DOI:
10.1039/C3BM60034C
(Paper)
Biomater. Sci., 2013,
1, 1101-1110
Crystal structures of CaSiO3 polymorphs control growth and osteogenic differentiation of human mesenchymal stem cells on bioceramic surfaces†
Received
7th February 2013
, Accepted 21st June 2013
First published on 18th July 2013
Abstract
The repair and replacement of damaged or diseased human bone tissue requires a stable interface between the orthopedic implant and living tissue. The ideal material should be both osteoconductive (promote bonding to bone) and osteoinductive (induce osteogenic differentiation of cells and generate new bone). Partially resorbable bioceramic materials with both properties are developed by expensive trial-and-error methods. Structure–reactivity relationships for predicting the osteoinductive properties of ceramics would significantly increase the efficiency of developing materials for bone tissue engineering. Here we propose the novel hypothesis that the crystal structure of a bioceramic controls the release rates, subsequent surface modifications due to precipitation of new phases, and thus, the concentrations of soluble factors, and ultimately, the attachment, viability and osteogenic differentiation of human Mesenchymal Stem Cells (hMSCs). To illustrate our hypothesis, we used two CaSiO3 polymorphs, pseudowollastonite (psw, β-CaSiO3) and wollastonite (wol, α-CaSiO3) as scaffolds for hMSC culture. Polymorphs are materials which have identical chemical composition and stoichiometry, but different crystal structures. We combined the results of detailed surface characterizations, including environmental Scanning Electron Microscopy (SEM) back-scattered imaging, and spot-analysis and 2D elemental mapping by SEM-Energy Dispersive X-ray (SEM-EDX), High Resolution Transmission Electron Microscopy (HRTEM) and surface roughness analysis; culture medium solution analyses; and molecular/genetic assays from cell culture. Our results confirmed the hypothesis that the psw polymorph, which has a strained silicate ring structure, is more osteoinductive than the wol polymorph, which has a more stable, open silicate chain structure. The observations could be attributed to easier dissolution (resorption) of psw compared to wol, which resulted in concentration profiles that were more osteoinductive for the former. Thus, we showed that crystal structure is a fundamental parameter to be considered in the intelligent design of pro-osteogenic, partially resorbable bioceramics.
Introduction
An important goal in biomaterial design is to develop osteoinductive resorbable scaffolds and bone filler materials. The fundamental concept is that when seeded with osteoblasts, hMSCS, genes or other stimuli, such materials will promote osteogenic differentiation, new bone generation, and osseointegration, and thus minimize the possibility of failure at the implant/bone interface. Several material properties affect the behavior of cells seeded on scaffold and filler materials, and ultimately, the osteoconductivity and osteoinductivity of the material. These properties include chemical composition, surface texture, elastic modulus, porosity, permeability, and elastic modulus, as well as soluble factors added to the material.1–5 Certain conceptual criteria have been developed for rationalizing the osteoconductivity of silicate biomaterials,6–8 but general principles for predicting osteoinductivity do not yet exist.
In detail, silicate glasses and glass ceramics within only specific ranges of chemical compositions are osteoconductive. Soluble Ca, Si (as H4SiO4) and other ions, if present, are released by partial resorption (dissolution) of the silicate biomaterial. These ions combine with the Ca and PO4 ions present in the blood or cell culture medium and are reprecipitated as an interfacial hydroxyapatite (HAP) layer at the scaffold implant/living bone interface. This HAP layer favors implant–bone bonding (osteoconductivity) of bioactive calcium silicate (±Na2O–MgO–P2O5) glasses, glass ceramics and ceramics.9–13 The concepts of silicate glass network connectivity and “three-ring” structures consisting of silicate (SiO4) tetrahedra have only recently provided a structural explanation for the mechanism of osteoconductivity of bioactive calcium silicate glasses and ceramics.6–8,14,15 A chemical–structural conceptual basis for predicting the osteoinductivity of silicate glasses and ceramics, however, is less clear and is an area of active research.16–18
Progress is hindered, in part, because well-constrained experimental studies are rare, and the effects of chemical composition, crystal structure, crystallinity, and surface texture are often convoluted. Using pseudowollastonite (psw, β-CaSiO3) of different surface textures, thus keeping the crystal structure and chemical composition constant, we have previously shown that (a) crystallinity affects the osteoinductivity of CaSiO3 bioceramics, but (b) surface roughness differences within a factor of two do not influence osteoinductivity of CaSiO3.19 We now propose here that, all other factors being equal, the crystallographic structure of bioceramics is a critical factor in controlling their resorption (dissolution) rate, the release rates of osteoinductive soluble factors from the bioceramics, the subsequent surface modifications resulting from the potential precipitation of new phases, and ultimately, the activities of cells seeded on the bioceramic surfaces including cell adhesion, viability, and osteogenic differentiation.
In order to test this hypothesis, we devised a novel approach by examining psw and wollastonite (wol, α-CaSiO3) as model substrates for our study, because these two ceramics are polymorphs, having identical chemical composition and stoichiometry, but different crystallographic structures. This choice of bioceramics permits us to isolate the effects of crystal structure on osteoconductivity and osteoinductivity.10,20–22,23–27 We chose CaSiO3 because it is already known to be osteoconductive and two well-defined polymorphs are known to exist, thus providing an ideal model system to test our hypothesis.
Pseudowollastonite and wollastonite differ in their manner of polymerization of the silicate tetrahedra. Psw is the high temperature polymorph and its crystal structure consists of three silicate tetrahedra, which are covalently bonded via corner-sharing oxygens to form “3-rings” (note that “3” refers to the number of silicate tetrahedra and not to the number of atoms in the ring; Fig. 1 and ESI Fig. 1a,b†). The low temperature polymorph is wol, composed of corner-sharing silicate tetrahedra linked to form chains. The negative-charge associated with the silicate rings and chains, respectively, is electrostatically balanced by Ca2+ ions in both polymorphs. The high strain associated with the Si–O–Si bond angles in the “3-rings” of psw makes these bonds much more susceptible to hydrolysis compared to the more open, and hence, more stable Si–O–Si bonds in the wol chains. The effect of these crystallographic bonding differences in the two polymorphs results in greater psw solubility and resorption (dissolution) rate compared to wol.28 These differences in crystal structure-controlled resorption (dissolution) should result in different concentration profiles over time, which reflect a combination of different rates of released soluble Si and Ca and potentially different subsequent surface modifications from precipitation of new phases. We suggest that these differences should, ultimately, influence the attachment, viability, and osteogenic differentiation of hMSCs seeded at the bioceramic surfaces (Fig. 1).
 |
| Fig. 1 Schematic representation of the central hypothesis illustrated in our study by using two CaSiO3 polymorphs: bioceramic crystal structure (top panels) controls the solubility and dissolution rate, which control the levels and time-dependent trends of the soluble factors, Si and Ca (middle panels) and, ultimately, the activities of hMSCs seeded on the bioceramics (bottom panels). Legend for atoms in top panels: O = red, Si = blue, Ca = green. | |
Calcium silicate has been clinically used as dental root-end materials, and is being investigated for coatings on orthopedic implants.29,30 Our study of the polymorph crystal structure effect on soluble factor release and subsequent cell activities provides a new way to design biomaterials with an optimal clinical outcome. Another important contribution of our work is to use an interdisciplinary approach combining the detailed characterization of the pellet surfaces, cell culture medium solutions and molecular/genetic assays of cell activities at multiple time-points up to 28 days. By combining the results of these different analytical techniques, we were able to identify the effects of soluble factor concentration and release rates, and thus of the crystal polymorph structure, on hMSC activities, such as adhesion, viability, proliferation, and osteogenic differentiation.
Materials and methods
Pellet synthesis and characterization
Pseudowollastonite and wollastonite substrates were synthesized in the form of dense, disc-shaped pellets (12 mm diameter × 1 mm thick) by pressing 0.4 g of psw (Sigma, cat. #372668) or wol (Nyco®, NYAD 5000) ceramic powder (both of similar particle sizes∼ 1–2 μm), in a hardened steel die, followed by sintering at 900 °C for 10 hours, at a heating rate of 50 °C per min starting from room temperature (Lindberg Blue M tube furnace).19 After sintering, pellets were naturally cooled down at room temperature.
In order to ensure that sintering did not alter the crystal phase of the polymorphs, the crystallinity and purity of both phases were checked by X-Ray Diffraction (XRD) using a Scintag Pad V diffractometer with Cu Kα for both kinds of pellets both before and after sintering (ESI Fig. 2†). Mineral particles from both pellets after sintering were also characterized by High Resolution Transmission Electron Microscopy (HRTEM, Philips CM200 UT) (ESI Fig. 1c,d†). Detailed sample preparation procedures have been published elsewhere.19
A white light interferometer (Zygo New View) was used to measure the surface roughness (Ra), the average vertical deviation of the surface profile from the mean line over an area of 2.81 mm × 2.10 mm of the scaffold pellets. Values of Ra were 0.74 μm and 0.76 μm, respectively, for psw and wol before reaction, and ∼0.81 μm up to 28 days cell culture.
A Hitachi S-3400 Variable Pressure Scanning Electron Microscope (SEM) combined with Energy Dispersive X-Ray (EDX) was used to characterize pellet surfaces both before and after cell culture in terms of surface morphology and chemical composition. Details of sample preparations and instrument settings were published in our previous study.19 Briefly, pellet surfaces before cell culture were carbon-coated and SEM images revealed particle sizes of ∼10–20 μm for both psw and wol pellets (ESI Fig. 1e,f†). Pellet surfaces after cell culture were prepared by first lysing the cells, and then flushing the surfaces using a 0.2% Triton X-100 solution. The pellets were then dried under ambient conditions and carbon-coated.
Si, Ca, and P analysis in culture media. In order to monitor the soluble Ca, Si, and P in growth medium or osteogenic induction medium, solution samples were collected every two days from well-plates containing pellets seeded with hMSCs or control pellets without hMSCs. The medium samples were diluted 10 times and acidified by high purity HNO3 (15–16 M) until the pH dropped below 2. Using standard procedures, the samples were stored at 4 °C until they were analyzed by Inductively Coupled Plasma-Optical Emission Spectroscopy (ICP-OES, Varian Vista-MPX). Standard deviations of four replicates per condition were calculated to calculate the error bars.
Cell culture
Human Mesenchymal Stem Cells (hMSCs, Cambrex®) at passage 5 were used for cell growth and osteogenic differentiation studies on the psw and wol surfaces. For the cell growth study, cells were seeded at a density of 10
000 cells cm−2, and cultured in growth medium composed of 10.08 g L−1 Alpha Modification of Eagle's Medium (AMEM), 1% penicillin/streptomycin as antibiotics and 10% Fetal Bovine Serum (FBS). For the hMSC osteogenic differentiation study, the seeding density was 30
000 cells cm−2 in osteogenic induction medium composed of growth medium + 0.28 mM 2-phosphate ascorbic acid + 100 nM dexamethasone + 10 mM glycerol 2-phosphate.
In order to sterilize psw and wol substrates, pellets were first washed with anhydrous ethanol, and then incubated overnight at a temperature of 180 °C. The pellets were placed in twenty-four well culture plates (1 pellet per well), which were pre-coated with 1% BSA (Bovine Serum Albumin) overnight at 4 °C to prevent cell attachment and growth on the culture plate surfaces, thus allowing cells to grow only on the pellet surfaces. The hMSCs were incubated for 24 hours in GM to allow cell adhesion on the pellet surface after cell seeding. All cultures were maintained at 37 °C in a humidified incubator with 5% CO2. Media were changed every two days. The “day 0” time point in the following figures means 24 hours after hMSC seeding. Pellets in growth medium and osteogenic induction medium without cells served as negative controls. All the quantitative assays were carried out in quadruplicate. The entire set of experiments described below was conducted twice.
Cell adhesion, morphology, and viability in growth medium
To prepare the samples for LIVE/DEAD fluorescence imaging, the pellet surfaces were washed twice with Phosphate Buffered Saline (PBS). Fluorescent agents, calcein AM (calcein acetoxymethyl ester) and EthD-1 (ethidium homodimer) at concentrations of 2 μM and 4 μM, respectively, were added to the pellet sample and incubated for 40 min at room temperature. This assay identifies esterase activity in live cells via green fluorescence emission from calcein AM and nuclear permeability in dead cells via red fluorescence emission from EthD-1. Cell adhesion, morphology, and viability were then investigated by epifluorescence microscopy at days 0, 8, and 16.
Cell growth
Cells were seeded on the psw and wol pellet surfaces in quadruplicate at a low seeding density of 10
000 cells cm−2 and cultured in growth medium. Two assays were carried out to investigate cell growth on psw and wol substrates. Total DNA content was measured at days 0, 8, 12, and 20 using a CyQuANT® Assay Kit (Invitrogen). Total live cell number on substrates was determined using CellTiter Blue® reagent (Promega) at days 0, 2, 8, 12, 20, and 28. The underlying principle of this assay is that living cells are able to convert a redox dye named resazurin into a fluorescent end product, resorufin. Detailed procedures of this assay are listed on the manufacturer's website and in our previous publication.19 Fluorescence is measured with an excitation wavelength of 560 nm and an emission wavelength of 590 nm.
Osteoblastic cell differentiation in osteogenic induction medium
Analyses of alkaline phosphatase (ALP) enzymatic activity and the expression of three osteogenic genes, osteocalcin (OCN), osteopotin (OPN), and Runx2 or core binding factor alpha-1 (cbfa-1), are markers for different stages of bone formation. These analyses were combined with detailed characterizations of scaffold pellet surfaces to investigate osteogenic differentiation of the hMSCs.
The activity of ALP was measured at days 0, 8, 12, and 16 using standard procedures published previously.19 ALP concentrations in Fig. 2i were normalized by total DNA content as described above, and expressed as μg ALP per ng DNA after subtraction of the values obtained for cell-free controls, which contain hMSCs but no bioceramic pellet.
 |
| Fig. 2 Effects of psw and wol scaffolds on hMSC activities. (a, h) Effects on attachment, growth, and proliferation. The hMSCs were seeded at a density of 10 000 cells cm−2 in growth medium; (f) effects on hMSC osteogenic induction as indicated by alkaline phosphatase (ALP) enzymatic activity normalized by the total number of cells (DNA). The hMSCs were seeded at a density of 30 000 cells cm−2 in osteogenic induction medium. The expression of ALP on psw at day 0 was below the detection limit. (a–f) LIVE (green)/DEAD (red) fluorescence microscopy images of hMSCs on psw (a, b, c) and wol (d, e, f) surfaces at days 0 (a, d), 8 (b, e) and 16 (c, f). (g) Total DNA and (h) total live cell number over time in growth medium. Results are reported after subtraction of cell-free blanks. Asterisks indicate statistically significant differences (p < 0.05). | |
For RT-PCR, experiments were carried out in quadruplicate, at days 8 and 16, using osteogenic induction medium. Detailed protocols for RT-PCR experiments are available in our previous paper.19 Briefly, total RNA was extracted using an RNeasy® Mini kit (Qiagen) according to the manufacturer's instructions. For every sample, 50 ng of RNA was reverse transcribed into cDNA (complementary DNA) with Illustra™ Ready-to-Go RT-PCR beads. After first-strand cDNA synthesis, gene-specific primers (Table 1) were added into the sample for thermal cycling. A sample without gene-specific primers was used as the negative control. Electrophoresis was conducted at 100 V until the dye front has traveled approximately two-thirds of the gel length. Images were taken by placing the gel in a UV light box.
Table 1 Primer sequences used for the RT-PCR
Gene |
Sequence 5′ to 3′ |
Osteocalcin |
Forward |
GGCAGCGAGGTAGTGAAGAG |
|
Reverse |
CTGGAGAGGAGCAGAACTGG |
Osteopontin |
Forward |
TGAAACGAGTCAGCTGGATG |
|
Reverse |
TGAAATTCATGGCTGTGGAA |
Runx2 or Cbfα-1 |
Forward |
CACCGAGACCAACAGAGTCA |
|
Reverse |
TGCTTGCAGCCTTAAACTGA |
β-Actin |
Forward |
GGACTTCGAGCAAGAGATGG |
|
Reverse |
AGCACTGTGTTGGCGTACAG |
Results
Surface characterization of psw and wol pellets revealed their similar crystallinity and surface texture (ESI Fig. 1c–f†), and surface roughness (0.74 and 0.76 μm, respectively). Surface roughness variations within a factor of two between otherwise identical surfaces do not affect the osteoconductivity and pro-osteoinductivity of bioceramic surfaces.19
hMSC attachment, viability, and growth
The adhesion and viability of hMSCs at psw and wol surfaces were examined in growth medium up to 28 days (Fig. 2). On day 0, we observed a spherical cell morphology on psw, no cell–cell contacts, and several dead cells, indicating minimal adhesion, and suggest cytotoxicity of psw at this time point. In contrast, the cells were spindle-shaped on wol, no dead cells were observed, and the number of live cells was greater than on psw, indicating better initial attachment and viability and lower cytotoxicity. At day 8, hMSCs attained a spindle-morphology on psw, but still with minimal cell–cell contact, while on wol the spindle-shaped hMSCs began to aggregate and form clusters. Cells on both substrates reached confluence by day 16. Fluorescence images taken at day 20 and 28 (not shown) also showed that cells had reached confluence. These qualitative results are consistent with quantitative total DNA (Fig. 2g) and live cell number (viability) assays (Fig. 2h), which showed that cell number was initially lower on psw than on wol, but became statistically greater at days 20 and 28.
Osteogenic differentiation of hMSCs
Osteoblastic differentiation of hMSCs at psw and wol scaffold surfaces in osteogenic induction medium was monitored for 16 days (Fig. 2i). The upregulation of the enzyme, alkaline phosphatase (ALP), is a non-specific, but widely accepted indication of osteoblastic differentiation. The expression of ALP on psw peaked at day 8, but an obvious peak on wol was not observed (Fig. 2i). Alkaline phosphatase activity on psw was greater than that on wol at days 0, 8 and 12, but was lower by day 16. Thus, the upregulation of ALP was greater and earlier on psw than on wol. The osteoblastic differentiation of hMSC on both psw and wol was also supported by the expressions of OCN, OPN, and cbfα-1 genes as determined by our RT-PCR analyses (Fig. 3).
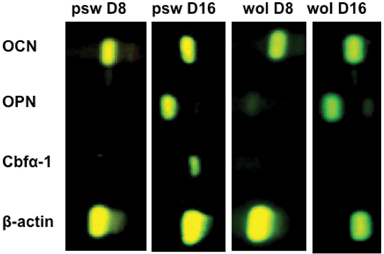 |
| Fig. 3 Osteogenic gene expressions determined by RT-PCR on psw and wol at different time points in osteogenic induction medium and at a seeding density of 30 000 cells cm−2. Genes reported are osteocalcin (OCN), osteopontin (OPN), core-binding factor alpha-1 (Cbfα-1), and β-actin as a house-keeping gene. Four replicates were processed for each condition. The result above is from one of the representative images. | |
Detailed surface characterizations of psw and wol scaffolds were conducted on day 12 of reaction in osteogenic induction medium (Fig. 4 and 5). In detail, we observed a layer of very fine-grained calcium phosphate background precipitate identified as hydroxyapatite and nodular amorphous silica on the surfaces of both cell-free controls (Fig. 4a,d, blue arrow) and cell-culture samples of psw and wol (Fig. 4b,e, blue arrow; Fig. 4c,f, green arrow). Polycrystalline aggregates, which were ∼20–50 μm across and 30 μm high, were seen only on the psw control (Fig. 4a, red arrow) and sample (Fig. 4b,c, red arrow), but not on the wol control and sample. Elemental mapping of Si, Ca and P on the reacted surfaces revealed very interesting patterns. The polycrystalline aggregates were deficient in Si and enriched in Ca, but not in P (Fig. 5a,b). Similar crystals were identified as calcite (CaCO3) using Kikuchi analysis in our previous work19 and are identified here also as calcite crystals. On the psw sample, some of the smaller, Si-deficient areas were co-localized with Ca and the P enriched areas, which were ∼5–2 μm in diameter (Fig. 5b). These regions were spatially highly correlated with the nodular amorphous precipitates seen in the corresponding SEM images (Fig. 4c, green arrow). The nodules were identified as CaP or bone nodules, consistent with our previous study (ESI Fig. 3†).19 This observation is important because bone nodule formation is the phenotype in cell culture corresponding to induction of bone formation in vivo. Significantly, the bone nodules were not seen on psw control surfaces without hMSCs indicating that their formation on psw samples is associated only with hMSC activity.
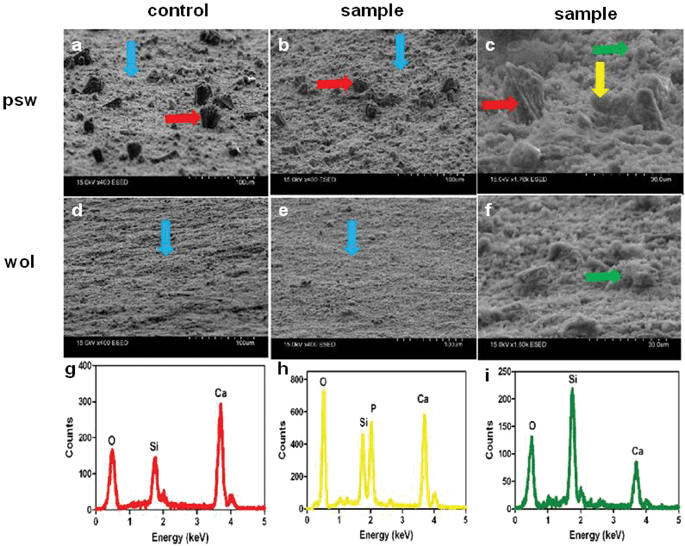 |
| Fig. 4 SEM image and SEM-EDX spot analysis of psw and wol surfaces after 12 days of hMSC culture in osteogenic induction medium at a seeding density of 30 000 cells cm−2. Cell-free controls were also studied. Images were obtained by SEM with an environmental secondary electron detector set at 75° angle to the pellet surfaces and using different magnifications. (a–c) psw and (d–f) wollastonite. (a) Cell-free psw control, (d) cell-free wol control, (b, c) psw samples with hMSCs, and (e, f) wol samples with hMSCs. Blue arrow: fine-grained, background, osteoconductive layer of HAP; green arrow: amorphous silica; red arrow: calcite; yellow arrow: amorphous Ca–PO4 bone nodule. (g–i) SEM-EDX spot analysis of polycrystalline aggregates of calcite (g), amorphous CaP bone nodule (h), and amorphous silica (i). Note that bone nodules were observed only on psw samples. | |
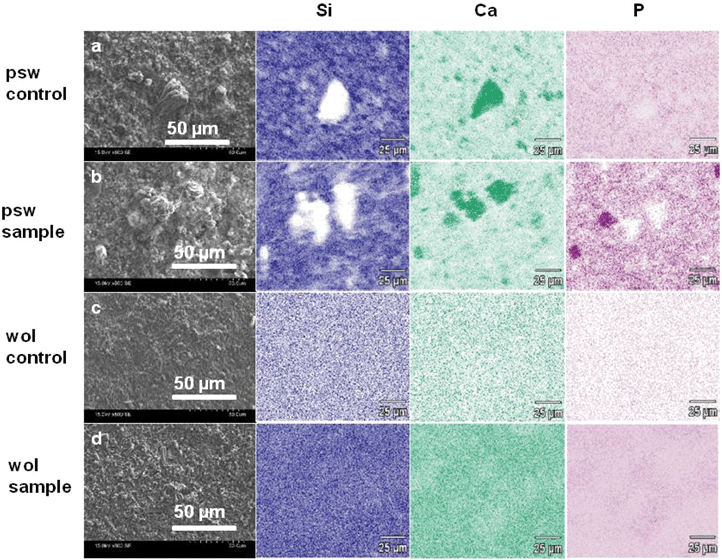 |
| Fig. 5 SEM image and elemental mapping (chemical composition analysis) over two-dimensional areas of (a, b), psw and (c, d), wol surfaces after 12 days of hMSC culture in osteogenic induction medium at a seeding density of 30 000 cells cm−2. (a–d) SEM images with a secondary electron detector. SEM-EDX spectral maps of Si (dark blue), Ca (light green), and P (purple) over a 0.15 mm × 0.10 mm area. (a) Cell-free control psw, (b) sample psw, (c) cell-free control wol, and (d) sample wol. In the spectral maps, lighter and darker colors, respectively, indicate lower and higher concentrations of the element. | |
Release of soluble factors
In order to determine the effects of the polymorphs’ crystal structures on soluble factor concentrations and to relate these factors to hMSC activities (adhesion, viability, growth and osteoinduction), we determined the concentrations of Si, Ca, and P in both growth medium and osteogenic induction medium (Fig. 6). The trends and absolute concentrations for Si and Ca levels over time were similar in both culture media, suggesting that the same reaction mechanisms controlled Si and Ca concentrations in both media. Silicon concentrations peaked at higher levels and earlier time points for psw than for wol (Fig. 5a,b).
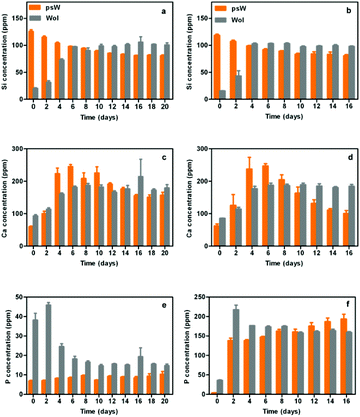 |
| Fig. 6 Soluble factor analyses in growth medium (GM) and osteogenic induction medium (IM) from psw and wol cell culture samples. (a, b) Si, (c, d) Ca and (e, f) P. (a, c, e) Concentrations of Si, Ca, and P from GM at a cell seeding density of 10 000 cells cm−2. (b, d, f) Concentrations of Si, Ca, and P from IM at a cell seeding density of 30 000 cells cm−2. | |
This result is consistent with our expectation that the three-ring silicate structure of the psw polymorph will have a higher solubility and dissolution (resorption) rate versus the chain silicate structure of the wol polymorph and potentially different surface precipitates forming. The eventual attainment of steady-state Si values on both surfaces indicated that the Si release rate by dissolution of the underlying substrate became equal to the Si removal rate by amorphous silica precipitation. The higher solubility and faster dissolution rate of psw relative to wol also released a higher Ca concentration and at a faster rate (Fig. 4c,d). The released Ca was removed from solution by first combining it rapidly with phosphate from the culture medium to form the background, fine-grained, osteoconductive layer of HAP. The amount of Ca removed is dictated by the solubility limit of HAP. Excess soluble Ca released from the psw sample combined with carbonate from the culture medium to form large, polycrystalline aggregates of metastable calcite. Such behaviour of the dissolution products of CaSiO3 bioceramic has been reported previously.31 These calcite crystals were not seen on wol (Fig. 4a,b), because of the slower dissolution rate of wol related to its more stable chain-silicate structure, such that smaller Ca levels were released. Thus, only the background CaP or HAP osteoconductive layer was formed. Finally, we note that the source of phosphate for the formation of the background, osteoconductive HAP layer is the soluble P which was already present in the starting culture media. Trends in P concentrations with time were closely related to those of Ca, suggesting that P levels are indirectly affected by the crystal structure of the two polymorphs.
Discussion
In general, cells respond to both the substrate physical properties of and soluble factors released by a resorbable scaffold. The hypothesis for the present study is that, if all other factors are equal, then the intrinsic crystal structure of biomaterials controls their solubility, dissolution rate, and subsequent surface modifications by precipitation, which will be reflected in the soluble factor concentrations and trends over time, ultimately influencing hMSC initial attachment and viability, growth, proliferation, and osteogenic differentiation. In our study, (1) the chemical composition and stoichiometry of both polymorphs are identical; (2) the surface roughness for both surfaces did not change significantly before and after cell culture experiments, and we have shown previously that the variation of surface roughness within a factor of 2 does not cause significant changes in cell activities; and (3) other surface textural effects are minimal because of the similar grain size of the starting powders of each polymorph and the similar pore sizes (ESI Fig. 1†).32,33 Thus, our study design enabled us to separate out the effects of only the crystal structure and soluble factor release on hMSC activities, and we discuss our results in this context.
Effect of soluble factors on cell growth
High Si concentrations >120 ppm released from a variety of silicate biomaterials including Bioglass®, zeolites and pseudowollastonite2,19,34,35 are cytotoxic towards osteoblasts, whereas a lower soluble Si concentration of 16 ppm from Bioglass® 45S5 promoted human osteoblast proliferation (ESI Fig. 4†).16 The significantly higher initial Si levels released from psw than from wol, in the present study, explains the greater initial cytotoxicity of psw towards the hMSCs, as well as the hMSC proliferation trends. Higher initial Si concentrations from psw “shocked” the hMSCs more compared with wol, with a corresponding lower cell growth. By day 10, however, cells on psw gradually recovered as Si concentrations decreased, while Si levels for wol increased to ∼98 ppm. The number of cells on psw, therefore, gradually caught up with the population on wol by day 10. Subsequently, Si concentrations on psw reached a steady state value of ∼80 ppm versus ∼100 ppm on wol. Thus, a higher proliferation rate and a higher total cell number were achieved eventually on psw.
Effect of soluble factors on cell osteogenic differentiation
Significantly higher and much earlier activity of ALP was observed on psw than on wol. The formation of bone nodules was also seen only on psw. Thus, greater and earlier osteoblastic differentiation occurred in psw cell culture than in wol. Both ALP and RT-PCR results, however, suggest that some limited extent of osteoblastic differentiation did occur on wol, but did not proceed to bone nodule formation at least up to day 12. Previous studies have shown that Si concentrations <19 ppm or Ca concentrations of 240–600 ppm favor osteoblastic differentiation.16,17,34–37 Our ongoing experiments indicate that Si is osteoinductive to hMSCs at concentrations up to 100 ppm in osteogenic induction medium (unpublished data). In the present study, Si concentration peaked earlier and reached higher levels in the psw samples compared to wol. Furthermore, the Ca concentration peaked at >240 ppm for psw and concentrations were <240 ppm for wol over the entire period of the experiment. Thus, we have shown that the crystal structure of a bioceramic controls the concentrations of soluble factors, which ultimately influence the osteoinductivity of the ceramic. Secondly, not just the concentrations but also the time-release profile (release rates) rates are significant for influencing osteoinductivity. Finally, we note that, when comparing the results of different studies in the literature for the effects of soluble factors, it is critical to compare only those studies in which the results are for the same type of cells or cell lines.
We did not observe a close correlation of ALP expression with total dissolved P concentrations in our study. Performing a detailed discussion of the pro-osteoinductive effects of soluble P is complicated for several reasons. In one study,38 for example, a bioactive glass scaffold with the lowest inorganic P content was reported to promote osteoblast differentiation, but the effect was convoluted with the highest soluble Ca concentration. Furthermore, inorganic phosphate concentrations from 128 to 320 ppm have also been reported to induce the expression of the OPN gene (a marker for osteoinduction) when low-passage mouse osteoblastic cells MC3T3-E were cultured with a sodium phosphate supplement up to 21 days.39 These inorganic P supplements, however, did not promote the expression of another osteogenic gene, osteocalcin, adding even more complexity to the effect of P on osteoblastic differentiation. Finally, the total P concentrations measured here included inorganic and organic P, which changed constantly due to hydrolysis of osteogenic induction supplements (e.g., β-glycerol phosphate) by alkaline phosphatase.
Conclusions
Calcium silicate is a promising biomaterial for bone regeneration because it is bioactive and can release soluble factors that enhance cell activity and new bone formation. We studied the crystal structure effects of two CaSiO3 polymorphs via substrate dissolution and surface precipitation on the levels of soluble factors and the subsequent hMSC adhesion, growth, proliferation, and differentiation. The initial hMSC adhesion and viability on psw was lower than that on wol, because of the initially higher, cytotoxic Si levels obtained from psw due to its unstable “three-ring silicate” crystal structure compared to the “chain-silicate” structure of wol. Subsequently, a smaller inhibition of osteogenic differentiation by lower steady-state Si levels and a greater pro-osteoinductivity by higher Ca steady-state levels from psw resulted in earlier osteogenic differentiation on psw than on wol. Our results support the hypothesis that, all other factors being equal, the crystal structure of resorbable bioceramics can help predict the soluble factor levels and, thus, the behavior of seeded hMSCs on scaffold surfaces. Accounting for the effects of crystal structure contributes towards developing a rational approach to discovering novel, pro-osteoinductive, bioceramics for bone-tissue engineering.
Acknowledgements
The authors are grateful for financial support from the NSF CAREER award (EAR 0346689), NSF DMR (0906817), Wisconsin Alumni Research Foundation (WARF) awards and start-up funds from the University of Akron to Nita Sahai; a Bailey Fellowship and summer research support to Nianli Zhang from the Department of Geoscience, University of Wisconsin–Madison; and AO Research Foundation Fund F-07-65M to Bill Murphy. The authors are also thankful for useful discussions with members of the Sahai research group. N.S. is grateful to the late Prof. Michel Anseau, University of Mons-Hainuat, for his encouragement of this research at its inception.
Notes and references
- P. Ducheyne and Q. Qiu, Bioactive ceramics: the effect of surface reactivity on bone formation and bone cell function, Biomaterials, 1999, 20(23–24), 2287–2303 CrossRef CAS
.
- R. C. Bielby, I. S. Christodoulou, R. S. Pryce, W. J. P. Radford, L. L. Hench and J. M. Polak, Time- and concentration-dependent effects of dissolution products of 58S sol-gel bioactive glass on proliferation and differentiation of murine and human osteoblasts, Tissue Eng., 2004, 10(7–8), 1018–1026 CAS
.
- V. Karageorgiou and D. Kaplan, Porosity of 3D biomaterial scaffolds and osteogenesis, Biomaterials, 2005, 26(27), 5474–5491 CrossRef CAS
.
- G. Stylios, T. Y. Wan and P. Giannoudis, Present status and future potential of enhancing bone healing using nanotechnology, Injury, 2007, 38, S63–S74 CrossRef
.
- F. Rosso, A. Giordano, M. Barbarisi and A. Barbarisi, From cell-ECM interactions to tissue engineering, J. Cell. Physiol., 2004, 199(2), 174–180 CrossRef CAS
.
- N. Sahai and J. A. Tossell, Molecular orbital study of apatite nucleation at silica bioceramic surfaces, J. Phys. Chem. B, 2000, 104, 4322–4341 CrossRef CAS
.
- N. Sahai and R. M. Anseau, Cyclic silicate active site and epitaxial apatite nucleation on pseudowollastonite bioceramic-bone interfaces, Biomaterials, 2005, 26, 5763–5370 CrossRef CAS
.
-
M. Cerruti and N. Sahai, Silicate biomaterials for orthopaedic and dental implants, in Medical mineralogy and geochemistry, ed. N. Sahai and M. Schoonen, Reviews in Mineralogy and Geochemistry, 2006, vol. 64, pp. 283–313 Search PubMed
.
- P. N. De Aza, Z. B. Luklinska and M. Anseau, Bioactivity of diopside ceramic in human parotid saliva, J. Biomed. Mater. Res., B, 2005, 73(1), 54–60 CrossRef
.
- N. P. De Aza, B. Z. Luklinska, R. M. Anseau, F. Guitian and S. De Aza, Morphological studies of pseudowollastonite for biomedical application, J. Microscopy, 1996, 182(Pt. 1), 24–31 Search PubMed
.
- K. L. Lin, W. Y. Zhai, S. Y. Ni, J. Chang, Y. Zeng and W. J. Qian, Study of the mechanical property and in vitro biocompatibility of CaSiO3 ceramics, Ceram. Int., 2005, 31(2), 323–326 CrossRef CAS
.
- S. Y. Ni and J. Chang,
In vitro degradation, bioactivity, and cytocompatibility of calcium silicate, dimagnesium silicate, and tricalcium phosphate bioceramics, J. Biomater. Appl., 2009, 24(2), 139–158 CrossRef CAS
.
- T. Nonami and S. Tsutsumi, Study of diopside ceramics for biomaterials, J. Mater. Sci.: Mater. Med., 1999, 10(8), 475–479 CrossRef CAS
.
- R. Hill, An alternative view of the degradation of bioglass, J. Mater. Sci. Lett., 1996, 15(13), 1122–1125 CrossRef CAS
.
- A. Tilocca, Structural models of bioactive glasses from molecular dynamics simulations, Proc. R. Soc. London, Ser. A, 2009, 465(2104), 1003–1027 CrossRef CAS
.
- I. D. Xynos, A. J. Edgar, L. D. K. Buttery, L. L. Hench and J. M. Polak, Ionic products of bioactive glass dissolution increase proliferation of human osteoblasts and induce insulin-like growth factor II mRNA expression and protein synthesis, Biochem. Biophys. Res. Commun., 2000, 276(2), 461–465 CrossRef CAS
.
- I. D. Xynos, M. V. J. Hukkanen, J. J. Batten, L. D. Buttery, L. L. Hench and J. M. Polak, Bioglass (R) 45S5 stimulates osteoblast turnover and enhances bone formation in vitro: implications and applications for bone tissue engineering, Calcif. Tissue Int., 2000, 67(4), 321–329 CrossRef CAS
.
- M. Bohner, Silicon-substituted calcium phosphates – a critical view, Biomaterials, 2009, 30(32), 6403–6406 CrossRef CAS
.
- N. L. Zhang, J. A. Molenda, J. H. Fournelle, W. L. Murphy and N. Sahai, Effects of pseudowollastonite (CaSiO3) bioceramic on in vitro activity of human mesenchymal stem cells, Biomaterials, 2010, 31(30), 7653–7665 CrossRef CAS
.
- N. P. De Aza, B. Z. Luklinska, R. M. Anseau, F. Guitian and S. De Aza, Transmission electron microscopy of the interface between bone and pseudowollastonite implant, J. Microscopy, 2001, 201(pt. 1), 33–43 CrossRef
.
- P. N. De Aza, Z. B. Luklinska, M. R. Anseau, F. Guitian and S. De Aza, Bioactivity of pseudowollastonite in human saliva, J. Dentistry, 1999, 27(2), 107–113 CrossRef CAS
.
- P. N. de Aza, Z. B. Luklinska, A. Martinez, M. R. Anseau and F. Guitian, Morphological and structural study of pseudowollastonite implants in bone, J. Microscopy, 2000, 197, 60–67 CrossRef CAS
.
- X. Y. Liu, C. X. Ding and P. K. Chu, Mechanism of apatite formation on wollastonite coatings in simulated body fluids, Biomaterials, 2004, 25(10), 1755–1761 CrossRef CAS
.
- X. Y. Liu, C. X. Ding and Z. Y. Wang, Apatite formed on the surface of plasma-sprayed wollastonite coating immersed in simulated body fluid, Biomaterials, 2001, 22(14), 2007–2012 CrossRef CAS
.
- S. Y. Ni, K. L. Lin, J. Chang and L. Chou, Beta-CaSiO3/beta-Ca-3(PO4)(2) composite materials for hard tissue repair: in vitro studies, J. Biomed. Mater. Res., Part A, 2008, 85(1), 72–82 CrossRef
.
- J. Wei, F. P. Chen, J. W. Shin, H. Hong, C. L. Dai, J. C. Su and C. S. Liu, Preparation and characterization of bioactive mesoporous wollastonite – polycaprolactone composite scaffold, Biomaterials, 2009, 30(6), 1080–1088 CrossRef CAS
.
- C. T. Wu, Y. Ramaswamy, P. Boughton and H. Zreiqat, Improvement of mechanical and biological properties of porous CaSiO3 scaffolds by poly(D,L-lactic acid) modification, Acta Biomater., 2008, 4(2), 343–353 CrossRef CAS
.
- H. W. Casey, R. H. Westrich, F. J. Banfield, G. Ferruzzi and W. G. Arnold, Leaching and reconstruction at the surfaces of dissolving chain-silicate minerals, Nature, 1993, 366, 253–256 CrossRef
.
- M. G. Gandolfi, P. Taddei, F. Siboni, E. Modena, G. Ciapetti and C. Prati, Development of the foremost light-curable calcium-silicate MTA cement as root-end in oral surgery. Chemical-physical properties, bioactivity and biological behavior, Dental Mater., 2011, 27(7), E134–E157 CrossRef CAS
.
- S. Xu, K. Lin, Z. Wang, J. Chang, L. Wang, J. Lu and C. Ning, Reconstruction of calvarial defect of rabbits using porous calcium silicate bioactive ceramics, Biomaterials, 2008, 29(17), 2588–2596 CrossRef CAS
.
- Y. Iimori, Y. Kameshima, K. Okada and S. Hayashi, Comparative study of apatite formation on CaSiO3 ceramics in simulated body fluids with different carbonate concentrations, J. Mater. Sci. Mater. Med., 2005, 16(1), 73–79 CrossRef CAS
.
- T. P. Kunzler, T. Drobek, M. Schuler and N. D. Spencer, Systematic study of osteoblast and fibroblast response to roughness by means of surface-morphology gradients, Biomaterials, 2007, 28(13), 2175–2182 CrossRef CAS
.
- S. K. Nishimoto, M. Nishimoto, S. W. Park, K. M. Lee, H. S. Kim, J. T. Koh, J. L. Ong, Y. X. Liu and Y. Z. Yang, The effect of titanium surface roughening on protein absorption, cell attachment, and cell spreading, Int. J. Oral Maxillofac. Implants, 2008, 23(4), 675–680 Search PubMed
.
- I. Christodoulou, L. D. K. Buttery, P. Saravanapavan, G. P. Tai, L. L. Hench and J. M. Polak, Dose- and time-dependent effect of bioactive gel-glass ionic-dissolution products on human fetal osteoblast-specific gene expression, J. Biomed. Mater. Res., Part B, 2005, 74(1), 529–537 CrossRef
.
- P. E. Keeting, M. J. Oursler, K. E. Wiegand, S. K. Bonde, T. C. Spelsberg and B. L. Riggs, Zeolite-a increases proliferation, differentiation, and transforming growth-factor-beta
production in normal adult human osteoblast-like cells-in vitro, J. Bone Miner. Res., 1992, 7(11), 1281–1289 CrossRef CAS
.
- O. Tsigkou, J. R. Jones, J. M. Polak and M. M. Stevens, Differentiation of fetal osteoblasts and formation of mineralized bone nodules by 45S5 Bioglass (R) conditioned medium in the absence of osteogenic supplements, Biomaterials, 2009, 30(21), 3542–3550 CrossRef CAS
.
- S. Maeno, Y. Niki, H. Matsumoto, H. Morioka, T. Yatabe, A. Funayama, Y. Toyama, T. Taguchi and J. Tanaka, The effect of calcium ion concentration on osteoblast viability, proliferation and differentiation in monolayer and 3D culture, Biomaterials, 2005, 26(23), 4847–4855 CrossRef CAS
.
- S. Lossdorfer, Z. Schwartz, C. H. Lohmann, D. C. Greenspan, D. M. Ranly and B. D. Boyan, Osteoblast response to bioactive glasses in vitro correlates with inorganic phosphate content, Biomaterials, 2004, 25(13), 2547–2555 CrossRef CAS
.
- G. R. Beck, B. Zerler and E. Moran, Phosphate is a specific signal for induction of osteopontin gene expression, Proc. Natl. Acad. Sci. U. S. A., 2000, 97(15), 8352–8357 CrossRef CAS
.
Footnote |
† Electronic supplementary information (ESI) available. See DOI: 10.1039/c3bm60034c |
|
This journal is © The Royal Society of Chemistry 2013 |
Click here to see how this site uses Cookies. View our privacy policy here.