holmes, Nature, 1995, 376, 498–500 CrossRef CAS
. - W. Yin and M. Dadmun, ACS Nano, 2011, 5, 4756–4768 CrossRef CAS
. - X. N. Yang, J. K. J. van Duren, M. T. Rispens, J. C. Hummelen, R. A. J. Janssen, M. A. J. Michels and J. Loos, Adv. Mater., 2004, 16, 802–806 CrossRef CAS
. - H. Hoppe, A. Drees, W. Schwinger, F. Schaffler and N. S. Sariciftci, Synth. Met., 2005, 152, 117–120 CrossRef CAS
. - X. N. Yang, J. K. J. van Duren, R. A. J. Janssen, M. A. J. Michels and J. Loos, Macromolecules, 2004, 37, 2151–2158 CrossRef CAS
. - H. Hoppe, T. Glatzel, M. Niggemann, A. Hinsch, M. Lux-Steiner and N. S. Sariciftci, Nano Lett., 2005, 5, 269–274 CrossRef CAS
. - F. Piersimoni, S. Chambon, K. Vandewal, R. Mens, T. Boonen, A. Gadisa, M. Izquierdo, S. Filippone, B. Ruttens, J. D'Haen, N. Martin, L. Lutsen, D. Vanderzande, P. Adriaensens and J. V. Manca, J. Phys. Chem. C, 2011, 115, 10873–10880 CAS
. - A. Swinnen, I. Haeldermans, M. vande
Ven, J. D'Haen, G. Vanhoyland, S. Aresu, M. D'Olieslaeger and J. Manca, Adv. Funct. Mater., 2006, 16, 760–765 CrossRef CAS
. - M. Reyes-Reyes, R. Lopez-Sandoval, J. Arenas-Alatorre, R. Garibay-Alonso, D. L. Carroll and A. Lastras-Martinez, Thin Solid Films, 2007, 516, 52–57 CrossRef CAS
. - L. Li, G. Lu, S. Li, H. Tang and X. Yang, J. Phys. Chem. B, 2008, 112, 15651–15658 CrossRef CAS
. - M. Dante, J. Peet and T. Q. Nguyen, J. Phys. Chem. C, 2008, 112, 7241–7249 CAS
. - M. Casalegno, S. Zanardi, F. Frigerio, R. Po, C. Carbonera, G. Marra, T. Nicolini, G. Raos and S. Meille, Chem. Commun., 2013, 49, 4525–4527 RSC
. - M. T. Rispens, A. Meetsma, R. Rittberger, C. J. Brabec, N. S. Sariciftci and J. C. Hummelen, Chem. Commun., 2003, 2116–2118 RSC
. - R. Dabirian, X. Feng, L. Ortolani, A. Liscio, V. Morandi, K. Müllen, P. Samorì and V. Palermo, Phys. Chem. Chem. Phys., 2010, 12, 4473–4480 RSC
. - L. Zheng and Y. Han, J. Phys. Chem. B, 2012, 116, 1598–1604 CrossRef CAS
. - F. Gajdos, H. Oberhofer, M. Dupuis and J. Blumberger, J. Phys. Chem. Lett., 2013, 4, 1012–1017 CrossRef CAS
. - D. L. Cheung and A. Troisi, J. Phys. Chem. C, 2010, 114, 20479 CAS
. - H. Oberhofer and J. Blumberger, Phys Chem. Chem. Phys., 2012, 14, 13846 RSC
. - R. C. I. MacKenzie, J. M. Frost and J. Nelson, J. Chem. Phys., 2010, 132, 064904 CrossRef
. - Agilent (2012). CrysAlis Pro. Agilent Technologies, Yarnton, Oxfordshire, England.
- G. M. Sheldrick, Acta Crystallogr. Sect. A, 2008, 64, 112 CrossRef CAS
. - W. L. Jorgensen, D. S. Maxwell and J. Tirado-Rives, J. Am. Chem. Soc., 1996, 118, 11225 CrossRef CAS
. - K. Hedberg, L. Hedberg, D. S. Bethune, C. A. Brown, H. C. Dorn, R. D. Johnson and M. De Vries, Science, 1991, 254, 410 Search PubMed.
- P. A. Heiney, J. Phys. Chem. Solids, 1992, 53, 1333 CrossRef CAS
. - J. C. Phillips, R. Braun, W. Wang, J. Gumbart, E. Tajkhorshid, E. Villa, C. Chipot, R. D. Skeel, L. Kale and K. Schulten, J. Comput. Chem., 2005, 26, 1781 CrossRef CAS
. - F. Frigerio, M. Casalegno, C. Carbonera, T. Nicolini, S. V. Meille and G. Raos, J. Mater. Chem., 2012, 22, 5434 RSC
.
|
This journal is © The Royal Society of Chemistry 2013 |