A selective fluorescent chemosensor for phosphoserine†
Received 16th August 2013, Accepted 18th September 2013
First published on 19th September 2013
Abstract
A fluorescent chemosensor for the detection of phosphoserine is reported. The ditopic sensor features a phosphate-coordinating zinc(II)–dipicolylamine (Zn2+–DPA) unit tethered to an amine-binding coumarin aldehyde fluorophore. With phosphoserine, the sensor demonstrates a 30-fold fluorescence enhancement under buffered aqueous conditions.
Introduction
Phospholipids constitute the dominant class of lipids composing the lipid bilayer of cellular membranes and confer structural support, organization of membrane proteins, and intercellular signaling mechanisms.1–6 Phosphatidylcholine (PC), sphingomyelin (SM), phosphatidylethanolamine (PE), and phosphatidylserine (PS) represent over 97% of the total phospholipid composition and are asymmetrically distributed between the inner and outer leaflets of a cellular membrane.7 In quiescent cells, PC and SM constitute ∼87% of the total phospholipid content of the outer leaflet, with PS being exclusively restricted to and comprising ∼30% of the total phospholipid content of the inner leaflet (Fig. 1). Cell death randomizes the asymmetric distribution of phospholipids between the inner and outer leaflets, thereby externalizing PS to the outer cell surface.8–10 In fact, exposure of PS to the outer leaflet of the cellular membrane is the hallmark molecular biomarker for all types of cell death and serves as a signal for phagocytosis by providing ∼106–109 binding sites per cell.11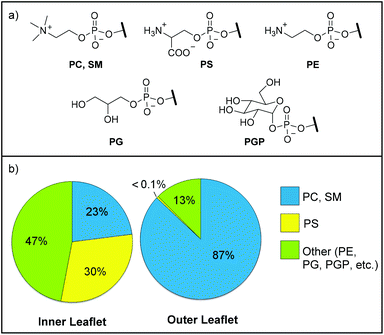 |
| Fig. 1 (a) Common cellular membrane phospholipid head groups. PC = phosphatidylcholine, SM = sphingomyelin, PS = phosphatidylserine, PE = phosphatidylethanolamine, PG = phosphatidylglycerol, PGP = phosphatidyl-glucopyranose. (b) Asymmetric phospholipid composition (mol%) of the outer and inner leaflets of healthy human cellular membranes.7 | |
Several methods to tag apoptotic cells and phospho-amino acid residues have appeared in the literature.12–17 In addition to biological probes (e.g., annexin assays), the vast majority of synthetic approaches have focused on detecting anionic phospholipid head groups, which includes phosphoserine. In particular, zinc(II)–dipicolylamine (Zn2+–DPA) complexes have proven to be admirable receptors for negatively-charged lipid head groups and have been used to tag apoptotic cells as well as bacterial cells.11,12,14,15,18,19 These primarily consist of two pre-coordinated Zn2+–DPA units tethered to a synthetic fluorophore that associate with the membrane surface of apoptotic cells through strong multipoint interactions with the anionic phospholipid head groups. These molecular probes are phospholipid tags and do not operate in a turn-on fashion. By contrast, a turn-on sensor for PS could give quantitative information about the amount of lipid present with low background as well as enable new types of experiments such as the ability to monitor the progression of cell death in a cell population.
Recently, we have been exploring the use of water-soluble shape-selective molecular tubes for hydrophobic sensing of lipids.20 In order to build selective sensors for PS, we require a head-group binding unit. We chose to use the highly-water soluble head groups as a model system for the analyses to demonstrate sensor efficacy because the head groups are the exposed units at the cell surface and the phospholipids lack water solubility. The head groups lack the hydrophobic lipid, yet remain functionally equivalent to the phospholipids and are reasonable analogues for evaluating head group binding units. In the case of phosphoserine, both the head group and PS possess a primary amine and an anionic phosphoester. Herein, we present a fluorescent turn-on molecular sensor based on the coumarin aldehyde scaffold for the selective recognition and sensing of phosphoserine (Fig. 2).
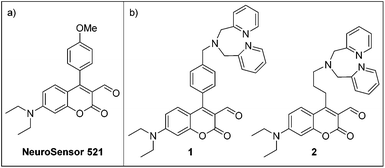 |
| Fig. 2 (a) Structure of NeuroSensor 521. (b) Ditopic sensors for phosphoserine. | |
The ditopic sensors 1 and 2 consist of an aldehyde recognition element that is integrated into the coumarin fluorophore and a single Zn2+–DPA recognition element appended to the coumarin scaffold to afford reversible binding to the primary amine and anionic phosphate moieties of phosphoserine, respectively (Scheme 1). The coumarin aldehyde has been shown to effectively produce a turn-on response to amine binding. Indeed, NeuroSensor 521 (NS521, Fig. 2a) was developed as a monotopic receptor for catecholamines and provides a turn-on response in live cells.21 In this study, we modified the NS521 scaffold by appending a Zn2+–DPA unit to the C4 position of the coumarin with both a rigid and flexible spacer to afford sensors 1 and 2, respectively. We hypothesized that a more rigid linker would provide stronger binding to the analyte but developed a flexible linker for comparison. Molecular models indicated that the methyl phenyl and propyl linkers would provide the optimal spacing for phosphoserine (see ESI†).
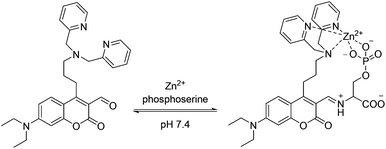 |
| Scheme 1 Ditopic binding of 2–Zn2+ to phosphoserine. | |
We reasoned that only phospholipid head groups with primary amines should bind to the coumarin aldehyde and form an iminium ion which causes an ∼40 nm bathochromic shift in absorption.21–23 By exciting the fluorophore at this new, more red wavelength, we can visualize a fluorescence increase due only to the bound sensor. The Zn2+–DPA group is likely the best receptor for phosphates in water and was incorporated to achieve selectivity.
Results and discussion
Synthesis of ditopic sensors
The syntheses of sensors 1 and 2 are shown in Scheme 2. To make sensor 1, compound 3 was coupled via its tosylate to an aryl boronic acid to produce compound 4. Chlorination followed by formylation using the Vilsmeier reagent gave compound 6 which was alkylated with a dipicolylamine unit to yield sensor 1. Sensor 2 was prepared from alcohol 723 by conversion to iodide 8 followed by alkylation with dipicolylamine.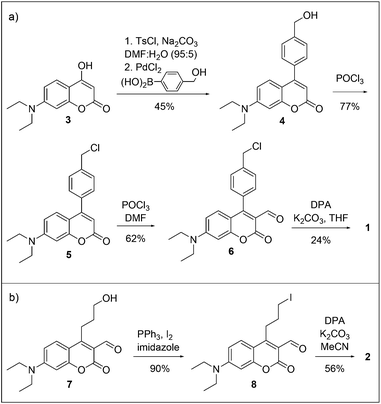 |
| Scheme 2 Syntheses of sensors (a) 1 and (b) 2. | |
UV and fluorescence titrations
Spectroscopic properties. When unbound, sensor 1 absorbs at 453 nm and emits at 517 nm. The quantum yield (Φ) was measured to be 0.0125 using fluorescein as a standard.24 Sensor 2 absorbs at 454 nm and emits at 513 nm with a quantum yield of 0.0213. Binding titrations. Sensors 1 and 2 were titrated with several phospholipid head groups: phosphoserine, phosphoethanolamine, phosphocholine, α-glycerolphosphate, and D-gluco-pyranose 1-phosphate, as well as the primary amines glutamate and glycine under buffered aqueous conditions (50 mM HEPES, 100 mM NaCl, pH 7.4). Glutamate and glycine served as controls for amine binding as they lack phosphate groups. The binding and spectroscopic data are summarized in Table 1. The absorbance of 1–Zn2+ shifted to 488 nm upon the addition of phosphoserine, phosphoethanolamine, glutamate, and glycine (see ESI†).
Table 1 Binding constants (Ka) and spectroscopic properties of sensors 1 and 2 with various analytesa
Analyte | 1 | 1–Zn2+ b | 2 | 2–Zn2+ b |
---|
Ka (M−1) | Isat/I0 c | Ka (M−1) | Isat/I0 c | Ka (M−1) | Isat/I0 c | Ka (M−1) | Isat/I0 c |
---|
Analytes of various concentrations were added to sensor (10 μM) in buffer (50 mM HEPES, 100 mM NaCl, pH 7.4). Sensor 1 was titrated in 97 : 3 buffer–DMSO and sensor 2 was titrated in 100% buffer. Zinc acetate (10 μM) was pre-coordinated with sensor prior to addition of analyte. Isat was taken from the theoretical maximum of the binding isotherm; λex = 488 nm; λem, 1 = 521 nm, λem, 2 = 513 nm. The phospholipid head groups phosphocholine, α-glycerolphosphate, and D-gluco-pyranose 1-phosphate did not bind or alter the fluorescence properties of either sensor. |
---|
Phosphoserine | 3.1 | 7.2 | 5.4 | 6.2 | 120 | 5.8 | 310 | 30 |
Phosphoethanolamine | 5.7 | 4.8 | 5.7 | 4.9 | 93 | 9.1 | 180 | 39 |
Glutamate | 4.7 | 7.0 | 7.0 | 4.3 | 22 | 13 | 190 | 17 |
Glycine | 6.1 | 6.1 | 6.2 | 4.0 | 5.3 | 16 | 6.6 | 29 |
Phosphocholine, α-glycerolphosphate, and D-gluco-pyranose 1-phosphate did not bind to the coumarin aldehyde of 1–Zn2+ and therefore did not alter the spectroscopic properties. All primary amine analytes bound to the coumarin aldehyde with low affinity and relatively low fluorescence enhancements (<7-fold) with emission maxima at 521 nm when excited at 488 nm. The binding results were similar to the control experiments conducted without Zn2+ indicating that the Zn2+–DPA had little effect on enhancing analyte binding to the coumarin aldehyde (see ESI†). Indeed, these binding constants are similar to those achieved with simple, unsubstituted coumarin aldehydes.21–23
Sensor 2 displayed similar absorption properties compared to sensor 1. The absorbance of 2–Zn2+ also shifted to the red only upon addition of phosphoserine, phosphoethanolamine, glutamate, and glycine. As seen with sensor 1, the phospholipid head groups that did not contain primary amines did not bind. The binding for phosphoserine was remarkably higher than for the other analytes tested with Ka = 310 M−1 (Fig. 3). Titrations with phosphoethanolamine and glutamate also demonstrated reasonable affinity, though not as high as with phosphoserine. The fluorescence increased over 30-fold with the addition of both phosphoserine and phosphoethanolamine, with emission at 513 nm when excited at 488 nm.
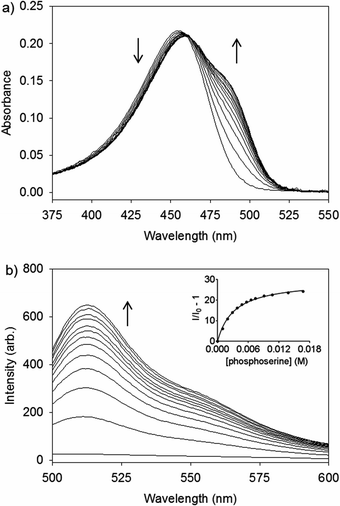 |
| Fig. 3 (a) UV/Vis and (b) fluorescence spectra of 2–Zn2+ (10 μM) with aliquots of 100 mM phosphoserine in buffer (50 mM HEPES, 100 mM NaCl, pH 7.4). λex = 488 nm. λem = 513 nm. Inset is the fit to a binding isotherm. | |
For sensor 2, the spectroscopic properties with Zn2+ were much more favorable than the control experiments without Zn2+, though the binding constants of 2 with phosphoserine, phosphoethanolamine, and glutamate were elevated compared to the typical low binding constants seen with monotopic coumarin aldehyde sensors.21–23 Presumably, the protonated form of the DPA can interact favorably with the charged phosphate to enhance binding. The fluorescence response of sensor 2 was lower without Zn2+ due to photoinduced electron transfer quenching from the uncoordinated DPA.
It is clear from the spectroscopic studies of both sensors that 2–Zn2+ is the best sensor for phosphoserine both in terms of binding constant and maximum fluorescence response (Fig. 4). Because of the flexible linker, 2–Zn2+ demonstrated more than two orders of magnitude better binding than the more rigid sensor 1. Clearly, the rigid structure for 1–Zn2+ prohibits a two-point binding interaction. Even sensor 2 lacking Zn2+ was a better receptor for phosphoserine than sensor 1.
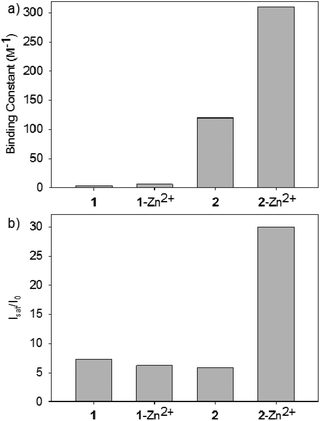 |
| Fig. 4 (a) Binding constants and (b) fluorescence enhancements (Isat/I0) of sensors 1 and 2 with phosphoserine. | |
Compared to the phospholipid tagging probes, 2–Zn2+ is unique in that it can coordinate to the analyte phosphate group and primary amine to achieve selective binding to phosphoserine over other phospholipid head groups. Thus, the head groups phosphocholine, α-glycerolphosphate, and D-gluco-pyranose-1-phosphate give no response, which is extremely important since the majority of membrane phospholipids are choline-based. In addition, 2–Zn2+ is also sensitive to the charge on the head group. Phosphoethanolamine and glutamate bind less effectively, as each bears one less negative charge than phosphoserine. It should be noted that while glutamate and glycine gave enhanced binding and fluorescence output, they are not expressed at high concentrations on a cellular membrane.
Conclusions
In conclusion, 2–Zn2+ demonstrates a means to selectively sense phosphoserine over other major phospholipid head groups by forming a two-point binding interaction with the phosphate and primary amine functional groups of the analyte under buffered aqueous conditions. Future work in this area will focus on joining this head group binding unit to our lipid receptor to produce sensitive and selective sensors for bioactive lipids.Experimental section
Spectroscopic analysis
Instrumentation. Absorption spectra were recorded on a Cary 1E spectrophotometer at 25 °C. Fluorescence spectra were recorded on a Shimadzu RF-5301 PC spectrofluorometer at 25 °C. NMR spectra were recorded on Bruker DRX 500.
Solution preparation. Solutions for UV/Vis and fluorescence spectroscopy were prepared from a 1 mg mL−1 sensor stock solution in MeOH that was diluted to volume with buffer (50 mM HEPES, 100 mM NaCl, pH 7.4) until a 10 μM sensor solution was produced following removal of the solvent. For sensor 1, DMSO was added to equal 3% of the total volume to improve solubility. Analytes were measured and diluted to volume with the buffered sensor solution to keep the sensor concentration constant throughout the titration.
Quantum yields. Separate stock solutions of sensors 1 and 2 (10 μM) in buffer (50 mM HEPES, 100 mM NaCl, pH 7.4) were prepared. The solution of sensor 1 contained 3% (v/v) DMSO as a cosolvent. For quantum yields (Φfl), a 1.0 μM solution of fluorescein (absolute Φfl = 0.85) in 0.1 N NaOH (pH 13) was used as a fluorescence standard. Sensors 1 and 2 were excited at 473 nm. The slit width was 2 nm for both excitation and emission. Quantum yields were obtained in triplicate and calculated as per the following equation: | 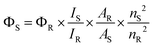 | (1) |
where ΦS = quantum yield of the sample, ΦR = quantum yield of the reference, IS = integrated fluorescence intensity of the sample, IR = integrated fluorescence intensity of the reference, AR = absorbance of the reference, AS = absorbance of the sample, nS = refractive index of the sample solvent, nR = refractive index of the reference solvent. Binding constant calculations. The concentration of analyte was plotted against the fluorescence intensity and fit to a one-site binding isotherm using the following equation: | 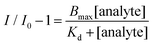 | (2) |
where I = fluorescence intensity, I0 = initial fluorescence intensity, Bmax = theoretical maximum fluorescence, and Kd = dissociation constant. Synthetic procedures
General methods. All reactions were carried out in dried glassware under a blanket of N2. Before use, tetrahydrofuran and diethyl ether were distilled from sodium benzophenone ketyl while dichloromethane and triethylamine were distilled from CaH2. NMR spectra were recorded on Bruker DRX 500. IR spectra were recorded on a Nexus 670 FT-IR E.S.P. spectrometer.
Materials. All chemicals were obtained from Sigma Aldrich, Fisher Scientific, Combi-Blocks, TCI America, or Alfa Aesar and were used without further purification. Flash chromatography was performed with 32–63 μm silica gel.
Compound 4. Compound 3 (250 mg, 1.072 mmol), Na2CO3 (340 mg, 3.215 mmol), and TsCl (204.4 mg, 1.072 mmol) were dissolved in 10 mL degassed DMF–H2O (95
:
5). The solution stirred at 60 °C for 1 h. Then 4-(hydroxymethyl)phenylboronic acid (179.2 mg, 1.179 mmol) and PdCl2 (29 mg, 0.161 mmol) were added and N2 was bubbled through for 5 min. The solution stirred at 60 °C for 16 h. The inorganic solids were filtered off and rinsed with acetone. The solvent was removed in vacuo and the remaining crude material was purified by chromatography (95
:
5 CH2Cl2–EtOAc) to yield compound 4 (156.5 mg, 45%) as a yellow solid (mp 62 °C): 1H NMR (500 MHz, CDCl3) δ 7.50 (d, 2H, J = 7.5 Hz), 7.43 (d, 2H, J = 7.5 Hz), 7.23 (d, 1H, J = 9.0 Hz), 6.57 (d, 1H, J = 2.0 Hz), 6.51 (dd, 1H, J = 9.0, 2.0 Hz), 5.99 (s, 2H), 4.80 (s, 2H), 3.42 (q, 4H, J = 7.0), 1.21 (t, 6H, 7.0 Hz); 13C NMR (125 MHz, CDCl3) δ 162.1, 156.8, 155.8, 150.6, 142.1, 135.5, 128.6, 127.8, 127.1, 108.5, 108.2, 107.9, 97.9, 64.8, 44.8, 12.4; IR (neat, cm−1) 3412, 2970, 1695, 1605, 1589, 1417, 1352, 1115; HRMS calculated for C20H21NO3Na (M + Na+): 346.1414. Found: 346.1414. Compound 5. Compound 4 (100 mg, 0.309 mmol) and POCl3 (3 mL) were combined in a round bottom flask and stirred at room temperature for 21 h. The mixture was poured over ice water (10 mL) and extracted with CH2Cl2 (10 mL × 4). The organic solvent was removed in vacuo and the resulting material was purified by chromatography (90
:
10 CH2Cl2–EtOAc) to yield compound 5 (81 mg, 77%) as a yellow oil: 1H NMR (500 MHz, CDCl3) δ 7.51 (d, 2H, J = 8.0 Hz), 7.43 (d, 2H, J = 8.0 Hz), 7.22 (d, 1H, J = 9.0 Hz), 6.56 (d, 1H, J = 2.5 Hz), 6.51 (dd, 1H, J = 9.0, 2.5 Hz), 5.98 (s, 1H), 3.41 (q, 4H, J = 7.0 Hz), 1.20 (t, 6H, J = 7.0 Hz); 13C NMR (125 MHz, CDCl3) δ 161.9, 156.7, 155.4, 150.6, 138.5, 136.3, 128.8, 128.7, 127.7, 108.5, 108.3, 107.6, 97.8, 45.6, 44.7, 12.4; IR (neat, cm−1) 2970, 1708, 1614, 1593, 1524, 1417, 1356, 1115; HRMS calculated for C20H20ClNO2Na (M + Na+): 364.1075. Found: 364.1077. Compound 6. The Vilsmeier reagent was made by combining 2 mL POCl3 and 3.6 mL DMF in a flame-dried round bottom flask at 0 °C and stirring for 30 min. In a separate flask, 142.7 mg of compound 5 was dissolved in 3 mL DMF. The starting material was cooled to 0 °C and 3 mL of the Vilsmeier reagent was added dropwise. The reaction was brought to room temperature and stirred for 40 h. The mixture was poured over ice water (30 mL), extracted with CH2Cl2 (20 mL × 6), the organic layers combined and dried over Na2SO4, and the solvent removed in vacuo. Purification via column chromatography (95
:
5 CH2Cl2–EtOAc) yielded compound 6 as a yellow oil (97 mg, 62%): 1H NMR (500 MHz, CDCl3) δ 9.89 (s, 1H), 7.54 (d, 2H, J = 8.0 Hz), 7.26 (d, 2H, J = 8.0 Hz), 6.94 (d, 1H, J = 9.5 Hz), 6.49–6.53 (m, 2H), 4.68 (s, 2H), 3.45 (q, 4H, J = 7.0 Hz), 1.23 (t, 6H, J = 7.0 Hz); 13C NMR (125 MHz, CDCl3) δ 188.2, 160.8, 160.1, 157.7, 153.1, 138.3, 133.4, 131.0, 128.6, 128.5, 112.0, 109.8, 108.9, 97.0, 45.7, 45.2, 12.4; IR (neat, cm−1) 1614, 1559, 1494, 1417, 1352; HRMS calculated for C21H20ClNO3Na (M + Na+): 392.1024. Found: 392.1022. Sensor 1. Compound 6 (102 mg, 0.276 mmol), di(2-picolyl)amine (55 μL, 0.290 mmol), K2CO3 (114 mg, 0.828 mmol), and 7 mL THF were combined in a round bottom flask at stirred at 55 °C for 21 h. The mixture was filtered and rinsed with acetone. The filtrate solvent was removed in vacuo and the remaining residue was purified via column chromatography (100% CH2Cl2 → 100% EtOAc → 80
:
20 EtOAc–MeOH) to yield sensor 1 as a yellow oil (54 mg, 47%). 1H NMR (500 MHz, CDCl3) δ 9.76 (s, 1H) 8.55 (d, 2H, J = 5.0 Hz), 7.69 (td, 2H, J = 7.5, 1.5 Hz), 7.61 (d, 2H, J = 8.0 Hz), 7.54 (d, 2H, J = 8.0 Hz), 7.21 (d, 2H, J = 8.0 Hz), 7.17 (m, 2H), 6.93 (d, 1H, J = 9.5 Hz), 6.45–6.51 (m, 2H), 3.88 (s, 4H), 3.80 (s, 2H), 3.43 (q, 4H, J = 7.0 Hz), 1.21 (t, 6H, J = 7.0 Hz); 13C NMR (125 MHz, CDCl3) δ 188.3, 162.2, 159.5, 159.4, 157.7, 153.0, 148.9, 140.2, 136.5, 131.5, 130.9, 128.7, 128.5, 122.8, 122.0, 112.2, 109.6, 108.9, 96.9, 60.1, 58.1, 45.1, 12.4; IR (neat, cm−1) 1746, 1611, 1495, 1422, 1356, 1133, 731; HRMS calculated for C33H32N4O3Na (M + Na+): 555.2367. Found: 555.2366. Compound 8. Compound 7 (0.0412 g, 0.1358 mmol), iodine (0.0655 g, 0.2580 mmol), triphenylphosphine (0.0641 g, 0.2444 mmol) and imidazole (0.0185 g, 0.2716 mmol) were dissolved in dichloromethane (7.0 mL) and stirred at room temperature for 2 h. The mixture was filtered and columned twice on silica with 9
:
1 CH2Cl2–EtOAc to give compound 8 (48.9 mg, 90%) as a yellow solid (decomp 138–141 °C). 1H NMR (500 MHz, CDCl3) δ 10.35 (s, 1H), 7.73 (d, 1H, J = 16.0 Hz), 6.69 (dd, 1H, J = 15.5, 4.5 Hz), 6.47 (d, 1H, J = 4.5 Hz), 3.33–3.52 (m, 8H), 2.05–2.15 (m, 2H), 1.26 (t, 6H, J = 7.0 Hz); 13C NMR (125 MHz, CDCl3) δ 190.9, 162.9, 161.5, 157.6, 152.8, 128.6, 111.8, 110.1, 108.4, 97.3, 45.1, 33.5, 28.9, 12.5, 6.8; IR (neat, cm−1) 2913, 1716, 1671, 1614, 1556, 1503, 1454, 1352; HRMS calculated for C17H20INO3Na (M + Na+): 436.0380. Found: 436.0372. Sensor 2. In a flame-dried flask, compound 8 (0.0485 g, 0.1174 mmol), potassium carbonate (0.0243 g, 0.1761 mmol), and di(2-picolyl)amine (31.7 μL, 0.1761 mmol) were dissolved in acetonitrile (4.1 mL) and the flask was flushed with N2. The mixture was stirred at room temperature for 60 h. Column chromatography on silica with 1
:
1 CH2Cl2–EtOAc gave sensor 2 (24.1 mg, 56%) as a yellow solid (mp 109 °C). 1H NMR (500 MHz, CDCl3) δ 10.34 (s, 1H), 8.55 (d, 2H, J = 7.0 Hz), 7.56–7.71 (m, 5H), 7.14–7.19 (m, 2H), 6.52 (dd, 1H, J = 9.5, 4.5 Hz), 6.44 (d, 1H, J = 4.5 Hz); 13C NMR (125 MHz, CDCl3) δ 190.7, 163.8, 163.1, 159.6, 157.5, 152.6, 149.0, 136.4, 128.7, 123.0, 121.9, 111.7, 109.8, 108.5, 97.2, 60.2, 54.3, 45.0, 27.8, 25.8, 12.4; IR (neat, cm−1) 2970, 2921, 1712, 1676, 1615, 1559, 1508, 1452, 1355, 1148, 1131; HRMS calculated for C29H32N4O3Na (M + Na+): 507.2367. Found: 507.2357. Acknowledgements
This work was supported by the National Science Foundation (CHE-1112194).Notes and references
- M. I. Gurr, J. L. Harwood and K. N. Frayn, Lipid Biochemistry: An Introduction, Oxford, UK, 5th edn, 2002, pp. 215–259 Search PubMed.
- R. O. Ryan and D. J. van der Horst, Annu. Rev. Entomol., 2000, 45, 233 CrossRef CAS PubMed.
- M. Dykstra, A. Cherukuri, H. W. Sohn, S.-J. Tzeng and S. K. Pierce, Annu. Rev. Immunol., 2003, 21, 457 CrossRef CAS PubMed.
- M. Kielian, P. K. Chatterjee, D. L. Gibbons and Y. E. Lu, Subcell. Biochem., 2000, 34, 409 CrossRef CAS.
- K. Lauber, E. Bohn, S. M. Kröber, Y.-J. Xiao, S. G. Blumenthal, R. K. Lindemann, P. Marini, C. Wiedig, A. Zobywalski, S. Baksh, Y. Xu, I. B. Autenrieth, K. Schulze-Osthoff, C. Belka, G. Stuhler and S. Wesselborg, Cell, 2003, 113, 717 CrossRef CAS.
- S. A. Islam, S. Y. Thomas, C. Hess, B. D. Medoff, T. K. Means, C. Brander, C. M. Lilly, A. M. Tager and A. D. Luster, Blood, 2006, 107, 444 CrossRef CAS PubMed.
- J. A. Virtanen, K. H. Cheng and P. Somerharju, Proc. Natl. Acad. Sci. U. S. A., 1998, 95, 4964 CrossRef CAS.
- Y. Y. Tyurina, A. A. Shvedova, K. Kawai, V. A. Tyurin, C. Kommineni, P. J. Quinn, N. F. Schor, J. P. Fabisiak and V. E. Kagan, Toxicology, 2000, 148, 93 CrossRef CAS.
- G. Preta and B. Fadeel, PLoS One, 2012, 7, 1 Search PubMed.
- T. T. Tung, K. Nagaosa, Y. Fujita, A. Kita, H. Mori, R. Okada, S. Nonaka and Y. Nakanishi, J. Biochem., 2013, 153, 483 CrossRef CAS PubMed.
- B. A. Smith, S. T. Gammon, S. Xiao, W. Wang, S. Chapman, R. McDermott, M. A. Suckow, J. R. Johnson, D. Piwnica-Worms, G. W. Gokel, B. D. Smith and W. M. Leevy, Mol. Pharmaceutics, 2011, 8, 583 CrossRef CAS PubMed.
- B. A. Smith and B. D. Smith, Bioconjugate Chem., 2012, 23, 1989 CrossRef CAS PubMed.
- B. A. Smith, S. Xiao, W. Wolter, J. Wheeler, M. A. Suckow and B. D. Smith, Apoptosis, 2011, 16, 722 CrossRef PubMed.
- R. G. Hanshaw and B. D. Smith, Bioorg. Med. Chem., 2005, 13, 5035 CrossRef CAS PubMed.
- S. W. Bae, M. S. Cho, A. R. Jeong, B.-R. Choi, D.-E. Kim, W.-S. Yeo and J.-I. Hong, Small, 2010, 6, 1499 CrossRef CAS PubMed.
- P. Atkinson, B. S. Murray and D. Parker, Org. Biomol. Chem., 2006, 4, 3166 CAS.
- T. Anai, E. Nakata, Y. Koshi, A. Ojida and I. Hamachi, J. Am. Chem. Soc., 2007, 129, 6232 CrossRef CAS PubMed.
- A. G. White, B. D. Gray, K. Y. Pak and B. D. Smith, Bioorg. Med. Chem. Lett., 2012, 22, 2833 CrossRef CAS PubMed.
- C. Lakshmi, R. G. Hanshaw and B. D. Smith, Tetrahedron, 2004, 60, 11307 CrossRef CAS PubMed.
- C. T. Avetta, B. J. Shorthill, C. Ren and T. E. Glass, J. Org. Chem., 2012, 77, 851 CrossRef CAS PubMed.
- K. S. Hettie, X. Liu, K. D. Gillis and T. E. Glass, ACS Chem. Neurosci., 2013, 4, 918 CrossRef CAS PubMed.
- K. E. Secor and T. E. Glass, Org. Lett., 2004, 6, 3727 CrossRef CAS PubMed.
- E. K. Feuster and T. E. Glass, J. Am. Chem. Soc., 2003, 125, 16174 CrossRef CAS PubMed.
- C. A. Parker and W. T. Rees, Analyst, 1960, 85, 587 RSC.
Footnote |
† Electronic supplementary information (ESI) available: UV/Vis and fluorescence spectroscopic results and 1H and 13C NMR spectra. See DOI: 10.1039/c3ob41677a |
|
This journal is © The Royal Society of Chemistry 2013 |