DOI:
10.1039/C3BM60058K
(Review Article)
Biomater. Sci., 2013,
1, 1012-1028
Applications of nanostructured calcium phosphate in tissue engineering
Received
4th March 2013
, Accepted 16th July 2013
First published on 9th August 2013
Abstract
Nanostructured calcium phosphate (CaP) is an important biomaterial for hard tissue repair with potential applications in some soft tissues as reported by recent studies. Herein, we shed light on recent research progress with respect to the biological effects of nanostructured CaP in some specific biological systems, including bone, cartilage, blood vessels, and nerve repair or regeneration. Specific cellular and molecular biological effects of nanostructured CaP and nanostructured CaP-based composites on numerous types of cells are summarized. Finally, the cytotoxicity and inflammatory response of nanostructured CaP are discussed.
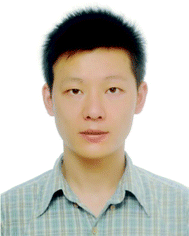 Changchun Zhou |
Changchun Zhou, Assistant Professor of the National Engineering Research Center for Biomaterials (NERCB), Sichuan University, China. From 2004 to 2011 he studied materials science for a masters degree and mechanical engineering for a doctoral degree at Sichuan University. During 2008–2010 he studied at the University of Washington (Seattle) and the University of Texas as a joint training doctoral student. After receiving his PhD in 2011 he joined the NERCB. His research focuses on nanostructured biomaterials and their applications. |
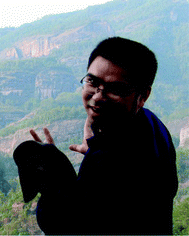 Youliang Hong |
Youliang Hong, Professor of Biomaterials, National Engineering Research Center for Biomaterials, Sichuan University. Dr Hong got his B.S. and Ph.D. degrees in Chemistry from Jilin University, and then completed his Postdoc work in the State Key Laboratory of Polymer Physics and Chemistry, Changchun Institute of Applied Chemistry in 2007. His recent research interests focused on biomaterials and regenerative medicine, including biopolymer/inorganic composites, bioceramics, micro-/nanobiomaterials, drug delivery, material–cell interactions, and mechanotransduction. |
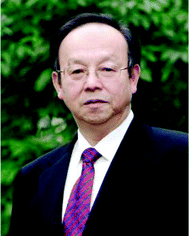 Xingdong Zhang |
Xingdong Zhang, Professor and Honorary Director of the National Engineering Research Center for Biomaterials at Sichuan University in China, Academician of the Chinese Academy of Engineering and President of the Chinese Society for Biomaterials. He graduated from the department of physics at Sichuan University in 1960 and has been engaged in biomaterials studies since 1983. His academic research has focused on innovative biomaterials and medical implants for regenerative medicine and tissue engineering, especially on tissue-inducing biomaterials, calcium phosphate-based biomaterials, surface and surface modification of biomaterials, bone tissue engineering and implants for dental and orthopedic applications. He has received numerous awards, including the National Chinese Natural Science Award (2007), the Chinese National Science and Technology Progress Award (1998), Fellow of Biomaterials Science & Engineering, IUSBSE (2000), and the Chinese National Expert with Outstanding Contribution (1992). |
1 Introduction
Calcium phosphate (CaP), like hydroxyapatites (HA), tricalcium phosphate (TCP) and biphasic calcium phosphate (BCP), has been widely used in the biomedical field because of its excellent biocompatibility and bioactivity.1–4 CaP is considered a preferred candidate for tissue repair, primarily because its composition is similar to the inorganic minerals of bone. Both in vitro and in vivo experiments have demonstrated that the biological performances of nanostructured CaP are excellent compared to conventional CaP.5–7 For example, bioceramics consisting of CaP nanocrystals have exhibited excellent mechanical properties and high bioactivities, which improve the viability and proliferation of the bone-relevant cells.5,8In vivo experiments have shown that biological effects, including protein adsorption, regulation of the osteogenic gene expression, biological degradation, biological risk and anticancer activity, have been demonstrated to be significantly different from conventional CaP ceramics.6,9–11 It is well established that the nanocrystalline forms of CaP can mimic both the chemical components and structure of calcified tissues. Thus, they are beneficial to biomineralization in hard tissue regeneration.12 Furthermore, due to their small size and large specific surface area, the matrices constructed by these CaP nanocrystals affect cellular attachment and the subsequent biological behaviour.13,14 Arnold et al. demonstrated that cellular adhesion sites are in the range of 5–200 nm, which indicates that very small components of a cell are influenced mainly by nanoscale rather than microscale substrates.15 Other findings16–18 have also showed that the presence of CaP nanocrystals can significantly increase the adsorption of proteins, adhesion and gene expression of osteoblasts.19–21 Therefore, it is believed that nanostructured CaP has great potential to improve the biological performance of tissue engineering scaffolds.22
It is crucial to understand the cellular and molecular biological effects of nanostructured CaP in order to better develop it for use. Some specific biological effects of nanostructured CaP have already been investigated at the cellular and molecular levels.23–25 The observed biological effects of CaP changed profoundly in various tissues when CaP was processed into nanostructures. The primary reasons that nanosized CaP has improved the biological performance over larger microsized CaP is not only because its chemical components are similar to the inorganic minerals of hard tissue, but also because biologically formed CaP has a nanocrystalline structure.26 It has been reported that HA crystals with diameters of 25–50 nm can accelerate the formation of biominerals, which is essential in terms of the mechanical properties and bioresorbability of bone.27 Moreover, it has been reported that the basic inorganic building blocks of teeth and bone are nanosized apatite particles. Although the hierarchical structures of bone and teeth are different from each other, they share similar inorganic building blocks consisting of apatite nanoparticles.28 It has also been observed by atomic force microscopy (AFM) in trabecular bone and vertebrae that nanosized plates or ball shaped minerals are densely packed in collagen fibrils,29,30 and their diameters are in the range of 30–120 nm.31 Therefore, some researchers have argued that apatite nanoparticles with smaller sizes are likely to be closer to natural apatite than larger apatite particles during biomineralization.32,33 The nanosized apatite can mimic biological structures of hard tissue so as to display the biological properties and functions of natural tissues.34–36
Besides its application in hard tissue engineering, nanosized CaP, especially nanocrystalline HA, has recently been widely studied for soft tissue repair. Some results have confirmed that the addition of HA nanocrystals in cartilage scaffolds can improve cellular adhesion and proliferation significantly, thus contributing to the repair and regeneration of cartilage.37–39 Adequate blood supply is a prerequisite for successful biomaterial–tissue interactions. Angiogenesis plays a key role in tissue growth, development, and repair.40,41 Previously, works have reported that HA nanocrystals could up-regulate the angiogenic signal pathways and some pivotal growth factors of angiogenesis.42,43 HA nanocrystals have demonstrated their ability to influence the formation of blood vessels, and maintain or stimulate their survival, morphology and subsequent functions. HA nanocrystals were also found to play a role in nerve repair by inducing axonal outgrowth and formation.44–46 Therefore, HA nanocrystals could possibly become a useful promoter for nerve repair or regeneration.
The cytotoxicity of nanosized CaP remains an important issue for applications in tissue engineering. Although nanosized CaP has been closely studied, understanding the interactions between CaP nanoparticles and living cells remains a challenge. The influence of nanoparticles on human health needs more investigation. Any clinical applications of nanosized CaP need to be closely scrutinized and verified before implementation. Many experiments have confirmed that the behaviour of cells exposed to micro-/macro-particles are quite distinct from the behaviour expressed in the presence of nanoparticles.7,47 When materials are tailored into nanoscale building blocks, they might induce cytotoxicity and lead to negative effects in organisms. The parameters that may influence nanoparticle cytotoxicity include concentration, size, morphology and structure.5,48–51 Additionally, different types of target cells experience different degrees of cytotoxicity and different mechanisms of toxicity when exposed to nanoparticles.52 For nanostructured CaP, although it can be potentially cytotoxic, the cytotoxicity is dependent on the target cells. This biological characteristic of nanosized CaP has attracted some researchers to use it in attempts to cure some cancer-related diseases. Interestingly, some recent results have shown that HA nanocrystals could indeed inhibit the proliferation of several types of cancer cells, including osteosarcoma, liver and throat cancer cells, while showing few side effects on normal cells.21,53,54
Herein we highlight recent progress of nanostructured CaP in different tissue engineering applications including bone, cartilage, blood vessels, and nerve tissue. We focus particularly on its biological effects at the cellular and molecular levels. However, this review does not consider the applications of nanosized CaP in medicines, e.g. drug delivery, gene transport, etc. Readers who are interested in the use of nanostructured CaP as drug/gene delivery systems should read recent reviews summarized by Chen, Epple, Kateb et al.55–57
2 Nanostructured CaP
In the biomaterials field, there are two different kinds of nanomaterials: nanobiomaterials and nanostructured biomaterials.58 Nanobiomaterials are defined as those biomaterials limited to the single molecule level, such as a single protein, while nanostructured biomaterials refer to any biomaterials whose structural elements, e.g., clusters, crystallites or molecules, have nanoscale dimensions (i.e. the size in any dimension is in the range of 1–100 nm).59 For example, nanostructured CaPs are calcium phosphate materials whose structural elements are in the range of nanoscale dimensions. Similarly, nanocrystalline CaPs are CaP materials that consist of many crystals, the majority of which have one or more dimensions in the order of 100 nm or less.60–62 CaP nanoparticles are defined as those CaP particles with a diameter of 100 nm or less.63 As a common biomaterial, nanostructured CaP may be used in various forms in tissue engineering. The development of nanotechnology has aided the development of nanoscale CaP. Up to now many methods have been developed to prepare various sizes and forms of nanoscale CaP, including the co-precipitation method, sol–gel synthesis, hydrothermal reaction, mechanical milling, and microwave sintering. For example, the application of CaP nanoparticles, CaP cements, CaP nanoparticle-based composites (the CaP nanoparticles mix and disperse into other matrixes), coating layers, nanoceramics, etc., have been reported using the above methods.4,64–70 These materials were developed by the strategy that nanostructured materials possess specific features that allow the materials to affect or control cell behaviours. Fig. 1 shows different morphologies of nanostructured CaP.71–73
The biological performance of nanostructured CaP depends strongly on its composition, structure, morphology and crystallite sizes. These biological characteristics include the biocompatibility, bioactivity, stability and mechanical properties of nanosized CaP.74 The CaP morphology may have an influence on the biological effects such as protein adsorption, cell viability and proliferation, angiogenesis and vascularization, cytotoxicity and apoptosis, etc.
It is generally accepted that nanosized CaP has better biological performances than conventional materials because it has a higher specific surface area and specific surface roughness. For example, nanophase HA appeared to absorb 11% more fetal bovine serum proteins per 1 cm2 than conventional HA.75 Cellular proliferation on a nanocrystalline HA substrate is significantly higher (2.6 times higher) than that on a conventional HA substrate.31 The resorption and degradation of nanocrystalline CaP ceramics are also quite different from those of the conventional ones.76,77 Furthermore, due to smaller crystalline grains and a greater specific surface area, nanosized CaP shows improved sinter-ability and densification, which may improve fracture toughness as well as other mechanical properties.78,79
3 The cellular and molecular biological effects
When biomaterials are tailored into nanoscale building blocks, many researchers have shown that some novel and special cellular and molecular biological effects can be achieved. Also, significant influences of nanostructured CaP on the cellular biological behaviours and responses have been reported. Cells are sufficiently sensitive to nanosized substrates and elicit the corresponding biological behaviours and responses.80 One possible explanation is that the ultrathin sizes of nanosized CaP can trigger the threshold of the cellular response.11 It is believed that nanostructured CaP can promote protein adsorption and influence cellular biological behaviours such as cellular adhesion, survival, morphology, migration and proliferation. Some special phenomena include inflammatory response, cytotoxicity resulting from exposure to nanostructured CaP, and various cellular responses such as up-regulating angiogenic signaling pathways, regulation of osteogenic gene expression, promotion of proangiogenic signals, and mediation of netrin 1, have also been reported.77,81–83Table 1 shows the applications of nanostructured CaP in tissue engineering and its biological effects. These phenomena show that nanostructured CaP may promote the rapid repair of tissue injury. Therefore, understanding the cellular and molecular biological effects of nanosized CaP in different tissues is very important for its future applications in implant devices. In the following sections, we will discuss the applications of nanostructured CaP in hard tissue, cartilage, blood vessels, and nerve tissue, respectively.
Table 1 Applications of nanostructured CaP in tissue engineering and its biological effects
Nanostructured CaP |
Applications in tissue engineering |
Biological effects |
CaP nanoparticles |
Hard tissue |
Influence protein adsorption |
CaP cements |
Cartilage |
Influence cells biological behaviour, including cellular adhesion, survival, morphology, migration, proliferation, etc. |
Nano-CaP composites |
Angiogenesis & vascularization |
Influence cells biological response, including up-regulating angiogenic signaling pathways, regulation of osteogenic gene expression, promotion of proangiogenic signals such as eNOS and FGF-2, mediation of netrin 1 |
Nano-CaP coating layers |
Nerve tissue |
Inflammatory response |
CaP nanoceramics |
|
Cytotoxicity |
3.1 Hard tissue
Natural bone consists of approximately 30% organic components and 70% rigid nanosized minerals.79,84 At the beginning of bone formation, its mineral phase, deposited around the protein fibers, is composed of nanosized, amorphous CaP (A-CaP). The A-CaP then matures to form crystals with specific nanostructures. Anatomical studies have confirmed that the sizes of bone crystals are in the range of 30–50 nm (length), 15–30 nm (width) and 2–10 nm (thickness).85 Tens to hundreds of these nanoscale building blocks are organized into organic protein matrices through specific models to endow natural bone with unique structural and physiological features such as porosity, mechanical strength, flexible toughness and insensitivity to growth/dissolution.32,86 Therefore, nanosized apatite is structurally closer to the mineral phase of natural bone than microsized apatite, and thus it is expected to have a better biological performance to repair bone defects.
Calcium phosphate cements (CPCs) have been successfully used as materials for hard tissue repair. As an alternative to sintered porous CaP ceramics, CPCs take advantage of the fact that these products have better formability, and the final product is a low temperature precipitated hydroxyapatite, which shows higher activity and a similarity to biological apatite. Some studies have pointed out that the surface properties of bioactive materials play crucial roles in affecting cell behaviours. Therefore, new strategies toward the design of substrates with specific features that can affect or control cell behaviours become promising approaches. Ginebra et al. studied the cellular biological effects on the micro- and nanostructural features of CPCs.87,88 They found that some specific responses in biological phenomena such as protein adsorption and cell adhesion, which strongly depended on the structure of the interface at nanoscale levels. The differences in the micro-/nanostructures at the surface must elicit very different cell responses. Cell proliferation and differentiation are strongly enhanced when cells are cultured on the finer substrates (nanoscale size). This phenomenon is attributed strictly to topographical effects. On the one hand, the higher specific surface area of a finer substrate provides more sites for adsorption of serum proteins from the culture medium. The concentration of these molecules at the surface can mediate the expression of the osteoblastic phenotype and the production of significant amounts of bone proteins. On the other hand, the higher dissolution of Ca2+ from the finer substrates can also improve the expression of the cellular osteoblastic phenotype and the formation of calcium nodules.
Porous CaP ceramics are another kind of important biomaterials candidate for the repair of hard tissues. However, the poor mechanical properties of traditional CaP ceramics limit their applications in load-bearing bone defect sites. Therefore, some researchers have tried to develop CaP nanoceramics to solve this problem. Bose et al. reported micro- and nanostructured HA ceramics, which were prepared using microwave sintering and had average grain sizes ranging from 168 ± 86 nm to 1.16 ± 0.17 μm. The compressive strength and cell activity of the HA ceramics increased in inverse proportion to their grain size.81 The adhesion and proliferation of bone cells increased largely with the decrease of HA grain size. Lin et al. reported that the maximal values of bending strength, elastic modulus, Vickers hardness and compressive strength of nanosized β-TCP samples were two times higher than those of microsized β-TCP.89 The degradability of microsized β-TCP ceramics was just about one-quarter of that of the nanosized ceramics. In addition, HA nanocrystals have also been used as coating layers.90–93 Roohani-Esfahani et al. investigated the effects of HA nanocrystals on the physical and biological properties of BCP scaffolds.94 They found that although the porosity of the BCP scaffolds decreased about 4% after coating, their macroporous structures were not influenced in essence, and the thin coated layer improved the mechanical and biological properties of the scaffolds. They also demonstrated that coated bioactive HA nanocrystals on BCP scaffolds induced differentiation of primary human bone-derived cells (HOBs), which significantly up-regulated osteogenic gene expression, including Runx2, osteopontin and bone sialoprotein.
Many results have shown that the size,31,97,98 shape5,99 and morphology26,95,96 of nanostructured CaP and nanostructured CaP-related materials affects the cellular response and subsequent biological effects.100,101 Qiang et al. studied the cell responses to different shapes of HA nanocrystals.5 The proliferation experiments of L929 cells demonstrated that spherical HA nanoparticles are more biocompatible than needle-shaped ones. Shi et al. studied the size effects of HA nanocrystals on the cell responses.31 In their experiments, HA crystals of micrometer size (m-HA) and two kinds of nanometer sizes 20 nm (np20) and 80 nm (np80) were used to investigate their biological response to human osteoblast-like MG-63 cells. According to the cell proliferation assay results (Fig. 2A), the proliferation of the MG-63 cells on both HA nanocrystal substrates was significantly higher than that on the m-HA substrate after 3 and 5 days of incubation. After 5 days incubation, the number of MG-63 cells on glass (control group), np20, np80 and m-HA were 357 ± 34, 299 ± 24, 215 ± 21 and 135 ± 13 mm−2, respectively, which is consistent with the results of AO staining (Fig. 2B). These results demonstrated that cellular proliferation is closely related to the size of the HA crystals. The decrease of HA crystal size promotes the proliferation of MG-63 cells.
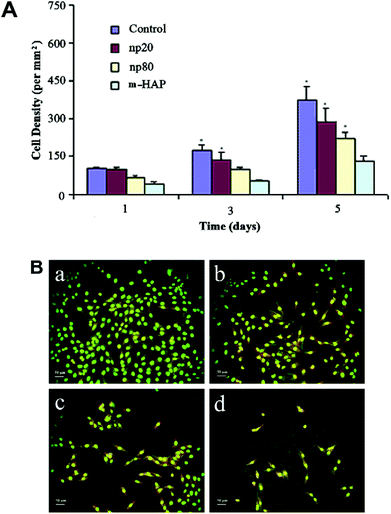 |
| Fig. 2 The size influence of HA nanocrystals on cellular proliferation. (A) Cell density of MG-63 on different substrates for 1, 3 and 5 days. (B) Fluorescence microscopic photographs of MG-63 cells (cultured for 5 days) on (a) glass, (b) np20, (c) np80 and (d) m-HAP.31 | |
In addition, some recent work also demonstrated that the crystallinity of CaP can affect the cell response.26,95,96 It is generally accepted that A-CaP can be adsorbed and assimilated more easily in vivo than well-crystallized CaP. However, when the size of the crystal CaP grains is reduced to a nanoscale size, cells adsorb and proliferate on the well-crystallized HA rather than on the A-CaP. The experimental results (Fig. 3) showed that differentiation of MSCs from osteoblasts is promoted significantly by HA nanocrystals. Thus, the crystallized phase of nanosized CaP provides a better substrate for MSCs than the amorphous substrate, excluding morphological factors.
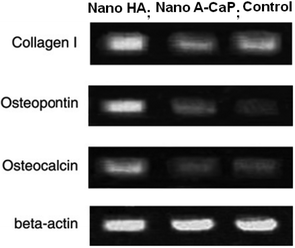 |
| Fig. 3 The crystallinity of CaP affects the cellular response. Agarose electrophoresis gels showing relative collagen I, osteopontin, osteocalcin and beta-actin expression levels studied by RT-PCR. The cells were seeded onto the films at a density of 6 × 103 cm2 and cultured for two weeks. The substrates of CaP have similar sizes ∼20 nm.26,96 | |
The size of nanostructured CaP which can affect cell behaviours profoundly is in the range of 5–200 nm. This suggests that very small components of a cell can be influenced on the nanoscale rather than on the microscale.15 Cells are very sensitive to nanosized substrates that elicit diverse biological behaviours. But how cells can identify the particle size and the underlying relative biological mechanisms is still unclear.102,103 The size, shape and morphology of particles decide the surface properties of CaP and in turn they affect the behaviours of the attached cells.104 Other findings showed that the introduction of CaP nanoparticles can significantly increase the protein adsorption, adhesion and gene expression of osteoblasts.20,105–109 These results indicate that the addition of nanostructured CaP has a positive effect on bone regeneration.
3.2 Cartilage
Historically, the repair of articular cartilage damage caused by injury has been difficult since articular cartilage is avascular.110 Without access to abundant nutrients or circulating progenitor cells and by possessing a nearly acellular nature, cartilage lacks the innate abilities to mount a sufficient healing response. Thus, the damaged tissue is not replaced with functional tissue and requires surgical intervention. Traditional techniques for cartilage repair include marrow stimulation, allografts, and autografts. However, each of these techniques has limitations. Marrow stimulation results in fibrocartilage of inferior quality that does not persist; allografts suffer from lack of integration, loss of cell viability due to graft storage, and concerns of disease transmission; autografts also lack integration and require additional defects.111,112 To avoid the use of auto-/allografts, numerous tissue engineering scaffolds have been attempted and many promising man-made biomaterials, especially bioceramic/polymer composites, e.g. poly-(lactide-co-glycolide) (PLGA),113 poly(vinyl alcohol) (PVA) hydrogel,114,115etc., have been proposed for cartilage regeneration.116,117 Due to their biocompatibility and osteoconductivity, HA nanocrystals have been widely studied and recognized as an important additive in cartilage regeneration.38,118
Some results have confirmed that the addition of HA nanoparticles could significantly improve cellular attachment and proliferation in cartilage scaffold composites. Cheng et al. reported that human cartilage cells attached and proliferated well on PLA/HA composites in which HA nanocrystals are homogeneously dispersed in PLA matrices.119 Xue et al. studied the biological effects of HA nanoparticles contained in PLGA scaffolds both in in vitro and in vivo models.120 In the in vitro study, the CLSM observation, MTT assay, and DNA assay of MSC growth all suggested that cartilage repair with the PLGA/HA scaffolds was superior to pure PLGA scaffolds. An in vivo study (Fig. 4) further compared these two scaffolds incorporated with MSCs for cartilage regeneration. The results showed that the viability and proliferation of MSCs in PLGA/HA scaffolds were significantly superior to in pure PLGA scaffolds. It was observed in PLGA/HA scaffolds that mature and well-developed hyaline cartilaginous tissues containing abundant glycosaminoglycan and collagen II accumulated. After being implanted for 12 weeks in vivo, the PLGA/HA scaffolds showed the best regeneration of cartilage defects in the rat model. The in vivo studies showed that the polymer/HA composite scaffolds may be suitable for cartilage repair. The PLGA/HA scaffolds could achieve a satisfactory osteochondral repair without adding any growth factors or gene transfer in the animal articular cartilage damage model.121
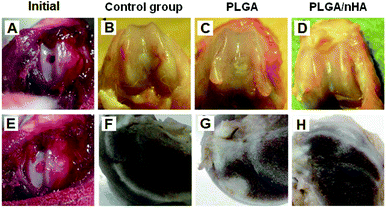 |
| Fig. 4 Photographs of rat articular cartilage defects after immediate creation (A) and after treatment with scaffolds (E). Gross appearance of the repaired cartilage at 12 weeks post-operation in the control group (B and F), pure PLGA group (C and G) and PLGA/HA nanoparticle group (D and H). Images (B–D) were front views and images (F–H) were cross-section views.120 | |
Because articular cartilage tissues are often exposed to friction, PVA hydrogel is proposed for cartilage repair due to its good biotribological properties.114,115,122,123 Some researchers have hypothesized that the incorporation of HA nanoparticles into PVA cannot only improve the mechanical properties of the composites but also endow the composites with excellent bioactivities. Pan et al. studied the mechanical properties of HA nanoparticle reinforced PVA gel composites as cartilage repair biomaterials.38,118,124 They found that by adjusting the amount of HA nanoparticles used, the PVA concentration and the freeze–thaw cycle, PVA/HA gel composites with optimal viscoelastic behavior can be obtained. For PVA/HA gel composites used in articular cartilage repair, the optimum preparation process of PVA/HA gel composites reported is that 6% of the HA nanoparticles are added into 20% of the PVA matrix and the freeze–thaw cycles are repeated 5 times. It is well known that the mechanical properties of polymers are improved by adding inorganic nanoparticles into the polymer matrix due to their high mechanical strength and surface energy.38,125 Thus, the addition of HA nanoparticles in the PVA gel matrix can increase the mechanical strength of the gel composites. Furthermore, due to the presence of the HA nanoparticles, the implanted materials may help to form a bone-bond and improve the adhesion properties between the implanted material and the natural tissue. The mechanical properties such as the tensile, compressive, and viscoelastic behaviour of PVA/HA composites can be well designed to mimic natural articular cartilage.126 Therefore, incorporating HA nanoparticles into polymers cannot only improve the mechanical properties of the composites but also endow the composites with excellent bioactivities in cartilage regeneration.
3.3 Angiogenesis and vascularization
In addition to bone and cartilage repair, nanostructured CaP has special functions to promote angiogenesis and vasculogenesis.42,127,128 The formation of blood vessels is essential to providing the site of the biomaterial implantation with oxygen, nutrients, soluble factors and cells to ensure the evacuation of metabolites.129–131 Angiogenesis and vasculogenesis are the mechanisms responsible for the development of blood vessels. Angiogenesis refers to the formation of capillaries from pre-existing vessels in the embryo and adult organism, while vasculogenesis is the development of new blood vessels from the differentiation of endothelial precursors (angioblasts) in situ. Angiogenesis and vasculogenesis, occurring during bone development, are a prerequisite for normal osteogenesis.132 During bone repair, the process of angiogenesis precedes osteogenesis. The newly formed vessels drive the orientation of the bone microcolumns, which activates and controls prospective tissue functions.133 Since blood supply and metabolite evacuation are necessary for successful biomaterial–living tissue interactions, angiogenesis and vasculogenesis contribute key functions for new tissue development, growth, and regeneration.134
The biological effect of HA nanocrystals on angiogenesis has been studied by Pezzatini et al.42 The pro-survival and pro-angiogenic results of nanostructured HA suggested that this material has the ability to influence the biological function of microvascular endothelial cells. Nanostructured HA shows excellent biocompatibility for endothelial cells, as it appears to favor the development of the angiogenic potential of these cells and promote vascularization. From their studies, a wide range of concentrations (from 2 to 500 μg mL−1) of nanostructured HA were tested for survival, migration and morphology of endothelial cells. They found that the nanostructured HA induced a migratory phenotype in endothelial cells, which is crucial for the occurrence of angiogenesis. After investigation by the MTT test with respect to the survival of endothelial cells exposed to nanostructured HA for a long time, it was found that nanocrystalline HA sustained the survival and proliferation of endothelial cells. Long-term exposure (7 days) to low concentrations (2–5 μg mL−1) of HA nanocrystals promotes the proliferation of endothelial cells and preserves their morphology. Following the prolonged exposure time, exposure to a high concentration of the HA nanocrystals (100–500 μg mL−1) was also non-toxic, but the cellular morphology changed with the elongation of the cell body and formation of filopodia.126
Some works reported that HA nanocrystals can up-regulate angiogenic signaling pathways and some important growth factors of angiogenesis. For example, endothelial cells express endothelial nitric oxide synthase (eNOS) and fibroblast growth factor-2 (FGF-2). The eNOS is a marker for physiological vascular function, and FGF-2 controls the activation of the endothelial cell toward an angiogenic phenotype.135–138Fig. 5 shows the up-regulation of eNOS in coronary venular endothelial cells (CVECs) in the presence of HA nanocrystals. In the control conditions (Fig. 5A), the microvascular endothelial cells showed a typical cytoplasmatic distribution of eNOS in small membrane-associated vesicles, resembling caveolae, as well as in the Golgi complex as described previously.128,139Fig. 5(B–D) show the eNOS localization and expression in CVECs after 4 days cultured in the presence of HA nanocrystals. These pictures show an increase in the fluorescence intensity in the perinuclear region. This means the eNOS was up-regulated in these HA nanocrystal-exposed samples.
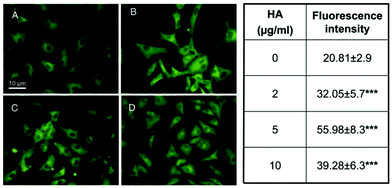 |
| Fig. 5 The eNOS expression in CVECs exposed to HA nanocrystals for 4 days. The effect of 2 mg mL−1 (B), 5 mg mL−1 (C) and 10 mg mL−1 (D) of HA nanocrystals compared to the control condition (A). The mean values of the eNOS signals of the cells treated with the HA nanocrystals (2, 5 and 10 μg mL−1) are reported in the right hand table. The fluorescence intensity in the treated samples was significantly higher than in the untreated ones, confirming the up-regulation of eNOS.42 | |
Fig. 6 shows the phenomena of the FGF-2 up-regulation. The endothelial cells maintained their survival through the expression/production of autocrine factors, predominantly FGF-2. The mRNA of FGF-2 was measured by quantitative real-time polymerase chain reactions (RT-PCRs), as shown in Fig. 6A. After 6 hours of exposure, nanocrystalline HA induced an increase in the FGF-2 gene expression (corrected for actin transcription) compared to in the control conditions. At a longer exposure time (18 h) the FGF-2 expression declined, but continued to be higher than for the control samples. The production of the FGF-2 protein in CVECs was studied by Western blotting analysis for 18, 72 and 96 hours of exposure to nanocrystalline HA (Fig. 6B). The CVECs expressed all different isoforms of FGF-2 (18, 22 and 34 kDa isoforms). The exposure of the CVECs to nanocrystalline HA for 72 h significantly increased the production of the 18 kDa FGF-2 isoform. The expression and localization of FGF-2 were measured by immunofluorescence. In the control sample (Fig. 6C), the protein labeling was cytoplasmatic with a web-like pattern, resembling the distribution of the endoplasmic reticulum.140 After exposure to the HA nanocrystals for 4 days (Fig. 6D–F), there was an increased intensity in the FGF-2 labeling, especially at the perinuclear level. These data indicate that the presence of nanocrystalline HA induces up-regulation of FGF-2, which controls the activation of endothelial cells toward an angiogenic phenotype.
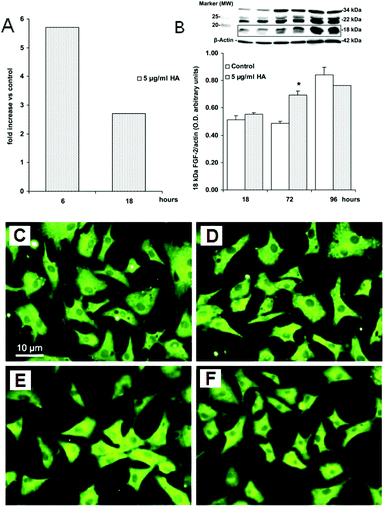 |
| Fig. 6 FGF-2 up-regulation in CVECs exposed to HA nanocrystals for 4 days. (A) The RT-PCR analysis of FGF-2 mRNA expression. CVECs were exposed to HA nanocrystals (5 μg mL−1) for 6 and 18 h. Data are reported as fold increases in the FGF-2 expression (corrected for actin transcription) over the control condition (0.1% serum). (B) The FGF-2 protein expression. Cell lysates were separated by 15% SDS-PAGE and FGF-2 expression was detected by Western blotting. Data of the 18 kDa isoform of FGF-2 are reported as the optical density of the ratio between the target protein and β-actin. (C) The control sample. (D–F) The expression and localization of the angiogenic factor FGF-2 were measured in CVECs grown for 4 days on glass coverslips by immunofluorescence. The concentrations of HA nanocrystals were 2 μg mL−1, 5 μg mL−1 and 10 μg mL−1, respectively.42 | |
Pezzatini et al. studied the endothelial cell organization and differentiation toward angiogenic phenotype induced by nanocrystalline HA.42 They tested the capacity of nanocrystalline HA to support the differentiation of microvascular cells in a three-dimensional protein matrix by mimicking the physiological conditions of fibrin clots in sites of natural bone tissue. They found that at a low concentration (2–10 μg mL−1), HA nanocrystals are biocompatible with microvascular endothelial cells and stimulate a pro-angiogenic phenotype. The cells also have an enhanced response to the vascular endothelial growth factor (VEGF), which induces the organization of endothelial cells into capillary-like structures. Thus, nanocrystalline HA promotes endothelial cell organization toward an angiogenic phenotype.
In summary, HA nanocrystals show excellent biocompatibility with endothelial cells. They interact with the endothelium and promote proangiogenic signals, such as eNOS and FGF-2. Nanocrystalline HA appears to stimulate the development of the angiogenic potential of endothelial cells and promote vascularization.
3.4 Nerve tissue
Nerve injury is a serious problem for patients. A traumatic axonal injury (TAI) is considered to be a significant characteristic of traumatic brain injury (TBI).141,142 An axonal injury causes direct transection or cortical contusions, which commonly result in neuronal death.143 Nerve regeneration and functional recovery are major issues after a nerve injury.144,145 Some nanomaterials have been used for axonal regeneration after nerve injuries.146–148 Among them, nanocrystalline HA is attractive due to its excellent biocompatibility and bioactivity. Some studies with regards to nanocrystalline HA have been proposed in nerve tissue engineering.148
Axonal guidance growth is important for nerve plasticity and axonal regeneration. Netrin 1 is one of the axonal guidance cues which has been found to play an important role in the development and regeneration of the central nervous system (CNS).149 Some previous studies showed that axon outgrowth was mediated by netrin 1, which is one of the prototypical axonal guidance cues. Liu et al. utilized netrin 1 to investigate the protective effects of nanocrystalline HA against nerve injury.44 They cultured the cortical neurons of rats treated with HA nanocrystals or carbon nanotubes (CNTs). CNTs have also been proven to improve the axonal connection in a target direction during nerve regeneration.148 However, the neurotoxicity of CNTs limits their further applications in biological systems. Liu et al. found that both the HA nanocrystals and CNTs inhibited the expression of netrin 1 in the media. Down-regulation of netrin 1 indicated that the nanocrystalline HA or CNT treatments helped axonal outgrowth. This is because netrin 1 mediates axonal guidance growth in target cells or axon migration in a specific direction. Axons normally lose their response to attractants when they arrive at their targets where the attractant is produced, and hence they expressed down-regulation of netrin 1. This observation is also in agreement with previous studies.149,150
In addition, nanocrystalline HA had a significant effect on axonal formation. Liu et al. cultured the cortical neurons of rats after treatment with HA nanocrystals or CNTs.44 It was found that the axons grew well in the nanocrystalline HA group (see Fig. 7). In the immunocytochemistry assay, the MAP2 stained cytoskeleton (left panel), the DAPI stained nucleolus (middle panel), and a combination (right panel) show that compared to the control group (top panel, Fig. 7A), the cell density decreased (Fig. 7B) and the axons grew significantly longer (Fig. 7C) after the nanocrystalline HA treatment. While the cells grew more concentrated, the cell density decreased significantly and the axons grew thinner after the CNT treatment. Compared to the CNT group, the axonal outgrowth was more obvious after the nanocrystalline HA treatment, and the cell density also increased significantly.
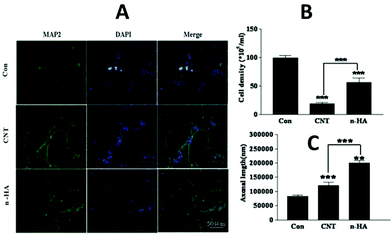 |
| Fig. 7 The biological effects of HA nanocrystals and CNTs on the cultured cortical neurons in the immunocytochemistry assay.44 | |
Therefore, nanocrystalline HA had a significant biological effect on axonal formation and nerve tissue engineering, and it has potential to be applied in nerve repair or regeneration.44
4 Cytotoxicity and inflammatory response
In recent years, a wide range of nanosized CaP biomaterials have been developed for a variety of applications due to their unique features.151,152 However, increasing evidence suggests that the special physicochemical properties of these nanomaterials also pose special biological effects and even potential risks to human health.153–155 Therefore, considerable efforts have been placed on studying the inflammatory response and potential toxicity to cells, tissues and organisms.157,158
Of the negative biological effects produced by nanostructured CaP, cytotoxicity remains the most critical. Biomaterials of a nanoscale size are possibly at the threshold of inducing different cytotoxicities. Recent studies displayed that the dosage (concentration), size, morphology or structure of HA nanoparticles have significant influences on the cellular biological behaviours and possibly arouse corresponding cytotoxicity.5,7,49–51 In addition, the target cell type is also critical in determining the intracellular response, degree of cytotoxicity and potential mechanisms of toxicity.52
As far as CaP nanoparticles are concerned, Zhao et al. reported that the length of rod-shaped HA nanocrystals (i.e. the particle size) can affect the particle–cell association and the generation of reactive oxygen species (ROS), which would ultimately determine their degree of cytotoxicity.7 Li et al. have shown that the size of the HA nanoparticles can influence the proliferation of tumor cells,156 while Cai et al. demonstrated that the cytotoxic effects of HA nanoparticles on mesenchymal stem cells (MSCs) and human osteosarcoma cells (U2OS) is dependent on the particle size and zeta potential.3,31 Moreover, the dosage and incubation time of the nanoparticle–cell also affects the degree of cytotoxicity. Zhao et al. demonstrated that the particle–cell biological effect varies with the particle dosage and exposure time.52 They studied a range of concentrations of HA nanoparticles (10–300 μg mL−1) with 4 to 24 hours of cell incubation times with respect to cytotoxicity. The results showed that a higher cytotoxicity is associated with a longer particle–cell incubation time. Cell death increased dramatically in direct proportion to the incubation time. Other available data from previous reports also suggest that a higher toxicity was associated with a higher dosage of the HA nanoparticles.157,158
Additionally, it is also accepted that the cytotoxicity of CaP nanoparticles is morphological dependent. Among the nanostructured CaPs with different morphologies such as plate, sphere, rod and needle, the CaP needles can lead to a serious inflammatory reaction and induce cells to produce inflammatory cytokines.159–161 The toxicity produced by needle-like CaP is speculated to be a result of the particle–cell interaction, which produces mechanical stresses on the cell’s surface.162,163 Also, irregularly shaped particles can produce responses of greater numbers and magnitudes than spherical particles. Zhao et al. investigated the morphology-induced cytotoxicity of CaP using four shapes of nanostructured HA: needle, plate, sphere and rod.7 In their studies, the effects of the nanostructured HA on the cytotoxicity, oxygen species generation, particle–cell association and cellular uptake were evaluated on BEAS-2B and RAW264.7 cells. ROS are often cited as the underlying cause of the nanoparticle-induced cytotoxicity. Cytotoxic assays (see Fig. 8) show significant differences in the cell viability of BEAS-2B among the different HA nanostructures, in which the HA needles and plates induced the highest cytotoxicities. However, regardless of shape, nanosized HA exhibited no significant toxicity to RAW264.7 cells. These results indicate that the cytotoxicity of nanosized HA is cell type-dependent at first and is further influenced by the particle morphologies.
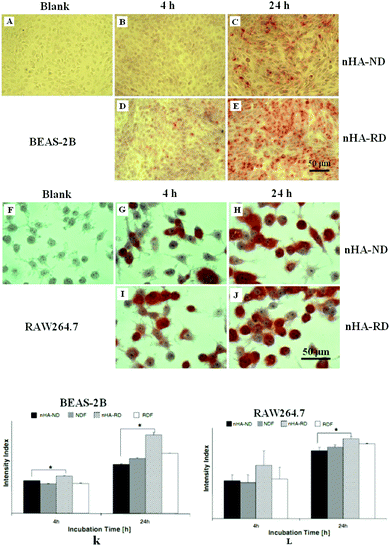 |
| Fig. 8 Images (A–E) are Alizarin red S stained BEAS-2B cells cultured in different conditions. Images (F–J) are Alizarin red S stained RAW264.7 cells cultured in different conditions. Graphs (K and L) are the quantifications of Alizarin red S staining of BEAS-2B (K) and RAW264.7 (I), cells were cultured in 100 μg mL−1 of nanocrystalline HA over 4 h and 24 h.52 | |
It was found that the HA needles and plates induced more cell death in BEAS-2B cultures compared to the HA spheres and rods. A necrosis-apoptosis assay using FITC Annexin V and propidium iodide (PI) staining revealed the loss of the majority of BEAS-2B cells by necrosis. No significant cell death was recorded in the RAW264.7 cells cultured with any of the shapes of the nanostructured HA. Correspondingly, no significant differences were observed in the TNF-α level for the RAW264.7 cells upon incubation with all shapes of nanostructured HA. In addition, the HA rods exhibited a higher degree of particle–cell association and internalization in both the BEAS-2B and RAW264.7 cells compared to the HA needles. These phenomena suggested that the shape of nanostructured HA significantly affects the cytotoxicity.
An in vivo model is the gold standard for cytotoxicity tests.166 Zhao et al. used zebrafish as an animal model to study the cytotoxicity of nanostructured HA due to their high degree of homology to the human genome and their comparable human tissue types.167 They evaluated the potential toxicity of different shapes of nanostructured HA (rods and needles) to mammalian cells. According to their results, the needle-shaped HA was found to inhibit the metabolic activity of catfish B cells (3B11) and T cells (28 s.3), especially at the highest test concentration of 300 μg mL−1. The nanocrystalline HA–cell interaction was time-dependent and thereby revealed that the cytotoxicity is likely to be caused by slow exocytosis. Moreover, both needle-shaped and rod-shaped HA delayed zebrafish embryos hatching. Approximately 75% of the embryos had malformed spines when exposed to the HA needles at 120 hpf, which could indicate a sub-lethal toxicity effect. Therefore, it can be concluded that the in vivo biological effects of HA nanocrystals are also shape-dependent.
As mentioned above, although it was confirmed that nanostructured CaP bears some potential cytotoxicity, the cytotoxicity is dependent on the type of exposed cells. Therefore, some researchers have tried to use nanosized CaP to treat some cancer-related diseases.168,169 Recent studies have shown that HA nanocrystals inhibited the growth and proliferation of several types of cancer cells, while having minimal side effects on normal cells. Li et al. found that when ultrafine HA particles were added to the cellular culture medium, the growth of human gastric cancer cells (MGC-803) was inhibited.169 They observed disorganization of microtubules and disruption of the stress fiber in cells treated with HA. It is believed that the ultrafine HA particles changed the morphology of the MGC-803 cells, which eventually affected the cytoskeletons of these cells. The effect of HA nanoparticles on the human hepatoma cell line (BEL-7402) was investigated by Liu et al., who found that it is dose-dependent. The nanoparticles inhibited cell growth, and the half effective inhibitory concentration (IC50) was 29.3 mg L−1.170 Fu et al. also found that spherical HA nanoparticles express an effective inhibition to the proliferation of osteosarcoma cells (U2OS).171,172 The inhibition rate of the HA nanoparticles to U2OS was 73.4 ± 3.1% after a 7 day culture in 250 μg mL−1 of the HA nanoparticle suspension. Fu et al. used spherical HA nanoparticles (20–40 nm) and measured the biological responses of normal cells (mouse fibroblasts, L929) and cancer cells (osteosarcoma cells, U2OS).172 The cell proliferation experiments showed that the spherical HA nanoparticles promoted the proliferation of L929 cells, while inhibiting the growth of U2OS cells, suggesting that it is biocompatible with L929 cells, but not with U2OS cells. The inhibition rate of the spherical HA nanoparticles increased with time and concentration. The half effective inhibitory concentration (IC50) of the nanoparticles to U2OS was 50.8 μg mL−1 at 72 h. These results indicate that nanocrystalline HA particles have potential applications to repair bone defects resulting from tumor resection, while having little adverse effect.
Besides cytotoxicity, the inflammatory response caused by nanostructured CaP is also an important issue for the application of nanostructured CaP. Zhao et al. reported that the inflammatory response of hydroxyapatite nanoparticles is shape dependent. Among the nanosized HAs with various morphologies, such as plate, sphere, rod and needle shaped, the HA needles can cause a serious inflammatory reaction and induce cells to overproduce inflammatory cytokines such as TNF-α and IL-6.159–161 It has been previously reported that TNF-α and IL-6 are important inflammatory cytokines expressed by most cell types.164,165 From their results, it has been found that BEAS-2B cell necrosis from exposure to HA nanoparticles was strongly correlated with the expression of IL-6 cytokines. IL-6 is a well-known responder to cellular injury, which regulates the acute phase of the inflammatory response. Although the CaP nanoparticles may cause an inflammatory response, they do not result in significant cell death. Zhao observed that after treatment with 100–300 μg mL−1 nanosized HA, RAW264.7 cells released a significant amount of TNF-α, but significant cell death did result. It is believed that inflammasomes serve as an early warning system to the innate immune system and the inflammatory response caused by nanostructured CaP does not represent a serious adverse effect.
In summary, although CaP nanoparticles may result in an inflammatory response, such an adverse effect does not affect its application and the cytotoxicity induced by nanosized CaP, on the other hand, is also associated with the type of exposed cells. The dosage, size, morphology and structure of the CaP nanoparticles play key roles in the cellular response and subsequent behaviours. The potential toxicity mechanisms of the nanoparticle–cell and nanoparticle–tissue interactions are likely to be drastically different and any clinical applications need careful investigation.
5 Final remarks
The development of nanotechnology facilitates the application of nanosized CaP. The potential applications of nanostructured CaP biomaterials in both hard tissues and soft tissues, including bone, cartilage, blood vessels and nerve tissues repair or regeneration, were highlighted in this review. Some specific biological effects and influences of nanosized CaP were extensively summarized. Some special phenomena, such as the preservation or stimulation of cellular survival, morphology and migration, up-regulation of angiogenic signaling pathways, promotion of some special growth factor expressions, etc., were also discussed. These phenomena are believed to be of benefit for the rapid repair of tissue injuries.
However, the mechanisms by which cells recognize the substrate size and their subsequent biological responses are still unclear. Material properties including the morphology, size, shape, as well as the distribution and concentration of the nanosized CaP affect the cellular behaviours and subsequent biological responses. However, understanding the interactions between nanoparticles and living cells is still a great challenge. Much work needs to be done in terms of the cells and their different behaviours with regards to their interactions with CaP nanostructures.
While promoting bone repair and accelerating tissue regeneration, nanosized CaP also displays a considerable ability to induce apoptosis to some types of cancer cells, while having few side effects on normal cells. This biological function of nanosized CaP has a large potential for repairing bone defects resulting from tumour resection. Nevertheless, the biosecurity of nanosized CaP is still a problem. Nanoparticles are likely to cause some inflammatory responses and can penetrate into biological organisms easily, for example they are likely to enter the circulatory system via blood vessels or lungs. Any clinical applications of nanosized CaP need be carefully investigated and fully evaluated before implementation.
Acknowledgements
The authors wish to express their appreciation to Professor Wei Li and Mr. Russell Borduin from the University of Texas at Austin for their helpful suggestions and criticism. This work was supported by The National High-tech R&D Program (2011AA030105), The National Natural Science Foundation of China (Grant 31170928) and The Youth Scholar Fund (20090181120078).
Notes and references
- L. L. Hench and J. M. Polak, Third-generation biomedical materials, Science, 2002, 295, 1014 CrossRef CAS.
- A. Veis, Materials science. A window on biomineralization, Science, 2005, 307, 1419 CrossRef CAS.
- Y. Cai, Y. Liu, W. Yan, Q. Hu, J. Tao, M. Zhang, Z. Shi and R. Tang, Role of hydroxyapatite nanoparticle size in bone cell proliferation, J. Mater. Chem., 2007, 17, 3780 RSC.
- P. N. Kumta, C. Sfeir, D. H. Lee, D. Olton and D. Choi, Nanostructured calcium phosphates for biomedical applications: novel synthesis and characterization, Acta Biomater., 2005, 1, 65 CrossRef.
- F. Qiang, M. N. Rahaman, Z. Nai, H. Wenhai, W. Deping, Z. Liying and L. Haifeng,
In vitro study on different cell response to spherical hydroxyapatite nanoparticles, J. Biomater. Appl., 2008, 23, 37 CrossRef.
- S. H. Chu, D. F. Feng, Y. B. Ma and Z. Q. Li, Hydroxyapatite nanoparticles inhibit the growth of human glioma cells in vitro and in vivo, Int. J. Nanomed., 2012, 7, 3659 CrossRef CAS.
- X. Zhao, B. C. Heng, S. Xiong, J. Guo, T. T. Tan, F. Y. Boey, K. W. Ng and J. S. Loo,
In vitro assessment of cellular responses to rod-shaped hydroxyapatite nanoparticles of varying lengths and surface areas, Nanotoxicology, 2011, 5, 182 CrossRef CAS.
- G. Xie, J. Sun, G. Zhong, C. Liu and J. Wei, Hydroxyapatite nanoparticles as a controlled-release carrier of BMP-2: absorption and release kinetics in vitro, J. Mater. Sci.: Mater. Med., 2010, 21, 1875 CrossRef CAS.
- K. Kandori, T. Kuroda, S. Togashi and E. Katayama, Preparation of calcium hydroxyapatite nanoparticles using microreactor and their characteristics of protein adsorption, J. Phys. Chem. B, 2011, 115, 653 CrossRef CAS.
- S. V. Dorozhkin, Nanosized and nanocrystalline calcium orthophosphates, Acta Biomater., 2010, 6, 715 CrossRef CAS.
- M. Nelson, G. Balasundaram and T. J. Webster, Increased osteoblast adhesion on nanoparticulate crystalline hydroxyapatite functionalized with krsr, Int. J. Nanomed., 2006, 1, 339 CAS.
- S. W. Choi, Y. Zhang, S. Thomopoulos and Y. Xia,
In vitro mineralization by preosteoblasts in poly(DL-lactide-co-glycolide) inverse opal scaffolds reinforced with hydroxyapatite nanoparticles, Langmuir, 2010, 26, 12126 CrossRef CAS.
- M. Nagano, T. Nakamura, T. Kokubo, M. Tanahashi and M. Ogawa, Differences of bone bonding ability and degradation behaviour in vivo between amorphous calcium phosphate and highly crystalline hydroxyapatite coating, Biomaterials, 1996, 17, 1771 CrossRef CAS.
- P. T. F. Frayssinet, N. Rouquet, P. Conte, C. Delga and G. Bonel, Comparative biological properties of HA plasma-sprayed coatings having different crystallinities, J. Mater. Sci.: Mater. Med., 1994, 5, 11 CrossRef CAS.
- M. Arnold, E. A. Cavalcanti-Adam, R. Glass, J. Blummel, W. Eck, M. Kantlehner, H. Kessler and J. P. Spatz, Activation of integrin function by nanopatterned adhesive interfaces, ChemPhysChem, 2004, 5, 383 CrossRef CAS.
- G. Balasundaram, M. Sato and T. J. Webster, Using hydroxyapatite nanoparticles and decreased crystallinity to promote osteoblast adhesion similar to functionalizing with RGD, Biomaterials, 2006, 27, 2798 CrossRef CAS.
- S. Ozkan, D. M. Kalyon and X. Yu, Functionally graded beta-TCP/PCL nanocomposite scaffolds: in vitro evaluation with human fetal osteoblast cells for bone tissue engineering, J. Biomed. Mater. Res., Part A, 2010, 92, 100 Search PubMed.
- S. K. Misra, T. Ansari, D. Mohn, S. P. Valappil, T. J. Brunner, W. J. Stark, I. Roy, J. C. Knowles, P. D. Sibbons, E. V. Jones, A. R. Boccaccini and V. Salih, Effect of nanoparticulate bioactive glass particles on bioactivity and cytocompatibility of poly(3-hydroxybutyrate) composites, J. R. Soc. Interface, 2010, 7, 453 CrossRef CAS.
- S. Jeanne, S. Tricot-Doleux, P. Pellen-Mussi, F. Perez, H. Oudadesse and G. Cathelineau, Effect of hydroxyapatite and beta-tricalcium phosphate nanoparticles on promonocytic U937 cells, J. Biomed. Nanotechnol., 2010, 6, 158 CrossRef.
- A. K. Guha, S. Singh, R. Kumaresan, S. Nayar and A. Sinha, Mesenchymal cell response to nanosized biphasic calcium phosphate composites, Colloids Surf., B, 2009, 73, 146 CrossRef CAS.
- K. Tan, P. Cheang, I. A. Ho, P. Y. Lam and K. M. Hui, Nanosized bioceramic particles could function as efficient gene delivery vehicles with target specificity for the spleen, Gene Ther., 2007, 14, 828 CrossRef CAS.
- S. C. Loo, T. Moore, B. Banik and F. Alexis, Biomedical applications of hydroxyapatite nanoparticles, Curr. Pharm. Biotechnol., 2010, 11, 333 CAS.
- M. Kamitakahara, K. Kimura and K. Ioku, Synthesis of nanosized porous hydroxyapatite granules in hydrogel by electrophoresis, Colloids Surf., B, 2012, 97, 236 CrossRef CAS.
- R. A. Perez, G. Altankov, E. Jorge-Herrero and M. P. Ginebra, Micro- and nanostructured hydroxyapatite-collagen microcarriers for bone tissue-engineering applications, J. Tissue Eng. Regener. Med., 2013, 7, 353 CrossRef CAS.
- X. Zhu, H. Zhang, D. Li, H. Fan and X. Zhang, Study on the enhanced protein adsorption of microwave sintered hydroxyapatite nanoceramic particles: Role of microstructure, J. Biomed. Mater. Res., Part B, 2012, 100B, 516 CrossRef CAS.
- Y. Cai and R. Tang, Calcium phosphate nanoparticles in biomineralization and biomaterials, J. Mater. Chem., 2008, 18, 3775 RSC.
- M. J. Olszta, X. Cheng, S. S. Jee, R. Kumar, Y.-Y. Kim, M. J. Kaufman, E. P. Douglas and L. B. Gower, Bone structure and formation: a new perspective, Mater. Sci. Eng., R, 2007, 58, 77 CrossRef.
- R. Tang, L. Wang, C. A. Orme, T. Bonstein, P. J. Bush and G. H. Nancollas, Dissolution at the nanoscale: Self-preservation of biominerals, Angew. Chem., Int. Ed., 2004, 43, 2697 CrossRef CAS.
- T. Hassenkam, G. E. Fantner, J. A. Cutroni, J. C. Weaver, D. E. Morse and P. K. Hansma, High-resolution afm imaging of intact and fractured trabecular bone, Bone, 2004, 35, 4 CrossRef.
- L. Zhang and T. J. Webster, Nanotechnology and nanomaterials: Promises for improved tissue regeneration, Nano Today, 2009, 4, 66 CrossRef CAS.
- Z. Shi, X. Huang, Y. Cai, R. Tang and D. Yang, Size effect of hydroxyapatite nanoparticles on proliferation and apoptosis of osteoblast-like cells, Acta Biomater., 2009, 5, 338 CrossRef CAS.
- A. P. Alivisatos, Biomineralization. Naturally aligned nanocrystals, Science, 2000, 289, 736 CrossRef CAS.
- A. L. Boskey, Biomineralization: An overview, Connect. Tissue Res., 2003, 44(Suppl 1), 5 CAS.
- D. W. Kim, I. S. Cho, J. Y. Kim, H. L. Jang, G. S. Han, H. S. Ryu, H. Shin, H. S. Jung, H. Kim and K. S. Hong, Simple large-scale synthesis of hydroxyapatite nanoparticles: in situ observation of crystallization process, Langmuir, 2010, 26, 384 CrossRef CAS.
- T. Yuasa, Y. Miyamoto, K. Ishikawa, M. Takechi, Y. Momota, S. Tatehara and M. Nagayama, Effects of apatite cements on proliferation and differentiation of human osteoblasts in vitro, Biomaterials, 2004, 25, 1159 CrossRef CAS.
- L. Cerroni, R. Filocamo, M. Fabbri, C. Piconi, S. Caropreso and S. G. Condo, Growth of osteoblast-like cells on porous hydroxyapatite ceramics: An in vitro study, Biomol. Eng., 2002, 19, 119 CrossRef CAS.
- I. Zizak, P. Roschger, O. Paris, B. M. Misof, A. Berzlanovich, S. Bernstorff, H. Amenitsch, K. Klaushofer and P. Fratzl, Characteristics of mineral particles in the human bone/cartilage interface, J. Struct. Biol., 2003, 141, 208 CrossRef CAS.
- Y. Pan, D. Xiong and F. Gao, Viscoelastic behavior of nano-hydroxyapatite reinforced poly(vinyl alcohol) gel biocomposites as an articular cartilage, J. Mater. Sci.: Mater. Med., 2008, 19, 1963 CrossRef CAS.
- K. Yudoh, K. Shishido, H. Murayama, M. Yano and K. Matsubayashi, Water-soluble C60 fullerene prevents degeneration of articular cartilage in osteoarthritis via down-regulation of chondrocyte catabolic activity and inhibition of cartilage degeneration during disease development, Arthritis Rheum., 2007, 56, 3307 CrossRef CAS.
- E. Wernike, M. O. Montjovent, Y. Liu, D. Wismeijer, E. B. Hunziker, K. A. Siebenrock, W. Hofstetter and F. M. Klenke, VEGF incorporated into calcium phosphate ceramics promotes vascularisation and bone formation in vivo, Eur. Cell Mater., 2010, 19, 30 CAS.
- F. C. Cackowski, J. L. Anderson, K. D. Patrene, R. J. Choksi, S. D. Shapiro, J. J. Windle, H. C. Blair and G. D. Roodman, Osteoclasts are important for bone angiogenesis, Blood, 2010, 115, 140 CrossRef CAS.
- S. Pezzatini, L. Morbidelli, R. Solito, E. Paccagnini, E. Boanini, A. Bigi and M. Ziche, Nanostructured HA crystals up-regulate FGF-2 expression and activity in microvascular endothelium promoting angiogenesis, Bone, 2007, 41, 523 CrossRef CAS.
- X. Tang, Q. Zhang, S. Shi, Y. Yen, X. Li, Y. Zhang, K. Zhou and A. D. Le, Bisphosphonates suppress insulin-like growth factor 1-induced angiogenesis via the HIF-1alpha/VEGF signaling pathways in human breast cancer cells, Int. J. Cancer, 2010, 126, 90 CrossRef CAS.
- M. Liu, G. Zhou, W. Song, P. Li, H. Liu, X. Niu and Y. Fan, Effect of nano-hydroxyapatite on the axonal guidance growth of rat cortical neurons, Nanoscale, 2012, 4, 3201 RSC.
- T. A. Fulga, I. Elson-Schwab, V. Khurana, M. L. Steinhilb, T. L. Spires, B. T. Hyman and M. B. Feany, Abnormal bundling and accumulation of F-actin mediates tau-induced neuronal degeneration in vivo, Nat. Cell Biol., 2007, 9, 139 CrossRef CAS.
- X. Liu, D. Fan, J. Zhang, J. Zheng and T. Ma, Comparison of distributions of muscle injection and intravenous administration of human mesenchymal stem cells in denervated muscles of the sciatic nerve injured rats, Beijing Da Xue Xue Bao, 2008, 40, 185 Search PubMed.
- Y. J. Han, S. C. Loo, J. Lee and J. Ma, Investigation of the bioactivity and biocompatibility of different glass interfaces with hydroxyapatite, fluorohydroxyapatite and 58 s bioactive glass, BioFactors, 2007, 30, 205 CrossRef CAS.
- Y. Yuan, C. Liu, J. Qian, J. Wang and Y. Zhang, Size-mediated cytotoxicity and apoptosis of hydroxyapatite nanoparticles in human hepatoma HEPG2 cells, Biomaterials, 2010, 31, 730 CrossRef CAS.
- M. Motskin, D. M. Wright, K. Muller, N. Kyle, T. G. Gard, A. E. Porter and J. N. Skepper, Hydroxyapatite nano and microparticles: correlation of particle properties with cytotoxicity and biostability, Biomaterials, 2009, 30, 3307 CrossRef CAS.
- C. Albrecht, A. M. Scherbart, D. van Berlo, C. M. Braunbarth, R. P. Schins and J. Scheel, Evaluation of cytotoxic effects and oxidative stress with hydroxyapatite dispersions of different physicochemical properties in rat NR8383 cells and primary macrophages, Toxicol. in Vitro, 2009, 23, 520 CrossRef CAS.
- J. Huang, S. M. Best, W. Bonfield, R. A. Brooks, N. Rushton, S. N. Jayasinghe and M. J. Edirisinghe,
In vitro assessment of the biological response to nano-sized hydroxyapatite, J. Mater. Sci.: Mater. Med., 2004, 15, 441 CrossRef CAS.
- X. Zhao, S. Ng, B. C. Heng, J. Guo, L. Ma, T. T. Tan, K. W. Ng and S. C. Loo, Cytotoxicity of hydroxyapatite nanoparticles is shape and cell dependent, Arch. Toxicol., 2013, 87, 1037 CrossRef CAS.
- L. Zhang, J. Rodriguez, J. Raez, A. J. Myles, H. Fenniri and T. J. Webster, Biologically inspired rosette nanotubes and nanocrystalline hydroxyapatite hydrogel nanocomposites as improved bone substitutes, Nanotechnology, 2009, 20, 175101 CrossRef.
- S. Sanchez-Salcedo, I. Izquierdo-Barba, D. Arcos and M. Vallet-Regi,
In vitro evaluation of potential calcium phosphate scaffolds for tissue engineering, Tissue Eng., 2006, 12, 279 CrossRef CAS.
- F. Chen, Y. Zhu, J. Wu, P. Huang and D. Cui, Nanostructured calcium phosphates: Preparation and their application in biomedicine, Nano Biomed. Eng., 2012, 4, 41 CrossRef CAS.
- M. Epple, K. Ganesan, R. Heumann, J. Klesing, A. Kovtun, S. Neumann and V. Sokolova, Application of calcium phosphate nanoparticles in biomedicine, J. Mater. Chem., 2010, 20, 18 RSC.
- B. Kateb, K. Chiu, K. L. Black, V. Yamamoto, B. Khalsa and J. Y. Ljubimova, Nanoplatforms for constructing new approaches to cancer treatment, imaging, and drug delivery: What should be the policy?, NeuroImage, 2011, 54(Suppl 1), S106 CrossRef.
- S. V. Dorozhkin, Bioceramics of calcium orthophosphates, Biomaterials, 2010, 31, 1465 CrossRef CAS.
- D. Williams, The relationship between biomaterials and nanotechnology, Biomaterials, 2008, 29, 1737 CrossRef CAS.
- P. Moriarty, Nanostructured materials, Rep. Prog. Phys., 2001, 64, 297 CrossRef CAS.
- T. J. Webster and E. S. Ahn, Nanostructured biomaterials for tissue engineering bone, Adv. Biochem. Eng. Biotechnol., 2007, 103, 275 CrossRef CAS.
- R. M. Streicher, M. Schmidt and S. Fiorito, Nanosurfaces and nanostructures for artificial orthopedic implants, Nanomedicine, 2007, 2, 861 CrossRef CAS.
-
E. Commission, Scientific committee on emerging and newly identified health risks (SCENIHR). Opinion on “the scientific aspects of the existing and proposed definitions relating to products of nanoscience and nanotechnologies”, Springer, Berlin, 2007 Search PubMed.
- S. C. Leeuwenburgh, J. A. Jansen and A. G. Mikos, Functionalization of oligo(poly(ethylene glycol)fumarate) hydrogels with finely dispersed calcium phosphate nanocrystals for bone-substituting purposes, J. Biomater. Sci. Polym. Ed., 2007, 18, 1547 CAS.
- J. E. Barralet, K. J. Lilley, L. M. Grover, D. F. Farrar, C. Ansell and U. Gbureck, Cements from nanocrystalline hydroxyapatite, J. Mater. Sci.: Mater. Med., 2004, 15, 407 CrossRef CAS.
- F. Barrere, M. M. Snel, C. A. van Blitterswijk, K. de Groot and P. Layrolle, Nano-scale study of the nucleation and growth of calcium phosphate coating on titanium implants, Biomaterials, 2004, 25, 2901 CrossRef CAS.
- J. S. Bow, S. C. Liou and S. Y. Chen, Structural characterization of room-temperature synthesized nano-sized beta-tricalcium phosphate, Biomaterials, 2004, 25, 3155 CrossRef CAS.
- M. C. Chang, C. C. Ko and W. H. Douglas, Preparation of hydroxyapatite-gelatin nanocomposite, Biomaterials, 2003, 24, 2853 CrossRef CAS.
- D. O. Costa, S. J. Dixon and A. S. Rizkalla, One- and three-dimensional growth of hydroxyapatite nanowires during sol–gel-hydrothermal synthesis, ACS Appl. Mater. Interfaces, 2012, 4, 1490 CAS.
- S. Hayakawa, Y. Li, K. Tsuru, A. Osaka, E. Fujii and K. Kawabata, Preparation of nanometer-scale rod array of hydroxyapatite crystal, Acta Biomater., 2009, 5, 2152 CrossRef CAS.
- F. Chen, Y. J. Zhu, K. W. Wang and K. L. Zhao, Surfactant-free solvothermal synthesis of hydroxyapatite nanowire/nanotube ordered arrays with biomimetic structures, CrystEngComm, 2011, 13, 1858 RSC.
- Y. Hong, H. Fan, B. Li, B. Guo, M. Liu and X. Zhang, Fabrication, biological effects, and medical applications of calcium phosphate nanoceramics, Mater. Sci. Eng., R, 2010, 70, 225 CrossRef.
- F. Chen, Y. Zhu, J. Wu, P. Huang and D. Cui, Nanostructured calcium phosphates: Preparation and their application in biomedicine, Nano Biomed. Eng., 2012, 4, 41 CrossRef CAS.
- C. Zhang, J. Yang, Z. Quan, P. Yang, C. Li, Z. Hou and J. Lin, Hydroxyapatite nano- and microcrystals with multiform morphologies controllable synthesis and luminescence properties, Cryst. Growth Des., 2009, 9, 2725 CAS.
- C. K. Chan, T. S. Kumar, S. Liao, R. Murugan, M. Ngiam and S. Ramakrishnan, Biomimetic nanocomposites for bone graft applications, Nanomedicine, 2006, 1, 177 CrossRef CAS.
- E. Kon, M. Delcogliano, G. Filardo, D. Pressato, M. Busacca, B. Grigolo, G. Desando and M. Marcacci, A novel nano-composite multi-layered biomaterial for treatment of osteochondral lesions: Technique note and an early stability pilot clinical trial, Injury, 2010, 41, 693 CrossRef CAS.
- R. Detsch, D. Hagmeyer, M. Neumann, S. Schaefer, A. Vortkamp, M. Wuelling, G. Zieglera and M. Epple, The resorption of nanocrystalline calcium phosphates by osteoclast-like cells, Acta Biomater., 2010, 6, 3223 CrossRef CAS.
- R. Z. LeGeros, Biodegradation and bioresorption of calcium phosphate ceramics, Clin. Mater., 1993, 14, 65 CrossRef CAS.
- H. Zhou and J. Lee, Nanoscale hydroxyapatite particles for bone tissue engineering, Acta Biomater., 2011, 7, 2769 CrossRef CAS.
- A. Kasaj, B. Willershausen, C. Reichert, B. Rohrig, R. Smeets and M. Schmidt, Ability of nanocrystalline hydroxyapatite paste to promote human periodontal ligament cell proliferation, J. Oral Sci., 2008, 50, 279 CrossRef CAS.
- S. Bose, S. Dasgupta, S. Tarafder and A. Bandyopadhyay, Microwave-processed nanocrystalline hydroxyapatite: simultaneous enhancement of mechanical and biological properties, Acta Biomater., 2010, 6, 3782 CrossRef CAS.
- A. Kasaj, B. Willershausen, R. Junker, S. I. Stratul and M. Schmidt, Human periodontal ligament fibroblasts stimulated by nanocrystalline hydroxyapatite paste or enamel matrix derivative. An in vitro assessment of pdl attachment, migration, and proliferation, Clin. Oral Invest., 2012, 16, 745 CrossRef.
- O. Kilian, V. Alt, C. Heiss, T. Jonuleit, E. Dingeldein, I. Flesch, U. Fidorra, S. Wenisch and R. Schnettler, New blood vessel formation and expression of vegf receptors after implantation of platelet growth factor-enriched biodegradable nanocrystalline hydroxyapatite, Growth Factors, 2005, 23, 125 CrossRef CAS.
- M. Rogel, H. Qiu and G. Ameer, The role of nanocomposites in bone regeneration, J. Mater. Chem., 2008, 18, 4233 RSC.
- L. Wang, G. H. Nancollas, Z. J. Henneman, E. Klein and S. Weiner, Nanosized particles in bone and dissolution insensitivity of bone mineral, Biointerphases, 2006, 1, 106 CrossRef CAS.
- B. Adele, Bone mineral crystal size, Osteoporos Int., 2003, 14(Suppl 5), S16–S20 Search PubMed , discussion S-1.
- M. P. Ginebra, F. C. M. Driessens and J. A. Planell, Effect of the particle size on the micro and nanostructural features of a calcium phosphate cement: a kinetic analysis, Biomaterials, 2004, 25, 3453 CrossRef CAS.
- E. Engel, S. D. Valle, C. Aparicio, G. Altankov, L. Sain, J. A. Planell and M. P. Ginebra, Discerning the Role of Topography and Ion Exchange in Cell Response of Bioactive Tissue Engineering Scaffolds, Tissue Eng. A, 2008, 14, 1341 CrossRef CAS.
- K. Lin, J. Chang, J. Lu, W. Wu and Y. Zeng, Properties of beta-Ca3(PO4)2 bioceramics prepared using nano-size powders, Ceram. Int., 2007, 33, 979 CrossRef CAS.
- G. L. Yang, F. M. He, J. A. Hu, X. X. Wang and S. F. Zhao, Effects of biomimetically and electrochemically deposited nano-hydroxyapatite coatings on osseointegration of porous titanium implants, Oral Surg., Oral Med., Oral Pathol., Oral Radiol. Endodontol., 2009, 107, 782 CrossRef.
- H. Autefage, F. Briand-Mesange, S. Cazalbou, C. Drouet, D. Fourmy, S. Goncalves, J. P. Salles, C. Combes, P. Swider and C. Rey, Adsorption and release of BMP-2 on nanocrystalline apatite-coated and uncoated hydroxyapatite/beta-tricalcium phosphate porous ceramics, J. Biomed. Mater. Res., Part B, 2009, 91, 706 CrossRef.
- S. M. Shibli and A. C. Jayalekshmi, A novel nano hydroxyapatite-incorporated ni-p coating as an effective inter layer for biological applications, J. Mater. Sci.: Mater. Med., 2009, 20, 711 CrossRef CAS.
- Y. P. Lu, Y. M. Chen, S. T. Li and J. H. Wang, Surface nanocrystallization of hydroxyapatite coating, Acta Biomater., 2008, 4, 1865 CrossRef CAS.
- S. I. Roohani-Esfahani, S. Nouri-Khorasani, Z. F. Lu, R. C. Appleyard and H. Zreiqat, Effects of bioactive glass nanoparticles on the mechanical and biological behavior of composite coated scaffolds, Acta Biomater., 2011, 7, 1307 CrossRef CAS.
- Y. Zhao, Y. Zhang, Y. Zhao, S. Hou and P. K. Chu, Synthesis and cellular biocompatibility of two nanophase hydroxyapatite with different Ca/P ratio, J. Nanosci. Nanotechnol., 2011, 11, 11069 CrossRef CAS.
- Q. Hu, Z. Tan, Y. Liu, J. Tao, M. Zhang, H. Pan, X. Xu and R. Tang, Effect of crystallinity of calcium phosphate nanoparticles on adhesion, proliferation, and differentiation of bone marrow mesenchymal stem cells, J. Mater. Chem., 2007, 17, 4690 RSC.
- X. Liu, M. Zhao, J. Lu, J. Ma, J. Wei and S. Wei, Cell responses to two kinds of nanohydroxyapatite with different sizes and crystallinities, Int. J. Nanomed., 2012, 7, 1239 CrossRef CAS.
- Y. Huang, G. Zhou, L. Zheng, H. Liu, X. Niu and Y. Fan, Micro-/nano-sized hydroxyapatite directs differentiation of rat bone marrow derived mesenchymal stem cells towards an osteoblast lineage, Nanoscale, 2012, 4, 2484 RSC.
- J. L. Xu, K. A. Khor, J. J. Sui, J. H. Zhang and W. N. Chen, Protein expression profiles in osteoblasts in response to differentially shaped hydroxyapatite nanoparticles, Biomaterials, 2009, 30, 5385 CrossRef CAS.
- M. J. Phillips, J. A. Darr, Z. B. Luklinska and I. Rehman, Synthesis and characterization of nano-biomaterials with potential osteological applications, J. Mater. Sci.: Mater. Med., 2003, 14, 875 CrossRef CAS.
- S. A. Catledge, M. D. Fries, Y. K. Vohra, W. R. Lacefield, J. E. Lemons, S. Woodard and R. Venugopalan, Nanostructured ceramics for biomedical implants, J. Nanosci. Nanotechnol., 2002, 2, 293 CrossRef CAS.
- M. M. Stevens and J. H. George, Exploring and engineering the cell surface interface, Science, 2005, 310, 1135 CrossRef CAS.
- A. K. Gupta, M. Gupta, S. J. Yarwood and A. S. Curtis, Effect of cellular uptake of gelatin nanoparticles on adhesion, morphology and cytoskeleton organisation of human fibroblasts, J. Controlled Release, 2004, 95, 197 CrossRef CAS.
- M. Bongio, J. J. van den Beucken, M. R. Nejadnik, S. C. Leeuwenburgh, L. A. Kinard, F. K. Kasper, A. G. Mikos and J. A. Jansen, Biomimetic modification of synthetic hydrogels by incorporation of adhesive peptides and calcium phosphate nanoparticles: in vitro evaluation of cell behavior, Eur. Cell Mater., 2011, 22, 359 CAS.
- N. Aboudzadeh, M. Imani, M. A. Shokrgozar, A. Khavandi, J. Javadpour, Y. Shafieyan and M. Farokhi, Fabrication and characterization of poly(D,L-lactide-co-glycolide)/hydroxyapatite nanocomposite scaffolds for bone tissue regeneration, J. Biomed. Mater. Res., Part A, 2010, 94, 137 CrossRef.
- Y. Cui, Y. Liu, Y. Cui, X. Jing, P. Zhang and X. Chen, The nanocomposite scaffold of poly(lactide-co-glycolide) and hydroxyapatite surface-grafted with L-lactic acid oligomer for bone repair, Acta Biomater., 2009, 5, 2680 CrossRef CAS.
- S. Zeng, S. Fu, G. Guo, H. Liang, Z. Qian, X. Tang and F. Luo, Preparation and characterization of nano-hydroxyapatite/poly(vinyl alcohol) composite membranes for guided bone regeneration, J. Biomed. Nanotechnol., 2011, 7, 549 CrossRef CAS.
- N. Ribeiro, S. R. Sousa and F. J. Monteiro, Influence of crystallite size of nanophased hydroxyapatite on fibronectin and osteonectin adsorption and on MC3T3-E1 osteoblast adhesion and morphology, J. Colloid Interface Sci., 2010, 351, 398 CrossRef CAS.
- S. Sethuraman, L. S. Nair, S. El-Amin, M. T. Nguyen, Y. E. Greish, J. D. Bender, P. W. Brown, H. R. Allcock and C. T. Laurencin, Novel low temperature setting nanocrystalline calcium phosphate cements for bone repair: osteoblast cellular response and gene expression studies, J. Biomed. Mater. Res., Part A, 2007, 82, 884 CrossRef.
- E. B. Hunziker, Articular cartilage repair: are the intrinsic biological constraints undermining this process insuperable?, Osteoarthritis Cartilage, 1999, 7, 15 CrossRef CAS.
- G. D. Smith, G. Knutsen and J. B. Richardson, A clinical review of cartilage repair techniques, J. Bone Joint Surg. Br., 2005, 87, 445 CAS.
- L. Hangody, G. Vasarhelyi, L. R. Hangody, Z. Sukosd, G. Tibay, L. Bartha and G. Bodo, Autologous osteochondral grafting—technique and long-term results, Injury, 2008, 39(Suppl 1), S32 CrossRef.
- J. Yang, G. Shi, J. Bei, S. Wang, Y. Cao, Q. Shang, G. Yang and W. Wang, Fabrication and surface modification of macroporous poly(L-lactic acid) and poly(L-lactic-co-glycolic acid) (70/30) cell scaffolds for human skin fibroblast cell culture, J. Biomed. Mater. Res., 2002, 62, 438 CrossRef CAS.
- M. Kobayashi, Y. S. Chang and M. Oka, A two year in vivo study of polyvinyl alcohol-hydrogel (PVA-H) artificial meniscus, Biomaterials, 2005, 26, 3243 CrossRef CAS.
- T. Noguchi, T. Yamamuro, M. Oka, P. Kumar, Y. Kotoura, S. Hyon and Y. Ikada, Poly(vinyl alcohol) hydrogel as an artificial articular cartilage: evaluation of biocompatibility, J. Appl. Biomater., 1991, 2, 101 CrossRef CAS.
- N. Tamai, A. Myoui, M. Hirao, T. Kaito, T. Ochi, J. Tanaka, K. Takaoka and H. Yoshikawa, A new biotechnology for articular cartilage repair: subchondral implantation of a composite of interconnected porous hydroxyapatite, synthetic polymer (PLA-PEG), and bone morphogenetic protein-2 (rhBMP-2), Osteoarthritis Cartilage, 2005, 13, 405 CrossRef.
- X. B. Jin, Y. S. Sun, K. Zhang, J. Wang, T. P. Shi, X. D. Ju and S. Q. Lou, Tissue engineered cartilage from htgf beta2 transduced human adipose derived stem cells seeded in PLGA/alginate compound in vitro and in vivo, J. Biomed. Mater. Res., Part A, 2008, 86, 1077 CrossRef.
- Y. Pan and D. Xiong, Study on compressive mechanical properties of nanohydroxyapatite reinforced poly(vinyl alcohol) gel composites as biomaterial, J. Mater. Sci.: Mater. Med., 2009, 20, 1291 CrossRef CAS.
- L. Cheng, S. M. Zhang, P. P. Chen, S. L. Huang, R. R. Cao, W. Zhou, J. Liu and Q. Luo, Fabrication and characterization of nano-hydroxyapatite/poly(D,L-lactide) composite porous scaffolds for human cartilage tissue engineering, Key Eng. Mater., 2006, 18, 943 CrossRef.
- D. Xue, Q. Zheng, C. Zong, Q. Li, H. Li, S. Qian, B. Zhang, L. Yu and Z. Pan, Osteochondral repair using porous poly(lactide-co-glycolide)/nano-hydroxyapatite hybrid scaffolds with undifferentiated mesenchymal stem cells in a rat model, J. Biomed. Mater. Res., Part A, 2010, 94, 259 CrossRef.
- S. C. Rizzi, D. J. Heath, A. G. Coombes, N. Bock, M. Textor and S. Downes, Biodegradable polymer/hydroxyapatite composites: Surface analysis and initial attachment of human osteoblasts, J. Biomed. Mater. Res., 2001, 55, 475 CrossRef CAS.
- R. J. Covert, R. D. Ott and D. N. Ku, Friction characteristics of a potential articular cartilage biomaterial, Wear, 2003, 255, 1064 CrossRef CAS.
- Y. S. D. Pan, S. Xiong and R. Y. Ma, A study on the friction properties of poly(vinyl alcohol) hydrogel as articular cartilage against titanium alloy, Wear, 2007, 262, 1021 CrossRef CAS.
- Y. Pan and D. Xiong, Stress relaxation behavior of nano-hydroxyapatite reinforced poly(vinyl alcohol) gel composites as biomaterial, J. Mater. Sci., 2010, 45, 5495 CrossRef CAS.
- N. Tudorachi and A. P. Chiriac, Poly(vinyl alcohol-co-lactic acid)/hydroxyapatite composites: synthesis and characterization, J. Polym. Environ., 2011, 19, 546 CrossRef CAS.
- Y. Pan, D. Xiong and X. Chen, Mechanical properties of nanohydroxyapatite reinforced poly(vinyl alcohol) gel composites as biomaterial, J. Mater. Sci., 2007, 42, 5129 CrossRef CAS.
- M. W. Laschke, K. Witt, T. Pohlemann and M. D. Menger, Injectable nanocrystalline hydroxyapatite paste for bone substitution: In vivo analysis of biocompatibility and vascularization, J. Biomed. Mater. Res., Part B, 2007, 82, 494 CrossRef.
- S. Pezzatini, R. Solito, L. Morbidelli, S. Lamponi, E. Boanini, A. Bigi and M. Ziche, The effect of hydroxyapatite nanocrystals on microvascular endothelial cell viability and functions, J. Biomed. Mater. Res., Part A, 2006, 76, 656 CrossRef.
- J. Harper and M. Klagsbrun, Cartilage to bone—angiogenesis leads the way, Nat. Med., 1999, 5, 617 CrossRef CAS.
- H. L. Guenther, H. Fleisch and N. Sorgente, Endothelial cells in culture synthesize a potent bone cell active mitogen, Endocrinology, 1986, 119, 193 CrossRef CAS.
- M. Samee, S. Kasugai, H. Kondo, K. Ohya, H. Shimokawa and S. Kuroda, Bone morphogenetic protein-2 (BMP-2) and vascular endothelial growth factor (VEGF) transfection to human periosteal cells enhances osteoblast differentiation and bone formation, J. Pharmacol. Sci., 2008, 108, 18 CrossRef CAS.
- C. N. Cornell and J. M. Lane, Current understanding of osteoconduction in bone regeneration, Clin. Orthop. Relat. Res., 1998, 355(Suppl), S267 Search PubMed.
- J. Street, M. Bao, L. deGuzman, S. Bunting, F. V. Peale Jr., N. Ferrara, H. Steinmetz, J. Hoeffel, J. L. Cleland, A. Daugherty, N. van Bruggen, H. P. Redmond, R. A. Carano and E. H. Filvaroff, Vascular endothelial growth factor stimulates bone repair by promoting angiogenesis and bone turnover, Proc. Natl. Acad. Sci. U. S. A., 2002, 99, 9656 CrossRef CAS.
- W. Suchanec and M. Yoshimura, Processing and properties
of hydroxyapatite-based biomaterials for use as hard tissue replacement implants, J. Mater. Res., 1998, 13, 94 CrossRef.
- S. Donnini and M. Ziche, Constitutive and inducible nitric oxide synthase: Role in angiogenesis, Antioxid. Redox Signaling, 2002, 4, 817 CrossRef CAS.
- L. Morbidelli, S. Donnini and M. Ziche, Role of nitric oxide in the modulation of angiogenesis, Curr. Pharm. Des., 2003, 9, 521 CrossRef CAS.
- M. Ziche, L. Morbidelli, R. Choudhuri, H. T. Zhang, S. Donnini, H. J. Granger and R. Bicknell, Nitric oxide synthase lies downstream from vascular endothelial growth factor-induced but not basic fibroblast growth factor-induced angiogenesis, J. Clin. Invest., 1997, 99, 2625 CrossRef CAS.
- S. Cantara, S. Donnini, L. Morbidelli, A. Giachetti, R. Schulz, M. Memo and M. Ziche, Physiological levels of amyloid peptides stimulate the angiogenic response through FGF-2, FASEB J., 2004, 18, 1943 CAS.
- H. F. Heijnen, S. Waaijenborg, J. D. Crapo, R. P. Bowler, J. W. Akkerman and J. W. Slot, Colocalization of enos and the catalytic subunit of PKA in endothelial cell junctions: A clue for regulated no production, J. Histochem. Cytochem., 2004, 52, 1277 CAS.
- M. Renko, N. Quarto, T. Morimoto and D. B. Rifkin, Nuclear and cytoplasmic localization of different basic fibroblast growth factor species, J. Cell. Physiol., 1990, 144, 108 CrossRef CAS.
- J. E. Greer, M. J. McGinn and J. T. Povlishock, Diffuse traumatic axonal injury in the mouse induces atrophy, c-jun activation, and axonal outgrowth in the axotomized neuronal population, J. Neurosci., 2011, 31, 5089 CrossRef CAS.
- D. H. Smith, D. F. Meaney and W. H. Shull, Diffuse axonal injury in head trauma, J. Head Trauma Rehabil., 2003, 18, 307 CrossRef.
- K. D. Barron, M. P. Dentinger, L. R. Nelson and J. E. Mincy, Ultrastructure of axonal reaction in red nucleus of cat, J. Neuropathol. Exp. Neurol., 1975, 34, 222 CrossRef CAS.
- V. Pernet, S. Joly, D. Dalkara, O. Schwarz, F. Christ, D. Schaffer, J. G. Flannery and M. E. Schwab, Neuronal nogo-a upregulation does not contribute to er stress-associated apoptosis but participates in the regenerative response in the axotomized adult retina, Cell Death Differ., 2012, 19, 1096 CrossRef CAS.
- P. G. Richardson, M. Delforge, M. Beksac, P. Wen, J. L. Jongen and O. Sezer, Management of treatment-emergent peripheral neuropathy in multiple myeloma, Leukemia, 2012, 26, 595 CrossRef CAS.
- X. R. Xia, N. A. Monteiro-Riviere and J. E. Riviere, An index for characterization of nanomaterials in biological systems, Nat. Nanotechnol., 2010, 5, 671 CrossRef CAS.
- P. Galvan-Garcia, E. W. Keefer, F. Yang, M. Zhang, S. Fang, A. A. Zakhidov, R. H. Baughman and M. I. Romero, Robust cell migration and neuronal growth on pristine carbon nanotube sheets and yarns, J. Biomater. Sci., Polym. Ed., 2007, 18, 1245 CrossRef CAS.
- G. Z. Jin, M. Kim, U. S. Shin and H. W. Kim, Neurite outgrowth of dorsal root ganglia neurons is enhanced on aligned nanofibrous biopolymer scaffold with carbon nanotube coating, Neurosci. Lett., 2011, 501, 10 CrossRef CAS.
- D. C. Merz, H. Zheng, M. T. Killeen, A. Krizus and J. G. Culotti, Multiple signaling mechanisms of the Unc-6/netrin receptors Unc-5 and Unc-40/dcc in vivo, Genetics, 2001, 158, 1071 CAS.
- K. Keleman and B. J. Dickson, Short- and long-range repulsion by the Drosophila Unc5 netrin receptor, Neuron, 2001, 32, 605 CrossRef CAS.
- H. S. Alghamdi, B. AJAvO, R. Bosco, J. J. van den Beucken, A. A. Aldosari, S. Anil and J. A. Jansen, Biological response to titanium implants coated with nanocrystals calcium phosphate or type 1 collagen in a dog model, Clin. Oral Implants Res., 2013, 24, 475 CrossRef.
- C. Schouten, G. J. Meijer, J. J. van den Beucken, S. C. Leeuwenburgh, L. T. de Jonge, J. G. Wolke, P. H. Spauwen and J. A. Jansen,
In vivo bone response and mechanical evaluation of electrosprayed cap nanoparticle coatings using the iliac crest of goats as an implantation model, Acta Biomater., 2010, 6, 2227 CrossRef CAS.
- G. Oberdorster, E. Oberdorster and J. Oberdorster, Nanotoxicology: an emerging discipline evolving from studies of ultrafine particles, Environ. Health Perspect., 2005, 113, 823 CrossRef CAS.
- S. K. Sohaebuddin, P. T. Thevenot, D. Baker, J. W. Eaton and L. Tang, Nanomaterial cytotoxicity is composition, size, and cell type dependent, Part. Fibre Toxicol., 2010, 7, 22 CrossRef.
- W. H. Suh, K. S. Suslick, G. D. Stucky and Y. H. Suh, Nanotechnology, nanotoxicology, and neuroscience, Prog. Neurobiol., 2009, 87, 133 CrossRef CAS.
- B. Li, B. Guo, H. Fan and X. Zhang, Preparation of nano-hydroxyapatite particles with different morphology and their response to highly malignant melanoma cell in vitro, Appl. Surf. Sci., 2008, 255, 357 CrossRef CAS.
- A. Nan, X. Bai, S. J. Son, S. B. Lee and H. Ghandehari, Cellular uptake and cytotoxicity of silica nanotubes, Nano Lett., 2008, 8, 2150 CrossRef CAS.
- B. K. Gaiser, T. F. Fernandes, M. Jepson, J. R. Lead, C. R. Tyler and V. Stone, Assessing exposure, uptake and toxicity of silver and cerium dioxide nanoparticles from contaminated environments, Environ. Health, 2009, 8(Suppl 1), S2 CrossRef.
- P. Laquerriere, A. Grandjean-Laquerriere, E. Jallot, G. Balossier, P. Frayssinet and M. Guenounou, Importance of hydroxyapatite particles characteristics on cytokines production by human monocytes in vitro, Biomaterials, 2003, 24, 2739 CrossRef CAS.
- A. Grandjean-Laquerriere, P. Laquerriere, M. Guenounou, D. Laurent-Maquin and T. M. Phillips, Importance of the surface area ratio on cytokines production by human monocytes in vitro induced by various hydroxyapatite particles, Biomaterials, 2005, 26, 2361 CrossRef CAS.
- A. Grandjean-Laquerriere, P. Laquerriere, D. Laurent-Maquin, M. Guenounou and T. M. Phillips, The effect of the physical characteristics of hydroxyapatite particles on human monocytes IL-18 production in vitro, Biomaterials, 2004, 25, 5921 CrossRef CAS.
- T. Blake, V. Castranova, D. Schwegler-Berry, P. Baron, G. J. Deye, C. Li and W. Jones, Effect of fiber length on glass microfiber cytotoxicity, J. Toxicol. Environ. Health, Part A, 1998, 54, 243 CrossRef CAS.
- G. D. Guthrie Jr., Mineral properties and their contributions to particle toxicity, Environ. Health Perspect., 1997, 105(Suppl 5), 1003 CrossRef.
- T. Xia, M. Kovochich, J. Brant, M. Hotze, J. Sempf, T. Oberley, C. Sioutas, J. I. Yeh, M. R. Wiesner and A. E. Nel, Comparison of the abilities of ambient and manufactured nanoparticles to induce cellular toxicity according to an oxidative stress paradigm, Nano Lett., 2006, 6, 1794 CrossRef CAS.
- S. Syed, A. Zubair and M. Frieri, Immune response to nanomaterials: Implications for medicine and literature review, Curr. Allergy Asthma Rep., 2012, 13, 50 CrossRef.
- L. I. Zon and R. T. Peterson,
In vivo drug discovery in the zebrafish, Nat. Rev. Drug Discovery, 2005, 4, 35 CrossRef CAS.
- X. Zhao, K. J. Ong, J. D. Ede, J. L. Stafford, K. W. Ng, G. G. Goss and S. C. Loo, Evaluating the toxicity of hydroxyapatite nanoparticles in catfish cells and zebrafish embryos, Small, 2013, 9, 1734 CrossRef CAS.
- F. Qing, Z. Wang, Y. Hong, M. Liu, B. Guo, H. Luo and X. Zhang, Selective effects of hydroxyapatite nanoparticles on osteosarcoma cells and osteoblasts, J. Mater. Sci.: Mater. Med., 2012, 23, 2245 CrossRef CAS.
- S. Li, S. Zhang, W. Chen and O. Wen, Effects of hydroxyapatite ultrafine powder on colony formation and cytoskeletons of MGC-803 cells, Bioceramics, 1996, 9, 225 CAS.
- Z. S. Liu, S. L. Tang and Z. L. Ai, Effects of hydroxyapatite nanoparticles on proliferation and apoptosis of human hepatoma bel-7402 cells, World J. Gastroenterol., 2003, 9, 1968 CAS.
- Q. Fu, N. Zhou, W. Huang, D. Wang, L. Zhang and H. Li, Preparation and characterization of a novel bioactive bone cement: Glass based nanoscale hydroxyapatite bone cement, J. Mater. Sci.: Mater. Med., 2004, 15, 1333 CrossRef CAS.
- Q. Fu, N. Zhou, W. Huang, D. Wang, L. Zhang and H. Li, Effects of nano HAP on biological and structural properties of glass bone cement, J. Biomed. Mater. Res., Part A, 2005, 74, 156 CrossRef.
|
This journal is © The Royal Society of Chemistry 2013 |