DOI:
10.1039/C3FO60291E
(Paper)
Food Funct., 2013,
4, 1668-1674
Investigation of milk proteins binding to the oral mucosa†
Received 23rd July 2013, Accepted 21st September 2013
First published on 23rd September 2013
Abstract
High protein dairy beverages are considered to be mouth drying. The drying sensation may be due to the product protein content; however the mechanism of this mouth drying is uncertain. This study investigated the potential adhesion of milk proteins to porcine oral mucosa in vitro. Purified casein and β-lactoglobulin were fluorescently labelled, placed on porcine oral mucosal tissues and their resistance to wash out with simulated saliva was monitored using fluorescence microscopy. Casein was found to be more adhesive to porcine mucosa than β-lactoglobulin. Some investigation into the reason for this difference in mucoadhesion was conducted by thiol-content analysis, rheology and zeta-potential measurements. The higher viscosity of casein solution and smaller zeta-potential is believed to be responsible for its better retention on mucosal surfaces. These findings suggest that casein and whey protein are both capable of binding and eliciting mouth drying in high protein dairy beverages.
Introduction
Astringency is a drying sensation elicited by some foods and beverages. In red wine, black tea, and cranberry juice, this sensation is thought to originate from the polyphenols present.1 Some reports also suggest that astringency may be related to the aggregation of salivary proteins caused by their interactions with food and beverage components.2 A drying sensation has been found to occur in some dairy based products, such as high protein shakes for athletes, acidic whey based beverages and nutritional supplements for those at risk of malnutrition.3This mouth drying sensation has also been found to build up with repeated consumption of protein rich dairy beverages.4
The reasons for mouth drying in dairy beverages are unclear. For reconstituted drinks, the rehydration of milk powders is poor,5 which may be the cause of some astringency. Additionally, the interaction of acidic whey beverages directly with saliva,6 or the precipitation of proteins from these whey beverages within the oral cavity at the isoelectric point7,8 may have an effect. However, many dairy beverages are pH 6.5–7 and therefore proteins are unlikely to precipitate within the oral cavity, thus suggesting a different mechanism must occur in such high protein products. Another potential mechanism may be the direct interaction between dairy proteins and the oral mucosa, i.e. their mucosal adhesion.
The human oral mucosa, is a protective barrier of cells, around 500–800 μm thick. It consists of two clear layers, the epithelium facing into the oral cavity, and the basement membrane below with associated connective tissues.9 The oral mucosa cells mature and migrate from the basal layer towards the epithelial surface, increasing in size and flattening out to prevent penetration of the epithelium by foreign substances.9,10 The basement membrane anchors the epithelium to the connective tissue below preventing foreign large molecules from penetrating the oral mucosa.10 Mucus gel, which mostly consists of negatively charged glycoproteins (mucins), covers the entire oral cavity.
Polymers containing ionic groups often exhibit a strong ability to adhere to these mucosal surfaces. Typical mucoadhesive polymers are either anionic, e.g. poly(acrylic acid), or cationic such as chitosan. Excellent mucoadhesive properties of poly(acrylic acid) are believed to be due to the formation of hydrogen bonds with mucins.11 Chitosan and other cationic polymers have a strong tendency to interact with negatively charged mucins via electrostatic attraction and additional secondary interactions (hydrogen bonding and hydrophobic effects).12,13
Relatively little is known about the mucoadhesive ability of amphoteric polymers such as proteins. It is believed that amphoteric polymers have poor ability to adhere to mucosal surfaces due to the self-neutralisation between cationic and anionic groups present within their macromolecules.14
This study aimed to evaluate the retention of milk proteins (casein and β-lactoglobulin) on the oral epithelial surfaces including buccal and tongue tissues. The hypothesis of this study was that the adhesion of milk proteins to the oral epithelium may be related to mouth drying in dairy products.
Materials and methods
Fluorescent labelling of milk proteins
Two major pure milk proteins, casein and β-lactoglobulin, were labelled with fluorescein isothiocyanate (FITC) to enable fluorescence. The proteins were purchased from Sigma Aldrich, UK at 99% purity for casein, comprising α-s1 (44%), α-s2 (12%), β (32%) and κ (12%) caseins whilst β-lactoglobulin protein was >90% pure. Protein powders were dissolved in a 0.1 M sodium carbonate buffer to make a 2 mg mL−1 solution at pH 9.0. FITC solution (1 mg mL−1, in DMSO) was added to protein solutions at a ratio of 50 μL per mL. The resulting FITC/protein solutions were incubated in a light proof container at 4 °C, for 8 h, before addition of ammonium chloride to a concentration of 50 mM. Solutions were further incubated for 2 h with stirring in the dark. Xylene cyanol and glycerol were added to 0.1% and 5%, respectively, before the unbound FITC was separated from the conjugate protein by fine-sized gel filtration using a column packed with Sephadex G50 (Sigma-Aldrich, UK). A 20–50 kDa exclusion limit was selected on the gel matrix in order that the conjugate proteins eluted prior to the unbound FITC. The conjugate proteins were then freeze dried for storage and rehydrated to 8.7% (representative of protein content of beverages) in distilled water at 20 °C and vortexed before use. To compare the occurrence of milk protein mucoadhesion to a negative control, 18 kDa FITC-dextran (Sigma Aldrich, UK) was dissolved at 1 mg mL−1, vortex mixed, and used in this study. Thin layer chromatography of the samples confirmed the absence of unbound FITC in the product (Fig. 1s, ESI†). All chemicals required for fluorescein protein labelling, and Ellman's assay reagents were purchased from Sigma Aldrich, UK.Spectrofluorometry of free and FITC-conjugated proteins
To measure the fluorescence of labelled and unlabelled proteins, 1 mg mL−1 solutions of lyophilised FITC-proteins were made up by direct dissolution in water. In addition, 1 mg mL−1 solutions of the unlabelled β-lactoglobulin and casein were produced by dissolution into PBS. The fluorescence of these solutions was then measured by spectrofluorometry (Jasco FP-6200, λexc: 488 nm, λemi: 505–600 nm). This aided confirmation of conjugation and resultant fluorescence.Sample preparation – tissue dissection and protein binding
Porcine cheek and tongue tissues were obtained from PC Turners, Abattoir, UK; Jennings of Caversham, UK; Gilberts Meat Market, UK and dissected into 1 cm2 sections of epithelial samples. Two porcine tongues and two buccal tissues were dissected in total, producing ten samples each from the three areas of each tongue for analysis, the apex, edges and posterior (Fig. 2s, ESI†).To assess the retention on the mucosa, protein solutions were applied to the oral epithelium and washed repeatedly with artificial saliva (A/S Pharma Orthana Kemisk, Denmark). The proportion of protein solution and saliva were assessed based on a 5 mL sip of high protein dairy-based beverages, 0.44 g protein per 5 mL was placed in contact with the oral mucosa and washed with 1 mL of saliva. This volume of saliva was selected as an estimation of stimulated saliva production per minute, although there can be a wide range of variation between individuals.15 To further simulate the conditions within the mouth, 20 μL of rehydrated protein solutions were pipetted directly onto the tissues, as the surface area of the mucosal tissue was considerably smaller than the mouth and observed directly under fluorescence microscopy. The tissues were then washed up to fifty times with 38.5 μL aliquots of artificial saliva and observed under fluorescence microscopy after 1, 5, 10, 20, 30 and 50 washes. This aimed to simulate the consumption of a sip of high-protein dairy beverage, and the effect of stimulated saliva production for the subsequent 50 minutes post ingestion.
Fluorescence microscopy
Fluorescence microscopy of buccal and tongue epithelial tissue samples was conducted on an Axioskop 2 (Zeiss, USA) fluorescence microscope. The specimens were observed at ×5 magnification, under blue laser light, which covered the excitation wavelength of fluorescein (λex: 488 nm) and the emitted green light was observed. The same mucosal tissue pieces were supplemented with protein and washed repeatedly, using a different tissue piece for each protein type; therefore there were twelve mucosal pieces in total, one for each area, and each protein type. At each washing step ten digital micrographs were taken of the surface of each tissue piece, producing 60 photos of each piece of epithelial tissue over the entire washing process (Axiocam, Zeiss, USA) and the fluorescence of each image was measured by ImageJ photo analysis software (version 1.46r, National Institutes of Health, USA). The fluorescence of each photo was normalised by mapping the original fluorescence data onto a 100 point scale, which assigned a scaled number to the fluorescence of each photo. The total fluorescence at each washing stage was averaged from the normalised data of the casein, β-lactoglobulin and dextran photos.Rheological analysis
To assess the potential differences in the mucoadhesive properties of casein and β-lactoglobulin, the viscosities of each protein solution were assessed at the same concentration as in mucoadhesion analysis (8.7% (w/w) protein in water). The viscosity was assessed with an oscillatory rheometer (AR2000, TA Instruments, USA), using a 40 mm head at a shear rate of 50 s−1, similar to the forces exerted within the mouth.16Zeta-potential measurements
Zeta-potential measurements were performed with solutions of proteins at 25 °C using Nano-S Zetasizer (Malvern Instruments, UK).Determination of the ‘force of bioadhesion’
To assess the potential differences in the mucoadhesive properties of casein and β-lactoglobulin, the viscosities of each protein solution were assessed and related to a ‘force of bioadhesion’ by a modification of the method of Hassan and Gallo.17 2% (w/v) solutions of casein and β-lactoglobulin were prepared in PBS. These were then mixed in equal volume with either PBS or artificial saliva to produce 1% (w/v) with, or without, mucin. A reference sample of artificial saliva, diluted twofold in PBS was also prepared. The viscosity of the solutions was assessed with an oscillatory rheometer (AR2000, TA Instruments, USA), using a 40 mm head at a shear rate of 50 s−1, similar to the forces exerted within the mouth.16 A force of bioadhesion (F) was assigned using the equation:
F = (ηm − ηs − ην) |
where ηm, ηs and ηp are the viscosities of the saliva/protein mixture, the saliva alone and the protein, respectively, in Pa·s.
is the shear rate, in s−1.Thiol content analysis
Ellman's assay was used to assess and compare the thiol contents in the proteins. This method was adapted from Bravo-Osuna et al. and Irmukhametova et al.18,19 Briefly, 1 mg mL−1 protein solutions were prepared in 500 μL phosphate buffer (0.5 M, pH 8) and allowed to dissolve for 60 minutes. 3 mg of the Ellman's reagent or DTNB (5,5′-dithiobis-(2-nitrobenzoic acid)) was dissolved in 10 mL of the same 0.5 M, pH 8.0 phosphate buffer, before 500 μL of this DTNB solution was added to 500 μL of the proteins and incubated in the dark for 2 h. The treated protein solution absorbance was then measured at 420 nm (Spectrophotometer UV/VIS Lambda 11 Perkin-Elmer, Norwalk, USA). The thiol content was subsequently calculated from the corresponding standard curve established using a range of cysteine hydrochloride concentrations between 18 and 500 μM.Results
Mucoadhesion of proteins to porcine oral mucosa
Fluorescence microscopy has previously been reported as a powerful technique to characterise the mucoadhesive properties of fluorescently labelled nanoparticles.19 The application of this technique has helped to compare the retention potential of different nanoparticles on ocular surfaces in a biological fluid flow. In this study we have utilised a similar approach through the use of fluorescently labelled casein and β-lactoglobulin. FITC-dextran was used as a negative control in these experiments because it is not expected to provide substantial retention effects as a non-ionic poorly mucoadhesive polymer.14Spectrofluorometry showed the emission of light in the region of 500–580 nm for both FITC-labelled β-lactoglobulin and casein during excitation at 488 nm. This range of emitted light corresponds to the green region of the visible light spectrum allowing the observation of labelled proteins on the surface of tissues, when excited with blue light (λ ≈ 450–490 nm).
The emission of light by the FITC-β-lactoglobulin samples was approximately 2.7 lower than the corresponding FITC-casein emission indicating a lower degree of conjugation, or increased fluorescence quenching by the protein in this case (Fig. 1).
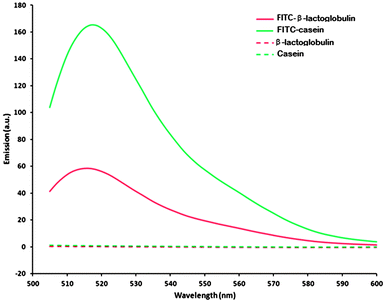 |
| Fig. 1 Fluorescent spectra of FITC-labelled and control proteins. | |
Unlabelled samples showed no fluorescence at this wavelength, indicating that no intrinsic fluorescence would be demonstrated by the proteins alone. Therefore, all fluorescence observed during microscopy originated from the FITC-bound proteins, with casein exhibiting greater fluorescence than β-lactoglobulin in this study. Dynamic light scattering indicated that the labelling process did not destroy the micellar structure of casein in solution (Fig. 3s, ESI†).
Buccal mucosa (cheek epithelium)
Micrographs were taken for each mucosal tissue before placing any solutions of fluorescently labelled materials to establish the background levels of fluorescence. The fluorescently labelled casein, β-lactoglobulin and FITC-dextran solutions were then placed on the porcine buccal mucosa, and washed repeatedly with artificial saliva. Fluorescent images were taken prior to washing and subsequently after each washing stage (Fig. 2). Areas rich in green colour indicate the presence of substantial amount of fluorescently labelled material on mucosal surface. Additionally, the distribution of protein on the surface of the tissue appears to be very heterogeneous, thus 5 replicate images were used to minimise the effect of this heterogeneity. The images were subsequently processed using ImageJ software to quantify the presence of fluorescent materials (Fig. 3).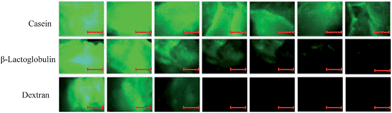 |
| Fig. 2 Exemplary fluorescent microphotographs, showing the retention of casein, β-lactoglobulin and dextran on buccal mucosa against the number of washes with artificial saliva. Scale bars indicate 500 μm at image magnification. Fluorescent microphotographs for other experiments are not shown. | |
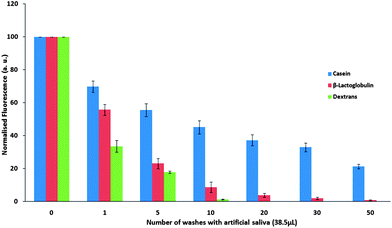 |
| Fig. 3 Normalised fluorescence intensity ± SD, of FITC-bound casein, β-lactoglobulin and dextran on porcine buccal mucosa over repeated washing with artificial saliva. | |
As expected, FITC-dextran exhibited relatively little interaction with the buccal mucosa, with poor resistance to saliva wash out, resulting in its complete disappearance from the buccal surface after 10–20 washes.
The presence of β-lactoglobulin on the mucosal surface was detected even after 30 washes, which confirms its stronger ability to adhere to buccal membrane compared to FITC-dextran. This suggests that although β-lactoglobulin interacts with the cheek mucosal surface, the salivary washes were able to eventually remove this protein. Casein was found to retain on the mucosal surface in substantial quantities even after 50 washes indicating that this protein also strongly interacts with mucosal surface. The buccal mucosa, unlike tongue mucosa, is non-specialised with no keratinisation and without papillae.20 This could influence and possibly enhance the binding of polymer to the mucosa compared to other regions of the oral cavity, and may therefore give clear indications of protein's mucoadhesive properties.
Tongue apex
The apex of a porcine tongue, similarly to a human tongue, is papillae dense.21 Therefore, this difference to the buccal mucosa may influence the interaction of the milk proteins with its epithelium. The wash out profiles recorded for fluorescently labelled casein, β-lactoglobulin and dextran from the porcine tongue apex tissue are shown in Fig. 4.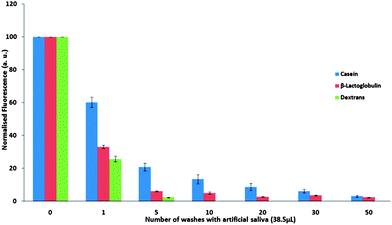 |
| Fig. 4 Normalised fluorescence intensity ± SD of FITC-bound casein, β-lactoglobulin and dextran on porcine tongue apex over repeated washing with artificial saliva. | |
The retention of casein and β-lactoglobulin is also fairly strong on the porcine tongue apex epithelium. This is confirmed by the presence of protein traces on the tissue even after fifty washes. However, a quicker decline in the intensity of fluorescence with the number of washes indicates that the binding to this tissue is not as strong as to the buccal mucosa for either protein. Casein shows slightly better retention compared to β-lactoglobulin.
Posterior tongue
The posterior area of the porcine tongue is rich in follate papillae, and therefore it may be considered as another potential surface for protein retention (Fig. 5). Both proteins showed relatively poor adhesion to the tongue posterior and were removed fully over twenty salivary washes. This is similar to the tongue edge findings suggesting that these milk proteins do not bind effectively to these regions of the tongue.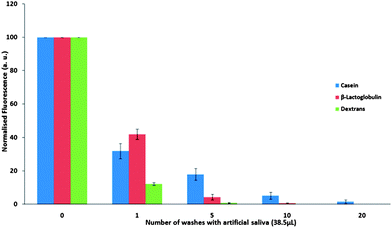 |
| Fig. 5 Normalised fluorescence intensity ± SD of FITC-bound casein, β-lactoglobulin and dextran on porcine tongue posterior over repeated washing with artificial saliva. | |
Tongue edge
The edges of the porcine tongue body also have papillae, but are less densely papillated than the apex, therefore should be assessed for mucoadhesive binding capabilities for milk proteins (Fig. 6).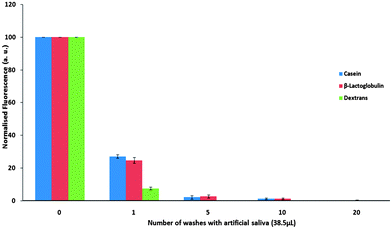 |
| Fig. 6 Normalised fluorescence intensity ± SD of FITC-bound casein, β-lactoglobulin and dextran on porcine tongue edge over repeated washing with artificial saliva. | |
On the porcine tongue edge the retention of both casein and β-lactoglobulin was found to be considerably less efficient compared to the other mucosal tissues of the oral epithelium. The presence of the proteins on the mucosal surface is detectable for around twenty washes compared to the fifty washes required to remove casein and β-lactoglobulin from the buccal mucosa and the tongue apex. The proteins also exhibit a better retention ability compared to dextran. This suggests some interaction of the proteins with the mucosal surface; however, the binding is relatively weak.
The differences observed in the binding of proteins to regions of oral mucosa may relate to a range of physiological factors. On the tongue surface, variation in papillae density and type on the areas investigated may influence mucoadhesion. Filiform and fungiform papillae cover the body and apex of the tongue, with vallate papillae towards the posterior tongue and the root.21,22 The quantity of papillae is greater at the apex than the dorsal surface or the posterior. The high density of fungiform papillae may also be responsible for additional binding to the tongue tip, which may explain the higher binding to the tongue apex especially over other regions such as the posterior.21
Thiol content, zeta-potential and rheology
The ability of pharmaceutical polymers to adhere and retain on mucosal surfaces is known to be related to a number of factors, including their chemical structure, molecular weights and rheological properties.14 Polymers bearing thiol functional groups (polymeric thiomers) have been reported to exhibit superior mucoadhesive ability compared to thiol-free materials.23 These excellent mucoadhesive properties of polymeric thiomers are related to their ability to form covalent –S–S– linkages with cysteine-rich domains in mucins. In order to get an insight into the nature of milk proteins mucoadhesive potential we have determined the thiol content in casein and β-lactoglobulin and evaluated their zeta-potential and rheological properties in solutions (Table 1).
Table 1 Thiol content, zeta-potential, viscosity and force of bioadhesion of casein and β-lactoglobulin solutions
Protein | Thiol content (μM mg−1) | Viscosity (mPa·s) | Zeta-potential (mV) | Force of bioadhesion (Pa) |
---|
Casein | 66.2 ± 8.1 | 1.4 ± 0.2 | −10.6 ± 2.1 | −0.020 ± 0.002 |
β-lactoglobulin | 197.3 ± 14.4 | 0.6 ± 0.1 | −22.1 ± 0.9 | 0.152 ± 0.032 |
The thiol content in casein and β-lactoglobulin was determined by Ellman's assay. The level of thiols in β-lactoglobulin was found to be significantly higher than in casein (p < 0.001), which is in good agreement with the previous reports.24–26 Both proteins were found to be negatively charged, with β-lactoglobulin having a higher potential at the shear plane (ξ = −22.1 ± 0.9 mV) compared to casein (ξ = −10.6 ± 2.1 mV). The greater zeta-potential of β-lactoglobulin implies a greater stability of the protein in solution, relative to casein. A comparison of rheological properties was conducted using the 8.7% protein solutions at 50 s−1, the shear forces present in the mouth.16
The viscosity of casein solution was 2.3 times higher than β-lactoglobulin, which is likely related to the ability of casein to form naturally self-assembled micellar structures.27 Measurement of the so-called ‘force of bioadhesion’ gave a value of 0.152 ± 0.032 Pa for β-lactoglobulin, but −0.020 ± 0.002 Pa for casein. This data supports the presence of an interaction between β-lactoglobulin and mucin present in the saliva, but not for casein. This may be a result of the markedly different rheological properties of casein, in part a result of its micellar nature, which has been reported to greatly influence measurement of the force of bioadhesion.28
The different extent of casein and β-lactoglobulin mucoadhesion to the oral epithelium suggests that these proteins differ in strength of interaction with the mucosa. Mucoadhesion to the oral epithelium may depend on the physicochemical properties and structural features of the proteins (presence of functional groups, charge, etc).29 The thiol content in β-lactoglobulin is higher compared to casein, which should possibly make the latter protein less mucoadhesive. However, in our case better mucoadhesiveness for casein was observed, which indicates that there are other factors determining the extent of mucoadhesion. The overall negative charge of both proteins in the near-neutral pH of the oral cavity30–32 suggests that electrostatic interactions are unlikely to be the main cause of mucoadhesion. However, the less charged casein should feel a weaker repulsion from the negatively charged mucosal surface compared to β-lactoglobulin. Additionally, zeta-potential measurements suggest that the casein is less stable to aggregation in solution than β-lactoglobulin, due to the lower magnitude of the potential. This may result in a more favourable deposition from the solution onto the tissue in the case of casein. Additionally, washing from the tissue surface may be less favoured, due to the lower affinity for solution. The higher viscosity of casein solution could also be an important factor behind its greater binding to the oral epithelium, as increased viscosity has been shown to enhance adhesion properties and prolong retention.33 Finally, the tongue shows variable hydrophobicity depending on the presence of saliva34 which indicates that hydrophobic interaction with mucoadhesives may be possible. Studies35,36 have demonstrated that the β-lactoglobulin has more hydrophobic character than casein so, whilst this hydrophobicity may play a role in mucoadhesion, it does not seem to be the crucial factor in this study. Throughout all of this analysis, it is also important to remember the micellar nature of casein almost certainly results in a very different nature of interaction than that seen for β-lactoglobulin.
Although fluorescent microscopy found milk proteins adhere to mucosal surfaces in the mouth, the standardised nature of this technique may not be fully representative of the consumption of high protein dairy-based beverages. Further trials into the mucoadhesive properties of milk proteins in vivo would help fully elucidate the effect of oral movements and forces on the binding and retention of casein and β-lactoglobulin to the oral mucosa. Previous studies found milk proteins to be associated with astringency; from potential binding of salivary proteins,5 the precipitation of proteins at pI,7 to the presence of mouth drying proteolysis breakdown products.37 However, the association between mucoadhesion of dairy proteins and these drying mechanisms has not widely been investigated. In a recent study, Ye et al.38 assessed the adhesion of β-lactoglobulin and lactoferrin to human buccal cells using enzyme-linked immunosorbent assay (ELISA) to judge astringency. The study found binding of 1.0% β-lactoglobulin to the mucosa at pH 7.4 which agrees with the results reported in this work.
Conclusions
This investigation found both casein and β-lactoglobulin to adhere strongly to the epithelial lining of porcine oral mucosa, with casein binding more efficiently than β-lactoglobulin. Some factors behind the differences in binding were investigated, with zeta-potential and viscosity being suggested as the driving factor behind the greater adhesion of casein. This study found that the milk proteins bound directly to the oral mucosa, and washing with artificial saliva, equivalent to saliva production for up to 50 minutes, did not remove the protein from the buccal mucosa or tongue apex. This suggests that milk proteins can be predicted to adhere to the oral epithelium for up to 50 minutes in vivo. These findings correlate with to the build-up of mouth drying observed during the repeat consumption of protein rich beverages assessed through sequential profiling over twenty minute period.3 Therefore, this lack of protein clearing may be a cause or at least contribute to the build-up in mouth drying and could be limiting factor in the enjoyment of milk protein enhanced beverages.39Milk proteins exhibited varied ability to retain on different mucosal surfaces in the mouth. Better retention of the proteins was observed on buccal mucosa and tongue apex compared to tongue posterior and edges. This difference could be related to the structural organisation of these tissues. Examining selected physicochemical properties of the mucoadhesive proteins suggested that both viscosity and zeta potential may be important parameters in mucoadhesion, the latter representing both electrostatic interaction (repulsion in this case) and affinity for salivary solutions.
Acknowledgements
This research was funded by Nestec Ltd and was also partially supported by the Leverhulme Trust (RPG-2013-017). The authors would like to acknowledge the advice and assistance of Kosai Al Khateb for rheology, Ellina Mun for Ellman's assay, Mike Saunders for useful discussions and Peter Morrison for tissue preparation and microscopy support. The authors are grateful to Professor Nicola Tirelli (University of Manchester) for providing access to zeta-potential measurements.References
- E. Jöbstl, J. O'Connell, J. P. A. Fairclough and M. P. Williamson, Biomacromolecules, 2004, 5, 942–949 CrossRef PubMed.
- D. Rossetti, G. E. Yakubov, J. R. Stokes, A.-M. Williamson and G. G. Fuller, Food Hydrocolloids, 2008, 22, 1068–1078 CrossRef CAS PubMed.
- Z. Rahemtulla, C. Baldwin, A. Spiro, C. McGough, A. R. Norman, G. Frost, D. Cunningham and H. J. N. Andreyev, Clin. Nutr., 2005, 24, 1029–1037 CrossRef CAS PubMed.
- L. Methven, K. Rahelu, N. Economou, L. Kinneavy, L. Ladbrooke-Davis, O. B. Kennedy, D. S. Mottram and M. A. Gosney, Food Quality and Preference, 2010, 21, 948–955 CrossRef PubMed.
- A. Mimouni, H. C. Deeth, A. K. Whittaker, M. J. Gidley and B. R. Bhandari, Food Hydrocolloids, 2009, 23, 1958–1965 CrossRef CAS PubMed.
- M. Kelly, B. Vardhanabhuti, P. Luck, M. A. Drake, J. Osborne and E. A. Foegeding, J. Dairy Sci., 2010, 93, 1900–1909 CrossRef CAS PubMed.
- H. Sano, T. Egashira, Y. Kinekawa and N. Kitabatake, J. Dairy Sci., 2005, 88, 2312–2317 CrossRef CAS.
- C. A. Lee and Z. M. Vickers, Int. Dairy J., 2008, 18, 1153–1156 CrossRef CAS PubMed.
- A. H. Shojaei, J. Pharm. Pharm. Sci., 1998, 1, 15–30 CAS.
- P. A. Gandhi, M. R. Patel, K. R. Patel and N. M. Patel, Int. J. Pharm. Res. Deliv., 2011, 3, 159–173 Search PubMed.
- M. M. Patel, J. D. Smart, T. G. Nevell, R. J. Ewen, P. J. Eaton and J. Tsibouklis, Biomacromolecules, 2003, 4, 1184–1190 CrossRef CAS PubMed.
- N. A. Fefelova, Z. S. Nurkeeva, G. A. Mun and V. V. Khutoryanskiy, Int. J. Pharm., 2007, 339, 25–32 CrossRef CAS PubMed.
- I. A. Sogias, A. C. Williams and V. V. Khutoryanskiy, Biomacromolecules, 2008, 9, 1837–1842 CrossRef CAS PubMed.
- V. V. Khutoryanskiy, Macromol. Biosci., 2011, 11, 748–764 CrossRef CAS PubMed.
- H. Ben-Aryeh, A. Shalev, R. Szargel, A. Laor, D. Laufer and D. Gutman, Biochem. Med. Metab. Biol., 1986, 36, 260–265 CrossRef CAS.
- F. Shama and P. Sherman, J. Texture Stud., 1973, 4, 111–118 CrossRef.
- E. E. Hassan and J. M. Gallo, Pharm. Res., 1990, 7, 491–495 CrossRef CAS.
- I. Bravo-Osuna, D. Teutonico, S. Arpicco, C. Vauthier and G. Ponchel, Int. J. Pharm., 2007, 340, 173–181 CrossRef CAS PubMed.
- G. S. Irmukhametova, G. A. Mun and V. V. Khutoryanskiy, Langmuir, 2011, 27, 9551–9556 CrossRef CAS PubMed.
- C. A. Squier and M. J. Kremer, J. Natl. Cancer Inst. Monogr., 2001, 29, 7–15 CrossRef.
- A. Mack, A. Singh, C. Gilroy and W. Ireland, Anat. Rec., 1998, 247, 33–37 CrossRef.
- C. Chamerro, P. de Paz, J. Fernandez and L. Anel, Scanning Microsc., 1993, 7, 313–322 Search PubMed.
- A. Bernkop-Schnürch, Adv. Drug Delivery Rev., 2005, 57, 1569–1582 CrossRef PubMed.
- L. K. Rasmussen, P. Højrup and T. Petersen, J. Dairy Res., 1994, 61, 485–493 CrossRef CAS.
- L. K. Rasmussen, P. Højrup and T. E. Petersen, Eur. J. Biochem., 1992, 207, 215–222 CrossRef CAS.
- S. Brownlow, J. H. Morais Cabral, R. Cooper, D. R. Flower, S. J. Yewdall, I. Polikarpov, A. C. North and L. Sawyer, Structure, 1997, 5, 481–495 CrossRef CAS.
- Y. D. Livney, Curr. Opin. Colloid Interface Sci., 2010, 15, 73–83 CrossRef CAS PubMed.
- H. Hägerström and K. Edsman, Eur. J. Pharm. Sci., 2003, 18, 349–357 CrossRef.
- A. Semalty, M. Semalty, R. Singh, S. K. Saraf and S. Saraf, Indian J Pharm. Sci., 2007, 69, 741–747 CrossRef CAS PubMed.
- S. Poole, S. I. West and C. L. Walters, J. Sci. Food Agric., 1984, 35, 701–711 CrossRef CAS.
- R. G. Jensen, B. Blanc and S. Patton, in Handbook of Milk Composition, ed. R. G. Jensen, Academic Press, 1995 Search PubMed.
- P. F. Fox, Milk Proteins: General and Historical Aspects, Kluwer Academic, Plenum Publishers, New York, 3rd edn, 2003 Search PubMed.
- F. Nakamura, R. Ohta, Y. Machida and T. Nagai, Int. J. Pharm., 1996, 134, 173–181 CrossRef CAS.
- H. Ranc, A. Elkhyat, C. Servais, S. Mac-Mary, B. Launay and Ph. Humbert, Colloids Surf., A, 2006, 276, 155–161 CrossRef CAS PubMed.
- D. L. Sackett and J. Wolff, Anal. Biochem., 1987, 167, 228–234 CrossRef CAS.
- B. Hiller and P. C. Lorenzen, J. Agric. Food Chem., 2008, 56, 461–468 CrossRef CAS PubMed.
- V. R. Harwalkar, H. Cholette, R. C. McKellar and D. B. Emmons, J. Dairy Sci., 1993, 76, 2521–2527 CrossRef CAS.
- A. Ye, T. Zheng, J. Z. Ye and H. Singh, Physiol. Behav., 2012, 106, 645–650 CrossRef CAS PubMed.
- M. Gosney, J. Adv. Nurs., 2003, 43, 275–280 CrossRef.
Footnote |
† Electronic supplementary information (ESI) available: Scheme of the regions of porcine tongue dissected for tissue specimens, TLC of labelled proteins and DLS of casein solutions. See DOI: 10.1039/c3fo60291e |
|
This journal is © The Royal Society of Chemistry 2013 |