Structural characterisation of hypoxia-mimicking bioactive glasses†
Received
13th November 2012
, Accepted 10th January 2013
First published on 11th January 2013
Abstract
Nickel and cobalt are both known to stimulate the hypoxia-inducible factor-1 (HIF-1α), thus significantly improving blood vessel formation in tissue engineering applications. We have manufactured nickel and cobalt doped bioactive glasses to act as a controlled delivery mechanism of these ions. The resultant structural consequences have been investigated using the methods of isotopic and isomorphic substitution applied to neutron diffraction. The structural sites present will be intimately related to their release properties in physiological fluids such as plasma and saliva, and hence the bioactivity of the material. Detailed structural knowledge is therefore a prerequisite for optimising material design. Results show that nickel and cobalt adopt a mixed structural role within these bioactive glasses occupying both network-forming (tetrahedral) and network-modifying (5-fold) geometries. Two thirds of the Ni (or Co) occupies a five-fold geometry with the remaining third in a tetrahedral environment. A direct comparison of the primary structural correlations (e.g. Si–O, Ca–O, Na–O and O–Si–O) between the archetypal 45S5 Bioglass® and the Ni and Co glasses studied here reveal no significant differences. This indicates that the addition of Ni (or Co) will have no adverse effects on the existing structure, and thus on in vitro/in vivo dissolution rates and therefore bioactivity of these glasses.
1 Introduction
Vascularisation is essential in wound healing for both soft and hard tissue regeneration. Bio-oxygen levels play an important role in modulating cell functions and tissue repair in vivo. Hypoxia (low oxygen partial pressure) can enhance the production of specific extracellular matrix components and increase blood vessel formation (angiogenesis) through the hypoxia-inducible factor-1 (HIF-1α) pathway.1 For example, HIF-1α over-expressing bone marrow stem cells significantly improve blood vessel formation in bone tissue engineering applications.2 Wan et al. demonstrated that the HIF-1α pathway is activated during bone repair and can be manipulated to improve skeletal healing.3 Reduced oxygen levels have also been shown to stimulate chondrogenesis in stem cells, which is important for cartilage regeneration.4
Transition metals such as Co and Ni have been shown to increase the HIF-1α transcription factor.5 Bioactive glasses offer an exciting route for potential delivery systems of transition metal ions within tissue regeneration scaffolds due to their ability to incorporate a large variety of elements and their largely controllable dissolution properties within physiological fluids.6 Bioglass®, developed by Hench, was developed to provide a controlled release of calcium and phosphorous ions under physiological conditions.7 These ions then precipitate into amorphous calcium phosphate which later crystallise into hydroxyapatite to form new bone mineral.8–10 In addition to providing the fundamental building blocks of bone (Ca and P) the dissolution products of bioglass were also found to stimulate osteoblast activity.11,12 More recently bioactive glasses have been developed to provide a controlled release mechanism for antimicrobial ions such as Ag and Ga to combat infections.13,14
In order to be able to model and predict the behaviour of bioactive glasses in situ it is important to have an understanding of the atomic scale structure and any modifications that may result due to the incorporation of additional elements. However, the structure of bioglass is particularly complex owing to the addition of two network modifiers (Na and Ca) into a glass composed of two network formers (Si and P). Significant progress has now been made on unravelling the atomic scale structure of archetypal 45S5 Bioglass through a series of molecular dynamic simulations,15–17 nuclear magnetic resonance18–20 and neutron diffraction studies.21–24 CaO and Na2O both adopt network modifier roles, breaking Si–O–Si bonds through the addition of oxygen. Other elements such as Zn, Mg, Ga and Al have been found to act as network intermediates where they can act as network formers, network modifiers or a mixture of both network formers and modifiers.25,26
The addition of a transition metal further complicates the picture as it is not possible to predict a priori the structural consequences of their incorporation into bioactive glasses. Cobalt, for example, could adopt various structural arrangements, as discussed by Azevedo et al., including entering the silicate network as four-fold Co–O or acting as a network modifier with a resultant higher coordination number up to possibly eight-fold Co–O.27 The role of cobalt (or Ni) in the glass network will determine the network connectivity (NC) and macroscopic properties, such as ion release rates and hydroxyapatite formation. Cobalt and nickel both have similar ionic radii and charge states that also closely match magnesium. According to Shannon, the ionic radii of four-fold Ni, Co and Mg are 0.58, 0.55 and 0.57 Å respectively.28 Given the similar ionic radii and therefore similar charge to size ratio it may be expected that Co and Ni will adopt a similar structural role to that of Mg, which has been shown to act as a network intermediate.25 Furthermore, Na+ and Ca2+ ions both show an affinity for phosphate species over silicates;29 again, it is difficult to predict the most energetically favourable environment of transition metals and their preferred structure.
Ultimately, the resultant structural influence of the transition metals may determine which applications these glasses are most suitable for (e.g. soft or hard tissue regeneration). For example, replacing Ca with Sr within the glass leads to an expanded matrix which results in an increased solubility and an increased rate of hydroxyapatite formation, which is highly desirable for bone regeneration applications.30 Conversely, replacing Ca with Mg within the glass leads to a contracted matrix and decreased rate of HA formation.25 For soft tissue applications such as cartilage regeneration a reduction in HA formation is desirable. Importantly, the local environment surrounding the transition metal will strongly influence its rate of dissolution from the glass. A fast release of transition metals will result in a high local concentration, which is known to cause cell toxicity.31 A controlled release rate, appropriate to enhanced angiogenesis, is therefore vital for any tissue engineering application.
It is important that detailed experimental studies are conducted to elucidate the structural consequences of incorporating transition metals into bioactive glasses. We report here on a detailed investigation of the structural effects of incorporating Ni and Co into bioactive glasses using advanced neutron diffraction techniques. 4 mol% NiO (or Co) was incorporated into bioglass to provide physiologically appropriate concentrations.27,32 Cobalt is cytotoxic in high concentrations however Wu et al. have shown that bioactive scaffolds containing less than 5 mol% CoO are not cytotoxic.32 The low concentration of transition metals incorporated in these glasses means it is not possible to extract structural information from a single total (i.e. conventional) diffraction pattern. By employing the method of isotopic substitution applied to neutron diffraction we have isolated the nickel correlations, providing definitive quantitative structural information on the local environment surrounding the nickel ions. In addition, by applying the method of isomorphic substitution between Co and Ni bioactive glasses, we have been able to confirm that Co adopts the same structural environment within these glasses.
2 Experimental
Sample preparation
Melt-quenched glass samples were prepared using SiO2 (Alfa Aesar, 99.5%), CaCO3 (Alfa Aesar, 99.95–100.5%) and Na2CO3 (Sigma-Aldrich, 99.5+%), NH4H2PO4 (Sigma-Aldrich, ≥99.5%), and the selected transition metal. The isotopically enriched Ni doped bioactive glasses were prepared using metallic 99.5% enriched 58Ni, natNi and 98% enriched 62Ni (ISOFLEX) respectively. Metallic Ni oxidises during the heating process to form nickel(II) oxide; a control sample was also prepared using natNiO and no difference in the XANES spectra or density measurements could be observed between samples prepared using metallic or oxide nickel. Cobalt doped bioactive glasses were prepared CoO (Alfa Aesar, 99.995%). The ratio of precursors was selected to yield glasses with compositions of Bioglass® 45S5 + 4 mol% transition metal oxide. The precursors, after thorough mixing, were heated in a platinum–rhodium (10%) crucible at a rate of 10 °C min−1 to 1450 °C and held at this temperature for 90 minutes. The melt was poured into a graphite mould which had been preheated to 450 °C and annealed at this temperature overnight before being allowed to cool slowly to room temperature. The resultant glass compositions were (MO)3.8(SiO2)44.3(CaO)25.9(Na2O)23.5(P2O5)2.5, where M represents the transition metal Ni or Co.
Density measurements
Density measurements were taken using a Quantachrome pycnometer using Archemedes' method with helium being used as the fluid.
Measurements were undertaken on the B18 instrument at the Diamond Light Source, Harwell Research Campus. Finely ground samples were diluted in cellulose and pressed into pellets to give a satisfactory K-edge absorption step. The spectra were recorded at room temperature and in transmission mode. The incident beam intensity and the transmitted beam intensity were measured using ionization chambers. The Ifeffit software analysis suite was used for the background corrections and normalisation (Athena).33 The data analysis was undertaken using pyspline.34 In brief, the data processing comprised calibrating the energy scale, normalizing the incident beam intensity and the spectra to provide an edge step of one. The data modelling was undertaken using EXCURV.35
Neutron diffraction
The neutron data was collected at the D4 diffraction instrument at the Institut Laue-Langvin, Grenoble.36 Calibration of the incident neutron wavelength was achieved using a polycrystalline nickel reference sample. The samples were ground into course lumps and contained in vanadium cans of internal diameter 6 mm and wall thickness 0.25 mm; a vanadium rod of diameter 6 mm was used to provide quantitative normalisation to the data. Data were collected from the empty instrument, empty vanadium container and the vanadium rod in addition to each of the samples. Structural information can be obtained from the data by removing the background scattering (from the instrument itself and from the container) and correcting for absorption and multiple scattering to find the differential cross-section using the program CORRECT.37,38
The total structure factor is given by39
| i(Q) = ∑i∑jcicjbibj[ρij(Q) − 1] | (1) |
where
ci and
bi denote the atomic fraction and coherent scattering length of the chemical species
i,
ρij(
Q) is the partial structure factor and
Q is the magnitude of the scattering vector given by
Q = 4πsin(
θ)/
λ where 2
θ is the scattering angle and
λ is the incident
neutron wavelength. These functions of
Q are referred to as reciprocal space data. The corresponding real-space data is obtained by Fourier transforming the total structure factor to give
T(
r), where
|  | (2) |
M(Q) is the window function that takes into account the finite experimentally obtainable maximum value of Q, in this case the quality of the data was sufficient to permit the use of a step function. T0(r) is the average density term, given by:39
| T0(r) = 4πrρ0(∑icibi)2 | (3) |
where
ρ0 is the macroscopic number density. The pair distribution function that results from a neutron diffraction experiment,
T(
r), can be represented as the weighted sum of partial correlation functions,
tij(
r).
| T(r) = ∑i,jcicjbibjtij(r) | (4) |
Examining eqn (4), it can be seen that, if samples are prepared that are identical except for the scattering length of one element, differences between the neutron scattering data from these samples will isolate the partial correlation functions. Since different isotopes (or isomorphs) of an element, have different neutron scattering lengths, isotopic (or isomorphic) substitution provides a method for achieving this separation. In the work presented here the scattering length of the nickel isotope was varied between a set of otherwise identical samples allowing isotopic substitution. Nickel was exchanged for cobalt to allow the isomorphic substitution. The difference in real space can be represented in terms of the partial pair correlation function, TM–j(r), such that only the environment of element M is probed.
| TM–j(r) = T(r) − T′(r) = cM2(bM2 − bM′2)t′MM(r) + 2∑j≠McMcjbj(bM − b′A)t′Mj(r) | (5) |
Structural information can be obtained by modelling the real-space correlation function. Pair functions, pij(Q), are generated in Q-space and Fourier transformed to allow comparison with the experimental data in real space. The pair functions are given by:13,40
| 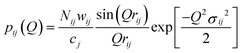 | (6) |
where
Nij,
rij, and
σij are the coordination number, atomic separation and disorder parameter (
i.e., a measure of static and thermal disorder) respectively of atom
i in relation to atom
j. The weighting factors
wij for a standard neutron diffraction experiment are given by:
In the case of the isomorphic and isotopic difference the weighting factors take the form:
| wi≠j = 2cicj(bM − b′M)bj | (9) |
| wi=j = ci2(bM2 − bM′2) | (10) |
In this case atom M is the transition metal, either nickel or cobalt. Nickel has several naturally occurring stable isotopes: 58Ni has a neutron scattering length, b, of 14.4 fm, whereas 62Ni has b = −8.7 fm, natNi has b = 10.3 fm and Co has b = 2.49 fm.41
3 Results and discussion
Bioactive glasses containing 3.8 mol% NiO or CoO were prepared. Three Ni samples were prepared: one containing natural abundance Ni, a second containing nickel enriched with 58Ni and a third enriched with 62Ni. The coherent scattering lengths of the nickel within the 58Ni-, natNi- and 62Ni-enriched glasses, allowing for the levels of isotopic enrichment, are 14.3, 10.3 and −8.4 fm respectively. The negative scattering lengths arise from a π phase shift of the neutron wave function on scattering. Glass samples are hereafter labelled natNi, 58Ni and 62Ni respectively. The densities of all the Ni and Co doped glasses were 2.83(1) g cm−3 with a corresponding number density of 0.0769(2) atoms per cubic Angström. Extended X-ray Absorption Fine Structure (EXAFS) data showed no differences between the local Ni–O environments for each of the Ni doped glasses (see ESI, Fig. S1†), thus further confirming that all of the samples were iso-structural.
The total interference functions, i(Q), for 58Ni, natNi and 62Ni are shown in Fig. 1. The real space data obtained by Fourier transforming the total interference functions is given in Fig. 2. It is apparent that the real-space datasets are qualitative similar e.g. the P–O and Si–O peaks at ∼1.6 Å and the Na–O, Ca–O and O–(P)–O, O–(Si)–O peaks around 2.3 to 2.7 Å. The main difference visible in the total diffraction patterns, T(r), occurs in the region around 2 Å where the Ni–O correlation is predicted to occur.42,43 However, the relatively small weighting factor of the Ni–O correlation (due to the low atomic concentration present) coupled with the overlapping correlation functions makes it difficult unambiguously to fit the Ni–O correlation solely from the total diffraction pattern. Those correlations not involving Ni can be eliminated by subtracting two total structure factors to give a first order difference function such as Δi(Q)58–62 = i(Q)58Ni − i(Q)62Ni (see Table S1 of the ESI†). The addition of the third total structure factor enables three separate first order difference functions to be determined (Δi(Q)58–62, Δi(Q)58–nat and Δi(Q)nat–62) in order to check the reliability/mutual consistency of the derived datasets. Fig. 3 presents the three isotopic substitution difference functions in reciprocal space, whilst Fig. 4 shows the real space pairwise correlation data ΔT(r) obtained by Fourier transforming the Δi(Q) functions given in Fig. 3. Those correlations not containing Ni successfully cancel, as demonstrated by the absence of the P–O and Si–O peaks at ∼1.6 Å. The main feature at ∼2 Å correspond to nearest neighbour Ni–O correlations. The broad feature at ∼3 < r (Å) < 3.5 corresponds to next nearest neighbours correlations such as Ni–(O)–Si, Ni–(O)–P, Ni–(O)–Na and Ni–(O)–Ca; it is not possible unambiguously to resolve these overlapping correlations. As shown in Fig. 4, ΔT(r)58–62 has the largest difference in Ni scattering lengths (22.7 fm) and correspondingly results in best signal-to-noise ratio, ΔT(r)nat–62 has a difference in scattering length of 18.7 fm whilst ΔT(r)58–nat is the least well defined with a scattering length difference of only 4.0 fm. The diffraction data was modelled using the NXFit program developed by Moss40 and the NDIS-derived fit parameters obtained for each of the first order difference functions are given in Table 1 together with the average values obtained by calculating an average of the ΔT(r) functions, weighted by scattering length difference.
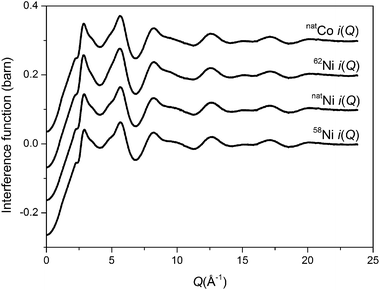 |
| Fig. 1 Total interference functions for the Ni and Co doped bioactive glasses. | |
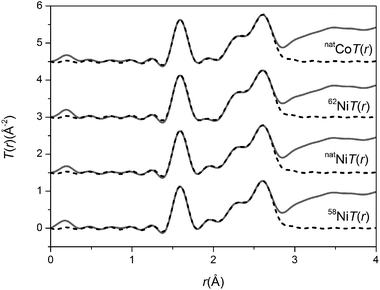 |
| Fig. 2 Real space data for the total diffraction patterns. The solid curve represents the experimental data and the broken curve represents the resultant fits. | |
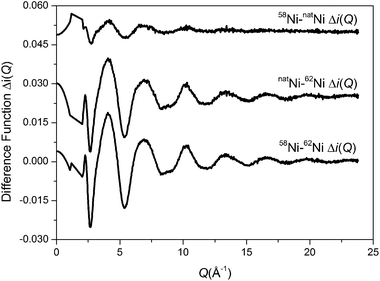 |
| Fig. 3 First order difference isotopic substitution functions Δi(Q). | |
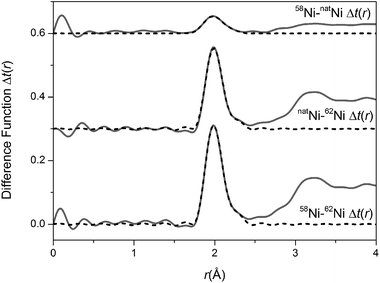 |
| Fig. 4 Real space data for the isotopic substitution difference functions. The solid curve represents the experimental data and the broken curve represents the resultant fits. | |
Table 1 First order difference fit parameters. Typical uncertainties for the distance, coordination number and width parameters are 0.02 Å, 0.05 and 0.05 Å respectively. Note that the error bars associated with the 58Ni–natNi correlation are significantly larger due to the lower signal to noise ratio as discussed in the text
|
Correlation |
r (Å) |
N
|
σ (Å) |
58Ni–62Ni |
Ni–O |
1.98 |
3.85 |
0.09 |
Ni–O |
2.19 |
0.81 |
0.10 |
natNi–62Ni |
Ni–O |
1.99 |
3.87 |
0.09 |
Ni–O |
2.20 |
0.68 |
0.10 |
58Ni–natNi |
Ni–O |
1.96 |
4.00 |
0.10 |
Ni–O |
2.15 |
1.27 |
0.10 |
Average (isotopic) |
Ni–O |
1.98 |
3.87 |
0.09 |
Ni–O |
2.19 |
0.80 |
0.10 |
Average (isomorphic) |
M–O |
1.99 |
3.86 |
0.09 |
M–O |
2.18 |
0.66 |
0.10 |
As shown, the average number of oxygen atoms around a given Ni atom is 4.67 ± 0.05. This is attributed to ∼33% of Ni occupying a 4-fold geometry with the remaining ∼67% occupying a 5-fold environment. The correlation around 2 Å is attributed partially to the 4-fold Ni–O and partially to 5-fold Ni–O where there are 4 short bonds (1.98 Å) and one longer bond at 2.19 Å. This places a lower limit of 33% of Ni occupying a tetrahedral (network forming) 4-fold geometry. If a small amount of Ni resides in a higher coordination geometry (i.e. octahedral) the fraction of tetrahedral Ni will increase accordingly. The results do not preclude the possibility of a tiny fraction of Ni occupying a six fold. However, whilst octahedral Ni is common in crystalline materials it is rarely found in oxide silicate glasses.44 Furthermore, 6-fold Ni–O typically exhibits a distinctive bright green colour which was not visible for the current glasses.44 Galoisy and Calas have reported 5-fold Ni in a trigonal bipyramidal environment with minor amounts of four-fold Ni in CaNiSi2O6 glass.42 In contrast they found predominantly 4-four Ni in glassy K2NiSi3O8, whilst Na2NiSi3O8 was found to have a mixture of 4- and 5-fold Ni.43 They therefore concluded that the nickel environment is strongly dependent on the nature of alkali present. Brendebach et al. report that nickel occupies a predominantly octahedral environment in sodium phosphate systems with a Ni–O distance ∼2.06 Å.45 In the present system there is a relatively small fraction of phosphorus compared to silicon and Ni is found to adopt a predominantly 4- and 5-fold environment. This suggests that Ni does not show a particularly strong affinity for the phosphate species present within this glassy system.
Given that Ni has a similar charge: ion size ratio as Mg it would be reasonable to expect it to adopt a similar structural chemistry. However, the present data suggests that a minimum of 33% of Ni is 4-fold and therefore acting as a network former; this is significantly greater than the 14% Watts et al. reported for magnesium and suggests that there are important differences that were not predicted.25 Bioglass doped with 3.8% NiO (or 1.37% metallic Ni) has a number density of 0.0769 atoms per Å3 compared to 0.0757 atoms per Å3 for 45S5 Bioglass. This represents a 1.6% increase in number density and also suggests that a proportion of Ni may act as a network former. Tetrahedral Ni will have shorter Ni–O bond lengths compared to higher coordinated Ni environments and would therefore be more likely to increase the number density.
Applying the method of isomorphic substitution46,47 enables the local structural environment surrounding Co to be determined in a broadly analogous manner. The data allows three separate first order difference isomorphic substitution functions to be created (Δi(Q)58Ni–Co, Δi(Q)natNi–Co and Δi(Q)Co–62Ni) as shown in Fig. 5. The corresponding real-space, ΔT(r), functions are shown in Fig. 6. The difference in Ni/Co coherent scattering factors are 11.81, 7.81 and 10.89 for the ΔT(r)58Ni–Co, ΔT(r)natNi–Co and ΔT(r)Co–62Ni functions respectively. The optimum (weighted average) fit parameters for the isomorphic difference function are given in Table 1. As shown in Table 1, there is excellent agreement between the metal–oxygen, M–O, correlations obtained for Ni using the method of isotopic substitution and the Co/Ni datasets obtained using the method of isomorphic substitution. This confirms that Co and Ni are isostructural within these bioactive glasses.
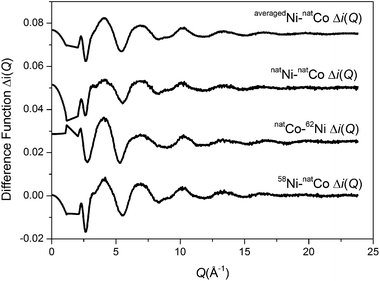 |
| Fig. 5 First order difference isomorphic substitution functions Δi(Q). | |
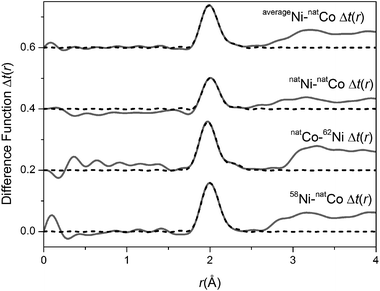 |
| Fig. 6 Real space data for the isomorphic substitution difference functions. The solid curve represents the experimental data and the broken curve represents the resultant fits. | |
The total diffraction patterns
The total diffraction patterns for the Ni and Co doped bioglasses were modelled using the NXFit program and the NDIS-derived fit parameters are given in Table 2. The results of a Ni/Co-free bioactive glass, prepared using an identical melt quench method, are also presented in Table 2 thus enabling a direct comparison of the present results with the archetypal 45S5 Bioglass®.22 The 45S5 results were collected using the ISIS pulsed neutron source and full details of the data analysis are given by Martin et al.22 To enable a direct comparison with the present data the reciprocal data was truncated at a Qmax of 23.7 Å (to match the present Ni (or Co) bioglass data) before Fourier transforming into real-space and fitting using the NXFit analysis program.
Table 2 Structural parameters for the Ni and Co doped bioactive glasses obtained using the NXFit fitting routine. The parameters for 45S5 Bioglass T(r) are included for comparison.22 Typical errors are ±0.02 Å on the peak position (rij), ±0.2 on the coordination number (Nij) and ±0.02 Å on the disorder parameter (σij)
|
58Ni |
natNi |
62Ni |
Co |
Bioglass® 45S5 |
r (Å) |
N
|
σ (Å) |
r (Å) |
N
|
σ (Å) |
r (Å) |
N
|
σ (Å) |
r (Å) |
N
|
σ (Å) |
r (Å) |
N
|
σ (Å) |
P–O |
1.57 |
4.0 |
0.02 |
1.57 |
4.0 |
0.01 |
1.58 |
4.0 |
0.03 |
1.57 |
4.0 |
0.03 |
1.60 |
4.0 |
0.01 |
Si–O |
1.60 |
4.0 |
0.07 |
1.60 |
4.0 |
0.07 |
1.60 |
4.0 |
0.07 |
1.60 |
4.0 |
0.07 |
1.62 |
4.0 |
0.08 |
Ni–O |
1.98 |
3.9 |
0.09 |
1.98 |
3.9 |
0.09 |
1.98 |
3.9 |
0.09 |
1.98 |
3.9 |
0.09 |
— |
— |
— |
Ni–O |
2.19 |
0.8 |
0.10 |
2.19 |
0.8 |
0.10 |
2.19 |
0.8 |
0.10 |
2.19 |
0.8 |
0.10 |
— |
— |
— |
Na–O |
2.34 |
3.1 |
0.14 |
2.33 |
3.1 |
0.13 |
2.32 |
3.1 |
0.13 |
2.32 |
3.1 |
0.13 |
2.33 |
3.1 |
0.15 |
Ca–O |
2.30 |
5.3 |
0.11 |
2.31 |
5.3 |
0.12 |
2.32 |
5.3 |
0.12 |
2.31 |
5.3 |
0.12 |
2.35 |
5.3 |
0.11 |
Na–O |
2.64 |
1.6 |
0.13 |
2.66 |
1.6 |
0.15 |
2.64 |
1.6 |
0.14 |
2.65 |
1.6 |
0.13 |
2.63 |
1.6 |
0.10 |
O–O |
2.61 |
3.9 |
0.10 |
2.61 |
4.0 |
0.10 |
2.61 |
4.0 |
0.10 |
2.61 |
4.0 |
0.10 |
2.65 |
4.0 |
0.11 |
Ca–O |
2.76 |
1.3 |
0.20 |
2.71 |
1.3 |
0.22 |
2.73 |
1.3 |
0.22 |
2.73 |
1.3 |
0.20 |
2.76 |
1.3 |
0.20 |
As expected, the short range order comprises ∼4 oxygen atoms around Si at 1.6 Å48 and ∼4 oxygen around P at a separation between 1.5 and 1.6 Å.49,50 The M–O correlations account for the region 2 < r (Å) < 2.2 as determined from the first order difference functions discussed above. The intermediate range order consists of overlapping Na–O, Ca–O, O–(P)–O and O–(Si)–O correlations and it is therefore not usually possible to resolve these unambiguously. Recently, by employing the method of isomorphic substitution, the Na–O22 and Ca–O23 correlations have been independently resolved in very similar glasses and a model has been proposed for the O–(X)–O correlation (where X = Si or P). These results provide an excellent starting point to begin modelling the overlapping correlations in the present data. The Ca–O feature was therefore modelled using two Ca–O distances based on recently obtained results for an analogous Ca/Sr bioglass system.23 The values, determined for the Ni/Co bioactive glass system, of 5.3(1) oxygen atoms around Ca at 2.31(2) Å and 1.3(1) oxygen atoms around Ca at 2.73(3) Å are in good agreement with the previous reported values of 5.3 oxygen around Ca at 2.35 Å and 1.3 oxygen around Ca at 2.76 Å.22 The Na–O feature was also modelled using two Na–O distances based on results recently obtained by the method of isomorphic substitution of a mixed Na/Li bioglass.22 An asymmetric Na–O peak has been reported both experimentally in sodium silicates and in molecular dynamic simulations on sodium calcium silicates and on Bioglass®.22,51,52 The Na–O values of 3.1 oxygen around Na at 2.33(1) Å and 1.6 oxygen around Na at ∼2.65(2) Å obtained in this study are consistent with these results.22 The first real space O⋯O correlation is expected to be dominated by O–(Si)–O correlations given that the concentration of Si is ∼10 times greater than the concentration of P, and other O⋯O correlations such as O–(Ca)–O and O–(Na)–O are expected to occur at longer real space distances.21 Given that Si is tetrahedrally coordinated, the expected O–(Si)–O distance is given by
. The O–(Si)–O distance of 2.61 Å given in Table 1 is therefore in good agreement given the reported Si–O distance of ∼1.6 Å. The O–(Si)–O coordination number also confirms that the network connectivity of the glass is largely unaffected by the addition of Ni or Co into these glasses, noting that ortho-silicates have an O–(Si)–O coordination number of 3 whilst a fully densified silica network would have an O–(Si)–O coordination number of 6.
Knowing the fraction of Ni (or Co) that acts as a network former enables the network connectivity of the glass to be calculated. If the network connectivity is too low, the glass dissolves too quickly and hydroxypatite does not have time to form – i.e. the bioactivity is effectively reduced. Conversely, if the network connectivity is too high, the glass does not release sufficient Ca and P for hydroxyapatite to form – reducing the bioactivity again. The network connectivity, NC, of a silicate glass is given by
with 4 representing a fully densified
silica network and
Z representing the number of excess oxygen per SiO
2. It therefore follows that the addition of NiO (or CoO) into a glass could either decrease the network connectivity (due to the additional oxygen incorporated) or increase the network connectivity if the metal (Ni or Co) adopts a network forming role. Assuming only 4-fold Ni (or Co) acts as a network formed then
| 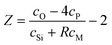 | (12) |
where
R is the fraction of the metal ions occupying a tetrahedral (network forming) environment. The phosphorus term takes into consideration its isolated orthophosphate environment where it binds to 4 oxygen atoms per phosphorus effectively removing these oxygen atoms from the network.
For the present system, given that 33% of Ni (or Co) occupies a network forming (tetrahedral) environment, it therefore follows that the network connectivity is 2.11 ± 0.02. This is very close to the connectivity required for optimal bioactivity and matches the network connectivity of Bioglass®. Hill recommends a network connectivity close to 2.0 for optimal bioactivity53 whilst Watts et al. also note that all the very bioactive glasses have a network connectivity close to 2.0.25 Edén has shown that when the glass network becomes defragmented bioactivity decreases.54 The time taken to form hydroxy-carbon apatite increased for glasses with connectivities of 2.01 and 1.97 compared to Bioglass (2.11). For the present system if the Ni (or Co) entered entirely as a network modifier the network connectivity would have fallen below these values, this suggests that the formation of 4-fold Ni (or Co) is important for maintaining optimal bioactivity. Currently, when designing Mg or Co glasses, multiple series are often manufactured based on the assumption that the metal ions act either entirely as network former or entirely as a network modifier.25,27 Knowledge of the local environment of the Co and Ni ions will enable these systems to be better modeled. Furthermore, with this knowledge, and using the above network connectivity model, it is now possible to alter the network modifier concentration in a controlled fashion in order to compensate for the effects of Ni or Co and thus design bioactive glasses with optimal network connectivities. However, further work is still needed to determine the in vitro and in vivo properties of these materials.
4 Conclusions
Co and Ni doped bioactive glasses have been successfully manufactured. This is, to the best of our knowledge, the first time a bioactive glass containing Ni has been synthesised. Using the methods of isotopic and isomorphic substitution we have shown that Ni and Co adopt the same structural environment within these bioactive glasses. The cobalt and nickel-doped Bioglass samples reported here, represent one of the most complex amorphous materials (6 chemical elements and therefore 21 partial structure factors) and the lowest level of isomorphic enrichment (1.37 mol% Ni/Co) ever studied using isomorphic substitution for an amorphous system. The results confirm that Ni and Co act as network intermediates with a significant fraction adopting a four-fold symmetry, thereby entering the network. Importantly, there is no measureable difference in the remaining structure correlations (e.g. Si–O, P–O, Ca–O, Na–O and O–Si–O) between the archetypal 45S5 Bioglass® and these bioactive glasses doped with Ni or Co, indicating that the addition of these transition metals should not adversely affect the overall bioactivity or existing structural models. The data provided above will enable Ni and Co doped bioactive glasses to be rationally designed and optimised in the future according to network connectivity, dissolution and bioactivity requirements.
Acknowledgements
The authors wish to thank the Institut Laue-Langevin, ILL, for the allocation of beam-time. The work was part-funded by an EPSRC Project Studentship EP/F021011/1, and through the STFC's support of the ILL.
References
- J. Malda, T. J. Klein and Z. Upton, Tissue Eng., 2007, 13, 2153 CrossRef CAS.
- D. Zou, Z. Zhang, J. He, K. Zhang, D. Ye, W. Han, J. Zhou, Y. Wang, Q. Li, X. Liu, X. Zhang, S. Wang, J. Hu, C. Zhu, W. Zhang, Y. Zhou, H. Fu, Y. Huang and X. Jiang, Biomaterials, 2012, 33, 2097 CrossRef CAS.
- C. Wan, S. R. Gilbert, Y. Wang, X. Cao, X. Shen, G. Ramaswamy, K. A. Jacobsen, Z. S. Alaql, A. W. Eberhardt, L. C. Gerstenfeld, T. A. Einhorn, L. Deng and T. L. Clemens, Proc. Natl. Acad. Sci. U. S. A., 2008, 105, 686 CrossRef CAS.
- H. Abdollahi, L. J. Harris, P. Zhang, S. McIlhenny, V. Srinivas, T. Tulenko and P. J. DiMuzio, J. Surg. Res., 2011, 165, 112 CrossRef CAS.
- Q. Ke and M. Costa, Mol. Pharmacol., 2006, 70, 1469 CrossRef CAS.
- J. R. Jones, P. Sepulveda and L. L. Hench, J. Biomed. Mater. Res., 2001, 58, 720 CrossRef CAS.
- L. L. Hench, J. Mater. Sci.: Mater. Med., 2006, 17, 967 CrossRef CAS.
- V. FitzGerald, R. A. Martin, J. R. Jones, D. Qiu, K. M. Wetherall, R. M. Moss and R. J. Newport, J. Biomed. Mater. Res., Part A, 2009, 91, 76 CrossRef CAS.
- R. A. Martin, H. Twyman, D. Qiu, J. C. Knowles and R. J. Newport, J. Mater. Sci.: Mater. Med., 2009, 20, 883 CrossRef CAS.
- R. A. Martin, S. Yue, J. V. Hanna, P. D. Lee, R. J. Newport, M. E. Smith and J. R. Jones, Philos. Trans. R. Soc. London, Ser. A, 2012, 370, 1422 CrossRef CAS.
- I. D. Xynos, A. J. Edgar, L. D. K. Buttery, L. L. Hench and J. M. Polak, Biochem. Biophys. Res. Commun., 2000, 276, 461 CrossRef CAS.
- I. D. Xynos, A. J. Edgar, L. D. K. Buttery, L. L. Hench and J. M. Polak, J. Biomed. Mater. Res., 2001, 55, 151 CrossRef CAS.
- R. M. Moss, D. M. Pickup, I. Ahmed, J. C. Knowles, M. E. Smith and R. J. Newport, Adv. Funct. Mater., 2008, 18, 634 CrossRef CAS.
- S. P. Valappil, D. Ready, E. A. Abou Neel, D. M. Pickup, W. Chrzanowski, L. A. O'Dell, R. J. Newport, M. E. Smith, M. Wilson and J. C. Knowles, Adv. Funct. Mater., 2008, 18, 732 CrossRef CAS.
- A. Tilocca, J. Mater. Chem., 2010, 20, 6848 RSC.
- A. Tilocca, A. N. Cormack and N. H. de Leeuw, Chem. Mater., 2007, 19, 95 CrossRef CAS.
- Y. Xiang and J. Du, Chem. Mater., 2011, 23, 2703 CrossRef CAS.
- A. Pedone, T. Charpentier, G. Malavasi and M. C. Menziani, Chem. Mater., 2010, 22, 5644 CrossRef CAS.
- A. Pedone, G. Malavasi and M. C. Menziani, J. Phys. Chem. C, 2009, 113, 15723 CAS.
- K. Fujikura, N. Karpukhina, T. Kasuga, D. S. Brauer, R. G. Hill and R. V. Law, J. Mater. Chem., 2012, 22, 7395 RSC.
- V. FitzGerald, D. M. Pickup, D. Greenspan, G. Sarkar, J. J. Fitzgerald, K. M. Wetherall, R. M. Moss, J. R. Jones and R. J. Newport, Adv. Funct. Mater., 2007, 17, 3746 CrossRef CAS.
- R. A. Martin, H. L. Twyman, G. J. Rees, J. M. Smith, E. R. Barney, M. E. Smith, J. V. Hanna and R. J. Newport, Phys. Chem. Chem. Phys., 2012, 14, 12105 RSC.
- R. A. Martin, H. L. Twyman, G. J. Rees, E. R. Barney, R. M. Moss, J. M. Smith, R. G. Hill, G. Cibin, T. Charpentier, M. E. Smith, J. V. Hanna and R. J. Newport, J. Mater. Chem., 2012, 22, 22212 RSC.
- R. A. Martin, R. M. Moss, N. J. Lakhkar, J. Knowles, G. J. Cuello, M. E. Smith, J. V. Hanna and R. J. Newport, Phys. Chem. Chem. Phys., 2012, 14, 15807 RSC.
- S. J. Watts, R. G. Hill, M. D. O'Donnell and R. V. Law, J. Non-Cryst. Solids, 2010, 356, 517 CrossRef CAS.
- R. A. Martin, P. S. Salmon, D. L. Carroll, M. E. Smith and A. C. Hannon, J. Phys.: Condens. Matter, 2008, 20, 115204 CrossRef.
- M. M. Azevedo, G. Jell, M. D. O'Donnell, R. V. Law, R. G. Hill and M. M. Stevens, J. Mater. Chem., 2010, 20, 8854 RSC.
- R. Shannon, Acta Crystallogr., Sect. A: Cryst. Phys., Diffr., Theor. Gen. Crystallogr., 1976, 32, 751 CrossRef.
- A. Tilocca and A. N. Cormack, J. Phys. Chem. B, 2007, 111, 14256 CrossRef CAS.
- Y. C. Fredholm, N. Karpukhina, D. S. Brauer, J. R. Jones, R. V. Law and R. G. Hill, J. R. Soc. Interface, 2012, 9, 880 CrossRef CAS.
- I. Catelas, A. Petit, H. Vali, C. Fragiskatos, R. Meilleur, D. J. Zukor, J. Antoniou and O. L. Huk, Biomaterials, 2005, 26, 2441 CrossRef CAS.
- C. Wu, Y. Zhou, W. Fan, P. Han, J. Chang, J. Yuen, M. Zhang and Y. Xiao, Biomaterials, 2012, 33, 2076 CrossRef CAS.
- B. Ravel and M. Newville, J. Synchrotron Radiat., 2005, 12, 537 CrossRef CAS.
-
A. Tenderholt, B. Hedman and K. O. Hodgson, PySpline: a modern, cross-platform program for the processing of raw averaged XAS edge and EXAFS data, in X-ray absorption fine structure-XAFS13, ed. B. Hedman and P. Painetta, 2007, vol. 882, p. 105 Search PubMed.
- S. J. Gurman, N. Binsted and I. Ross, J. Phys. C: Solid State Phys., 1984, 17, 143 CrossRef CAS.
- H. E. Fischer, P. Palleau and D. Feltin, Phys. B, 2000, 276, 93 CrossRef.
-
M. A. Howe, R. L. McGreevy and P. Zetterstrom, Computer Code CORRECT: Correction Program for Neutron Diffraction Data NFL Studsvik, 1996 Search PubMed.
- G. J. Cuello, J. Phys.: Condens. Matter, 2008, 20, 244109 CrossRef.
-
P. H. Gaskell, Glasses and Amorphous Materials, Materials Science and Technology, 9th edn, 1991, p. 175 Search PubMed.
-
R. M. Moss, PhD thesis, University of Kent, 2009.
- V. F. Sears, Neutron News, 1992, 3, 26 CrossRef.
- L. Galoisy and G. Calas, Am. Mineral., 1991, 76, 1777 CAS.
- L. Galoisy and G. Calas, Am. Mineral., 1992, 77, 677 CAS.
- L. Galoisy, Elements, 2006, 2, 293 CrossRef CAS.
- B. Brendebach, R. Glaum, M. Funke, F. Reinauer, J. Hormes and H. Modrow, Z. Naturforsch. A, 2005, 60A, 449 Search PubMed.
- R. A. Martin, P. S. Salmon, C. J. Benmore, H. E. Fischer and G. J. Cuello, Phys. Rev. B: Condens. Matter Mater. Phys., 2003, 68, 054203 CrossRef.
- R. A. Martin, P. S. Salmon, H. E. Fischer and G. J. Cuello, J. Non-Cryst. Solids, 2004, 345–346, 208 CrossRef.
- B. E. Warren and J. Biscce, J. Am. Ceram. Soc., 1938, 21, 49 CrossRef CAS.
- R. A. Martin, P. S. Salmon, H. E. Fischer and G. J. Cuello, J. Phys.: Condens. Matter, 2003, 15, 8235 CrossRef CAS.
- R. A. Martin, P. S. Salmon, H. E. Fischer and G. J. Cuello, Phys. Rev. Lett., 2003, 90, 185501 CrossRef.
- A. N. Cormack and J. C. Du, J. Non-Cryst. Solids, 2001, 293, 283 CrossRef.
- A. Tilocca, J. Chem. Phys., 2008, 129, 084504 CrossRef.
- R. Hill, J. Mater. Sci. Lett., 1996, 15, 1122 CrossRef CAS.
- M. Edén, J. Non-Cryst. Solids, 2011, 357, 1595 CrossRef.
Footnote |
† Electronic supplementary information (ESI) available. See DOI: 10.1039/c3tb00408b |
|
This journal is © The Royal Society of Chemistry 2013 |
Click here to see how this site uses Cookies. View our privacy policy here.