DOI:
10.1039/C2BM00138A
(Paper)
Biomater. Sci., 2013,
1, 285-293
Combined near-IR photothermal therapy and chemotherapy using gold-nanorod/chitosan hybrid nanospheres to enhance the antitumor effect†
Received
17th September 2012
, Accepted 17th October 2012
First published on 6th November 2012
Abstract
Motivated by the enhanced cytotoxicity of some chemotherapeutic agents at temperature rise, multifunctional chitosan nanospheres which co-carried gold nanorods and cisplatin were prepared. These hybrid nanospheres were characterized by dynamic light scattering, zeta potential, UV-Vis absorption spectra and transmission electron microscopy. It was demonstrated that these 120 nm-sized hybrid nanospheres could be selectively accumulated at the tumor site with about 11% injection dose per g of tumor, and produced local hyperthermia to an average temperature of 49 °C in tumor tissue after near-IR irradiation for 10 min. Due to the enhanced cytotoxicity of cisplatin at elevated temperatures, cisplatin-loaded hybrid nanospheres showed about one-second lower IC50 values than hybrid nanospheres alone in vitro and almost complete tumor growth inhibition in vivo. Compared with chemotherapy or photothermal treatment alone, the combined photothermal therapy and chemotherapy had a significantly synergistic effect and improved the therapeutic efficacy, which was supported by immunofluorescence staining and in vivo apoptosis imaging.
Introduction
Photothermal therapy (PTT) for treating solid tumors mediated by near-infrared (NIR) radiation has attracted considerable interest, since this technique involves the process of strongly absorbing NIR light and locally producing cytotoxic heat to kill cancer cells.1,2 Various photothermal agents, such as gold nanoshells,3,4 gold nanocages,5,6 gold nanorods,7,8 carbon nanotubes9,10 and graphene11 have been reported to deal with malignant tumors, with moderate success. However, complete tumor ablation is still hard to achieve and marginal recurrence is unavoidable, since local photothermal cytotoxicity is not sufficient to eliminate the diffused cancerous cells12 and heat transfer in organisms is quite complicated.13 In order to enhance the therapeutic efficacy, the combination of PTT with chemotherapy is highly desirable due to the synergistic effect.14 It has been reported that some chemotherapeutic agents have enhanced cytotoxicity at elevated temperatures.15 Although the mechanism of heat induced enhancement in cytotoxicity is not completely known, these drugs are expected to be useful to assist PTT therapy which has the obvious temperature raise.16 On the other hand, combining drug with heating can lower the drug dosage requirements to achieve comparable cytotoxicity of the unheated drug amount, and this has important implications to the dose-dependent side-effect of chemotherapeutic agents. Since traditional chemotherapeutic drugs bring severe side-effects such as liver and kidney toxicity as well as cardiotoxicity,17 the combined therapy is expected to minimize systemic side-effects of chemotherapeutic agents by lowering the drug doses.18 Thus, to achieve good therapeutic efficacy and low systemic toxicity, the combined therapy is being actively pursued. However, up to now, most of them focus on the combined gold nanoshells or nanorods with doxorubicin treatment,17,19–22 while the synergistic effect of gold nanorods and cisplatin has not been investigated except for the only in vitro report.23 It has been demonstrated that the thermal enhancement ratios (TERs) of cytotoxicity of doxorubicin and cisplatin are different. At temperatures of 43.5 °C, the TER of doxorubicin and cisplatin is 1 and 1.6, respectively.24 Moreover, a linear enhancement in the cytotoxic effect for cisplatin is observed if the temperature rises from 37 °C to over 40 °C.
Previously, we have developed chitosan/gold nanorod (CS/AuNR) multifunctional nanocarriers, fabricated by a simple nonsolvent-aided counterion complexation method, and anticancer drug cisplatin was loaded into the hybrid nanospherical CS matrix space, which can be used for cell imaging, drug delivery and NIR photothermal therapy.25 More importantly, their unique hybrid structure with gold nanorods as metal cores and cisplatin in spherical shells is a good candidate for the co-delivery of photothermal agents and chemotherapeutic agents into the tumor site. Therefore, this multifunctional system possesses an enormous potential to perform the combination therapy. However, further study on the feasibility of combination therapy has been not put into practice, especially for in vivo models.
In this work, we focus on the enhanced antitumor efficiency based on the synergistic effect of hyperthermia and cisplatin in both in vitro and in vivo cases. We hypothesize that integrating gold nanorods and cisplatin into 120 nm-sized CS nanospheres and utilizing an enhanced permeability and retention (EPR) effect-driven tumor accumulation will simultaneously achieve near IR induced hyperthermia and the thermal enhancement of cytotoxicity of cisplatin in tumor sites, resulting in multiple tumor suppression and complete tumor ablation. As gold nanorods are excellent photothermic conversion materials26 and cisplatin has the best synergistic effects with heat,27 a very impressive therapeutic effect in vivo is found in the present work. An almost complete destruction of the tumor is achieved with the quite lower possibility of recurrence than the PTT alone. In addition, the combination therapy can not only lower the dose of cisplatin with minimal side-effects but also improve the therapeutic efficacy. Therefore, this work provides a simple strategy of using multifunctional nanocarriers as a combinational application of photothermal therapy and chemotherapy for cancer therapy.
Experimental
Materials
Chitosan (CS) with a number-average molecular weight (Mn) of 5000 was purchased from Yuhuan Biomedical Company (Zhejiang, China) and used without further purification. Ethylene diamine tetraacetic acid (EDTA), cetyltrimethylammonium bromide (CTAB), glutaraldehyde (GA), sodium borohydride (NaBH4), chloroauric acid (HAuCl4), cis-diamine-dichloroplatinum(II) (cisplatin), 3-(4,5-dimethylthiazol-2-yl)-2,5-diphenyltetrazolium bromide (MTT) and NIR-797 isothiocyanate were purchased from Sigma-Aldrich and used as received. Annexin-Vivo 750 was provided by Perkin-Elmer. Human cervical carcinoma HeLa cell line and murine hepatic H22 cell line were obtained from Shanghai Institute of Cell Biology (Shanghai, China). Male ICR mice (6–8 weeks, 20–22 g) were purchased from Animal Central Laboratory of Nanjing Medical University (Nanjing, China).
Preparation of chitosan/gold nanorod hybrid nanospheres (CS-AuNR NSs)
The CS-AuNR NSs were prepared by a nonsolvent counterion complexation method.25 Briefly, gold nanorods were first synthesized by the classical seed-mediated growth method,28 and then CTAB was removed by repeated centrifuging and washing with distilled water. For the preparation of hybrid nanospheres, 10 mg CS and 2 mg EDTA were initially dissolved in 1 mL water and then mixed with 1 mL concentrated AuNR solution (1.2 mg Au mL−1). The resultant mixture was sonicated for 2 min. After that, 2 mL ethanol (nonsolvent) was added dropwise to the system with moderate stirring. During this process, a change of the clear solution to opalescent was observed, indicating the formation of CS-AuNR NSs. Finally, the obtained CS-AuNR NSs were crosslinked by 6 μL GA solution (25%) for 4 h at room temperature, and purified by dialyzing against distilled water to remove the EDTA and the ethanol. The empty CS NSs were prepared similarly in the absence of AuNR.
Characterization of hybrid nanospheres
Morphology and structure of the resulting nanospheres were observed by transmission electron microscopy (TEM, JEM-100S, JEOL, Japan) and scan electron microscopy (SEM, JED-2300, JEOL, Japan). The hydrodynamic diameters and distribution of the hybrid nanospheres were determined by a dynamic light scattering (DLS) method using a Brookhaven BI-9000AT instrument (Brookhaven Instruments Corporation, USA) using a wavelength of 633.0 nm at 25 °C with a detection angle of 90°. The zeta potential of the nanospheres was obtained with a Zetaplus (Brookhaven Instruments Corporation). The results were the average of triplicate runs. The UV-vis absorption spectra were recorded on a spectrophotometer (UV3100, Shimadzu, Japan). Thermogravimetric analyses (TGA) were performed using a TAC71DX thermal analyzer under air flow in the temperature range 25–800 °C at a heating rate of 20 °C min−1. The gold concentrations were quantified using inductively coupled plasma-mass spectrometry (ICP-MS, Perkin-Elmer Corporation, USA). Digestion of the samples was performed in concentrated nitric acid and heated to 90 °C for 12 h before measurements. All results were the average of triplicate measurements.
Drug-loaded hybrid nanospheres
The cisplatin-loaded hybrid nanospheres (CS-AuNR-Pt NSs) were prepared by mixing a predetermined CS-AuNR NS suspension (2 mg mL−1, 5 mL) with cisplatin aqueous solution (2 mg mL−1, 0.5 mL) in a shaking incubator (37 °C, 100 rpm min−1) overnight. Then the residual free cisplatin in the prepared CS-AuNR-Pt NS suspension was removed by centrifugation at 12 krpm for 30 min, and the sediment, i.e. CS-AuNR-Pt NSs, was re-dispersed in distilled water. The amount of free cisplatin in the supernatant was analyzed by a modified o-phenylenediamine colorimetric method at a wavelength of 704 nm.29 The drug-loading content and encapsulation efficiency were calculated by the following equations:
Drug release in vitro
The release profile of cisplatin from CS-AuNR NSs was evaluated by the dialysis method in PBS (0.01 M, pH 7.4) at 37 °C and 50 °C. Briefly, the freeze-dried CS-AuNR-Pt NS samples (10 mg) were placed in a pre-swelled dialysis bag (12 kDa cut-off, 2.5 × 2.5 cm). Then the bag was immersed in 5 mL PBS buffer and kept in an incubator. The release medium was withdrawn at given time intervals and equivalent fresh PBS buffer was added to the system. The concentration of cisplatin in the release medium was determined by the colorimetric method described above. Each result was an average of triplicate.
NIR797 dye (1 mg in 0.2 mL DMF) was added dropwise into the CS-AuNR NS solution (2 mg mL−1, 5 mL) and magnetically stirred at room temperature for 24 h in the dark. The residual unreacted NIR797 in the suspension was removed by centrifugation at 15 krpm for 30 min, and the sediment, i.e. NIR797-labeled CS-AuNR NSs (CS-AuNR-NIR797 NSs), was re-dispersed in distilled water for further use.
All the animal experiments were reviewed and approved by Animal Care and Use Committee, Nanjing University. 5 × 105 murine H22 cells in 0.1 mL saline were subcutaneously inoculated into the right limb armpits of every ICR male mouse. The mouse was kept for 7 days with free access to food and water. After that, CS-AuNR-NIR797 NSs (2 mg mL−1, 0.2 mL) were injected into the mice bearing the tumor through a tail vein. The time-dependent distribution of CS-AuNR-NIR797 NSs in mice was imaged using the Maestro in vivo fluorescence imaging system (CRi Inc., USA). The mice were anesthetized with isoflurane and the scans were carried out at 1 h, 4 h, 8 h, 24 h, 48 h, 72 h and 96 h post-injection. Thereafter, the mice bearing tumors were sacrificed and the tumor, heart, liver, spleen, lung, kidney, brain, intestine and stomach were harvested for isolated organ imaging.
In vivo biodistribution
Mice bearing the tumor were intravenously injected with CS-AuNR NSs at a dosage of 50 mg kg−1 (7.5 mg AuNR eq.) and sacrificed at 1 h, 4 h, 8 h, 24 h, 48 h, 72 h and 96 h post-injection (n = 3 at each time point), respectively. Subsequently, the tumor, liver, spleen, kidney, lung, heart and blood were excised and collected. The organs were weighed and decomposed in hot nitric acid to a colorless solution. After being evaporated to dryness, they were dissolved in 3.0 mL dilute nitric acid solution (1%). Then the Au concentration in the solution was measured by inductively coupled plasma-mass spectrometry (ICP-MS, Perkin-Elmer Corporation, USA). The data were normalized to the tissue weight and expressed as a percentage of injection dose/g organ at each test point.
For TEM observation, the tumor tissues of mice were excised at 8 hours after CS-AuNR NS injection and immediately fixed by immersion in a solution containing 3% glutaraldehyde and 4% formaldehyde in 0.01 M phosphate buffer (pH 7.4) for 2 h. The tissues were post-fixed for 2 h in 1% osmium tetroxide. Following dehydration by immersing in serially diluted aqueous ethanol solutions, the specimens were embedded in epoxy resin and the sections of about 70 nm thick were obtained by microtoming the resin sample at room temperature. The ultra-thin sections were stained with lead citrate and uranyl acetate, and viewed by TEM (JEM-100S, JEOL, Japan).
In vivo photothermal therapy
Mice bearing a tumor were injected with CS-AuNR NSs (50 mg kg−1) in the tail vein (0.2 mL per mouse), and irradiated for 8 h post-injection under the stabilized NIR fiber coupled laser system (LE-LS-808-3000TFC-D, Leo Photonics Company, Shanghai). The laser device has a 200 μm diameter fiber with a center wavelength at 808 nm ± 5 nm, and the beam diameter was expanded to 11.4 mm (∼1 cm2) by optical lens which can cover the entire tumor area. The output power density was stabilized at 2 W cm−2. The tumor area of the mice was depilated and exposed under the laser for 10 min irradiation. The irradiation-induced temperature rise was recorded by a highly sensitive infrared thermographic camera (ThermaCAMTM SC3000, FLIR, USA). The image was analyzed by ThermaCAM Researcher Professional 2.7 software.
In vitro irradiation-induced cytotoxicity
The in vitro cytotoxicity of CS-AuNR-Pt NSs was determined by standard MTT assays using human epithelial carcinoma cell lines, HeLa, as model. 5000 cells per well were seeded into a 96-well plate and incubated at 37 °C in a humidified atmosphere with 5% CO2. The culture medium was high-glucose Dulbecco's modified eagle's medium (DMEM), supplemented with 10% calf blood serum. After the cells were incubated with various concentrations of CS-AuNR-Pt NSs at 37 °C for 24 hours, the cells were exposed to the laser at 808 nm (5 mm beam diameter) with different power densities for 3 min or not. Then, the plate was further incubated for 24 hours. After that, 20 μL of MTT solution (10 mg mL−1) was added and the plate was incubated for another 4 h, allowing the viable cells to reduce the yellow MTT to dark-blue formazan crystals. Finally, all medium was removed and the crystals were completely dissolved in 150 μL dimethyl sulphoxide (DMSO). The absorbance of each well was measured at 570 nm by a microplate reader (DG-5031, Leidu Corporation, Shengzhen). The reported data represented the means of triplicate measurements. In addition, the cell viability was also qualitatively determined from the color of the cells stained with trypan blue (0.4%) by Olympus system microscope (BX51, Olympus, Tokyo).
In vivo antitumor effect
5 × 105 murine H22 cells in 0.1 mL saline were subcutaneously inoculated into the right limb armpits of every ICR male mice. When the tumor size reached to mean volume of ∼100 mm3, the mice were randomly divided into six groups with eight mice each group. Two groups of tumor-bearing mice were IV injected with saline and free cisplatin (3 mg kg−1), respectively. Next two groups were IV. administered with CS-AuNR NSs (50 mg kg−1, 7.5 mg AuNR eq.), and final two groups were IV administered with CS-AuNR-Pt NSs (50 mg kg−1, 7.5 mg AuNR eq., 1.5 mg kg−1 cisplatin eq.). Among them, one group received CS-AuNR NSs and one group received CS-AuNR-Pt NSs were followed by NIR irradiation (808 nm, 2 W cm−2, 10 min) at 8 h post-injection. The mice were anaesthetised by chloral hydrate solution (5%, 0.1 mL per mice) and the tumor surface was depilated. The tumor area was covered by the 1 cm2 beam irradiation. This day was set as Day 1 and tumor diameters were measured in two dimensions every other day using a vernier caliper for the following 18 days. Tumor volume was calculated as V = d2 × D/2 (where d was the tumor measurement at the shortest dimension, and D was the longest dimension) and the body weight of each mouse was recorded. In the meanwhile, the survival rate was also investigated during 60-day observation period.
In vivo toxicity
Healthy ICR mice were randomly divided into 3 groups with five mice each group and administered with saline, AuNR (7.5 mg kg−1) and CS-AuNR NSs (50 mg kg−1, 7.5 mg AuNR eq.) through tail veins, respectively. 18 days later, their blood biochemical parameters were assayed to assess possible toxicity. Blood samples were collected from the ocular vein (about 0.8–1 mL each mouse) and serum was collected by centrifuging the whole blood at 3 krpm for 15 min. White blood cell count (WBC), red blood cell count (RBC), lymphocyte (LY), granulocyte (GR), monocyte (MO), hemoglobin (HGB), mean corpuscular volume (MCV), mean corpuscular hemoglobin (MCH), mean corpuscular hemoglobin concentration (MCHC), and mean platelet volume (MPV), alanine aminotransferase (ALT), aspartate aminotransferase (AST), total bilirubin (TBILI), blood urea nitrogen (BUN) and creatinine (CRE) were tested.
Immunofluorescence staining and in vivo apoptosis imaging
Mice bearing the tumors were injected in the tail vein with saline, CS-AuNR NSs (50 mg kg−1) and CS-AuNR-Pt NSs (50 mg kg−1), and irradiated under the 808 nm laser for 10 min at 8 h post-injection. After 3 days, the tumors were excised and the specimens were formalin-fixed, paraffin-embedded, and cut into 4 μm-thick sections for proliferating cell nuclear antigen (PCNA) and terminal deoxynucleotidyl transferase-mediated dUTP-biotin nick-end labeling (TUNEL) assays. The PCNA/TUNEL-labeling index was calculated as the number of positive hepatocytic nuclei by the total number of hepatocytic nuclei under ×200 magnification in at least five randomly selected fields.
For live-body apoptotic imaging, apoptotic agent Annexin-Vivo™ 750 (ex: 755 nm, em: 772 nm) was used. 100 μL Annexin-Vivo solution (25 mM Hepes/NaOH, 140 mM NaCl, pH 7.4) was intravenously injected into the mice at 24 h after photothermal therapy combined with chemotherapy. The image was taken 2 hours after administering annexin, using the Maestro in vivo fluorescence imaging system (CRi Inc. USA). For analysis, images were loaded together to normalize all images to one color scale. A region of interest around the tumor was drawn, and quantified as the relative radiance flux ratio by the whole body.
Results and discussion
Preparation of CS-AuNR NSs
The CS-AuNR NSs were prepared by nonsolvent-aided counterion complexation between cationic CS and anionic EDTA in the presence of gold nanorods in aqueous solution, as shown Fig. 1a. Purified gold nanorods were first dispersed in the solution of CS and EDTA, then ethanol was added to the mixture as a nonsolvent. This resulted in the formation of AuNR-encapsulated CS-EDTA nanospheres.25 Subsequently, the CS moiety of the nanospheres was cross-linked by glutaraldehyde and the nanospheres were then dialyzed against weak basic aqueous solution to remove EDTA and ethanol; thereby AuNR-encapsulated CS nanospheres, CS-AuNR NSs, were obtained. Fig. 1b shows the TEM image of CS-AuNR NSs. It can be seen that CS-AuNR NSs have a spherical morphology and uniform size (about 90 nm). Observably, all of the gold nanorods are encapsulated into the interior of CS nanospheres. Also, the overwhelming majority of CS nanospheres contain a single gold nanorod in their interior. Based on the TG analysis, the encapsulation efficiency is 84%, and the loading content of gold nanorods in the nanospheres is 15%.
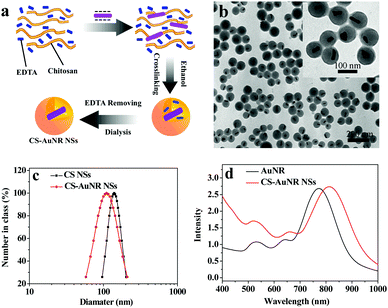 |
| Fig. 1 (a) Scheme of formation of CS-AuNR NSs; (b) TEM image of CS-AuNR NSs; (c) size distribution of CS NSs and CS-AuNR NSs; (d) UV-Vis absorption spectra of AuNR and CS-AuNR NSs. | |
The size distributions of AuNR-encapsulated and non-encapsulated CS nanospheres in aqueous solution were measured by DLS. It can be seen that CS-AuNR NSs have a slightly smaller size than empty CS NSs, with hydrodynamic diameters of 120 nm and 135 nm, respectively (Fig. 1c), and both samples have a narrow size distribution. This is due to the existence of AuNR, which acts as a nucleation center in the formation of CS-AuNR NSs and makes the number of nanospheres more than that without AuNR. Thus, a smaller amount of CS is distributed to each nanosphere, resulting in the hybrid nanospheres of a smaller size. In addition, both CS NSs and CS-AuNR NSs have a positively charged surface with a zeta potential of about 26 mV.
The gold nanorods used here are 10 nm in width and 39 nm in length with an aspect ratio of about 3.9 (Fig. S1a, ESI†), showing a natural longitudinal absorption band at around 770 nm (Fig. 1d). However, after encapsulation by CS nanospheres, the longitudinal absorption band of the gold nanorod in CS-AuNR NSs shifts to 810 nm because of the higher dielectric constant of the surrounding chitosan matrix.30 Therefore, CS nanospheres well preserve the optical properties of gold nanorods. Moreover, the storage stability of CS-AuNR NSs is also improved in DMEM and blood serum (Table S1, ESI†).
In vivo biodistribution
Before carrying out photothermal therapy in vivo, we needed to know the time-dependent localization and accumulation of CS-AuNR NSs in tumor bearing mice. To this end, a near infrared dye, NIR797, was labeled to CS-AuNR NSs, and near-infrared in vivo fluorescence imaging was performed. Fig. 2a shows the time-dependent NIR images of tumor-bearing mice after injection of NIR797 labeled CS-AuNR NSs. The different fluorescence intensities are represented by different colors, as shown in the color histogram. It can be seen that the strong fluorescence signals are observed in the liver and intestine in the initial 4 h post-injection, suggesting that partial CS-AuNR NSs accumulate in these organs and are rapidly captured by the phagocytic cells and the reticuloendothelial system (RES). After 8 h post-injection, the fluorescence signal of the CS-AuNR NSs appears in the tumor site and becomes stronger and stronger as time passes. At 24 h post-injection, the fluorescence intensity in the tumor is already comparable with that in the liver. At 96 h post-injection, the mice were sacrificed and all tissues were imaged (Fig. 2b). It is apparent that a relatively high concentration of CS-AuNR NSs exists in the liver and tumor and less in the kidneys at 96 h post-injection. Also, it can be seen that there are no CS-AuNR NSs in the spleen, heart, lung, stomach, brain and intestines at 96 h post-injection. Furthermore, TEM observation also confirms the accumulation of CS-AuNR NSs in subcutaneous tumors in mice (Fig. S2, ESI†).
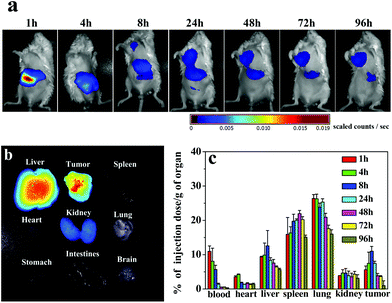 |
| Fig. 2 (a) NIR images of tumor bearing mice following IV injection of NIR797 labeled CS-AuNR NSs; (b) fluorescence imaging of various organs at 96 h post-injection; (c) biodistribution of CS-AuNR NSs in tumor bearing mice. The unit was a percentage of injection dose per gram organ (% ID per g) versus time post-injection. | |
To quantify the biodistribution of CS-AuNR NSs in vivo, the gold content in tissues was determined by ICP-MS after injecting CS-AuNR NSs into tumor-bearing mice. The distribution profiles of CS-AuNR NSs in various tissues at different time points are displayed in Fig. 2c. The data are expressed as a percentage of Au injection dose per unit mass of tissue. As shown in Fig. 2c, CS-AuNR NSs are rapidly cleared from the blood after injection and mainly accumulate in the liver, spleen, and lung of the reticuloendothelial system. However, it is noted that a fast accumulation of CS-AuNR NSs in the tumor is also displayed. The CS-AuNR NS concentration in the tumor is distinctly high in the range 4–24 h post-injection. The maximal accumulation of gold nanorod in tumor tissue reaches 11% of injection dose per gram of tumor at 8 h after injection, which was much higher than those passively and actively targeting gold nanoparticle systems,31 indicating significant superiority of our CS nanospheres system. More importantly, such an amount of AuNR accumulation at the tumor site could induce sufficient hyperthermia for photothermal therapy.12
Photothermal conversion of CS-AuNR NSs
As gold nanorods can effectively convert NIR light into hyperthermia to locally destroy tumor tissues, CS-AuNR NSs are used as near infrared photothermal agents in cancer therapy for deeper tissue penetration of NIR light. To investigate the hyperthermic potential of CS-AuNR NSs in vivo, tumor bearing mice were intravenously injected with CS-AuNR NSs (50 mg kg−1, 7.5 mg kg−1 Au eq.) and the tumor region was exposed to laser irradiation with a wavelength of 808 nm at a power density of 2 W cm−2 for 10 minutes at 8 h post-injection. The temperature at the tumor site was graphically monitored by an infrared thermal camera during irradiation, as shown in Fig. 3a, and the average temperature of the tumor region was displayed in Fig. 3b. It can be seen that the CS-AuNR NSs-accumulated tumor shows a more significant increase in temperature than the corresponding saline-injected one under identical conditions. The highest temperature at the irradiation center of the tumor reaches 59.4 °C and 48.2 °C for the groups receiving CS-AuNR NSs and saline, respectively. The average temperature of the tumor for the CS-AuNR NSs administered group changes from 28.2 °C to 49.0 °C after irradiation, which is sufficient for heat-induced cancer cell death.32 Moreover, the increase in temperature is rapid during the first 2 min and then becomes slow. In contrast, the average temperature of the tumor for the group receiving saline only reaches 41.9 °C after irradiation. This result suggests that CS-AuNR NSs can be an ideal photothermal converter in cancer therapy.
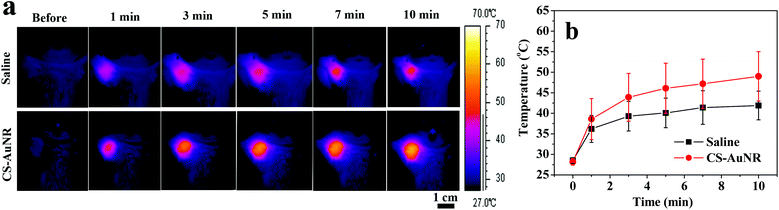 |
| Fig. 3 (a) Infrared thermal images of the tumor in tumor bearing mice at different NIR laser irradiation times. The irradiation was performed at 8 h following IV injection of saline or CS-AuNR NSs; (b) average temperature of the entire tumor region according to the infrared thermal images. | |
In vitro irradiation-induced cytotoxicity
Cisplatin, a chemotherapeutic agent, was reported to have hyperthermia-enhanced cytotoxicity.24 Accordingly, cisplatin was loaded into the CS-AuNR hybrid nanospheres (CS-AuNR-Pt NSs) with a drug loading content of 3% (w/w). The drug release profiles from the nanospheres at 37 °C and 50 °C in PBS (pH 7.4) are shown in Fig. S3.† At 37 °C, an initial release of about 60% of the total loaded drug in the first 10 hours is observed, and about 80% of the loaded drug is released in 120 hours. In addition, an elevation in temperature (50 °C) does not have much effect on the release rate of cisplatin from the nanospheres. In the control experiment (free cisplatin in a dialysis bag), almost 90% of the free cisplatin is released into the medium within 4 h (data not shown).
To assess the in vitro irradiation-induced cytotoxicity of gold nanorods and the synergistic effect with cisplatin, an MTT assay was carried out using the HeLa cell line incubated with various concentrations of CS-AuNR NSs and CS-AuNR-Pt NSs under NIR irradiation at 2 W cm−2, 5 W cm−2 and 10 W cm−2. Both dose-dependent and irradiation-dependent cytotoxicity were observed. In Fig. 4a, the un-irradiated cells did not show any cytotoxicity at various concentrations of CS-AuNR NSs. For the cells irradiated at an energy density of 2 W cm−2, a negligible cytotoxicity was displayed. However, when the energy density of NIR irradiation increased to 5–10 W cm−2, and the concentration of Au was higher than 40 μg mL−1, a significant cytotoxicity was produced. This result suggested that the blank CS-AuNR NSs without irradiation were biocompatible and could be used to kill cancer cells selectively under NIR irradiation. In the case of CS-AuNR-Pt NSs in Fig. 4b, an enhanced cytotoxicity was observed along with the dose and NIR irradiation compared with CS-AuNR NSs, due to the existence of cisplatin. For example, at an Au concentration of 20 μg mL−1, the cells co-incubated with CS-AuNR NSs after irradiation (NIR 5 W cm−2) showed only a 24.2% inhibition rate, while in the case of CS-AuNR-Pt NSs, the inhibition rate of irradiated cells reached 59.2%. Meanwhile, the inhibition rate of un-irradiated cells co-incubated with CS-AuNR-Pt NSs at an Au concentration of 20 μg mL−1 was 19.1%. These results indicated that the significantly synergistic effect of irradiation-induced hyperthermia and cisplatin caused higher cell inhibition than the conventional drug delivery system (CS-Au-Pt NSs with no NIR) or hyperthermia alone. Fig. 4c displays the calculated IC50 values of CS-AuNR NSs and CS-AuNR-Pt NSs under different conditions. At low NIR irradiation (lower than 2 W cm−2), limited hyperthermia for CS-AuNR NSs could not make sense for cell inhibition. Only at strong NIR irradiation (higher than 5 W cm−2), a sufficient cell inhibition or low IC50 value is observed. However, in the case of CS-AuNR-Pt NSs, independently of irradiation or non-irradiation, a low IC50 value is shown. At strong NIR irradiation (5 or 10 W cm−2), CS-AuNR-Pt NSs show lower IC50 values. Compared to that of their non-drug-loading counterparts, about a one-second decrease in IC50 value is observed.
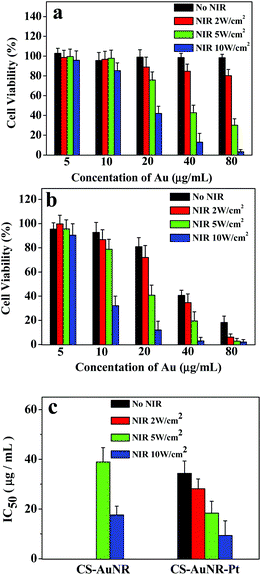 |
| Fig. 4 (a) and (b) Cell viability of HeLa cell line after co-incubation with CS-AuNR NSs and CS-AuNR-Pt NSs at different concentrations and irradiation by NIR light with varying energy densities, respectively; (c) IC50 of CS-AuNR NSs and CS-AuNR-Pt NSs against HeLa cell line under NIR irradiation. The results represent the mean ± SD (n = 3). | |
In an additional test, an increasing number of dead cells stained by trypan blue for CS-AuNR NSs and CS-AuNR-Pt NSs after NIR irradiation are observed in Fig. S4,† providing an in vitro demonstration of the photothermal therapy application of CS-AuNR NSs and the synergistic effect of CS-AuNR-Pt NSs.
In vivo antitumor effect
To assess the therapeutic effect of CS-AuNR-Pt NSs on the combined photothermal ablation and chemotherapy for solid tumors, the in vivo antitumor examinations were carried out using H22 tumor bearing mice as the animal model. The test was designed in six groups, including cisplatin-loaded CS-AuNR NSs with NIR irradiation (CS-AuNR-Pt (+)), cisplatin-loaded CS-AuNR NSs without irradiation (CS-AuNR-Pt (−)), CS-AuNR NSs with irradiation (CS-AuNR (+)), CS-AuNR NSs without irradiation (CS-AuNR (−)), free cisplatin without irradiation (free Pt (−)), and saline with irradiation (saline (+)). The administration dose was 7.5 mg kg−1 on an AuNR basis and 1.5 mg kg−1 on a cisplatin basis.
Fig. 5 shows the tumor volume and survival rates of mice that received different treatments. It is notable that the saline (+) and CS-AuNR (−) groups show an uninhibited tumor growth with no statistical difference. The average tumor volume almost reaches 6 × 103 mm3 on day 18, and their life spans are no more than 32 days (Fig. 5b), showing the considerably fast tumor growth rate. The free Pt (−) group performs an antitumor behavior to some extent. The tumor size is about 50% smaller than that of the saline or CS-AuNR (−) treated groups. The CS-AuNR-Pt (−) group displays a slightly better antitumor efficiency than the free cisplatin (−) group, reducing the tumor volume to below 2 × 103 mm3. On the other hand, a significant photothermal therapeutic effect on the ablation of H22 tumors is observed in the CS-AuNR (+) group. The tumor size experiences a growth stoppage at the initial stage and a slight growth to 1 × 103 mm3 on day 18. In detail, six mice out of eight show tumor recurrence to different extents on day 11, while the remaining two mice have no tumor recurrence during the whole test. The life time of the mice in the CS-AuNR (+) group increases to 59 days, nearly double that of the saline treated group. More noticeably, in the case of the CS-AuNR-Pt (+) group, the tumor growth almost completely stops. In fact, during the first 18-day observation, none of the eight mice have visible tumor or serious tumor recurrence. But only three mice in this group survive more than 60 days while five others die. The reason for death might be from tumor recurrence or toxicity of AuNR. Therefore, this group shows the highest antitumor efficacy over other formulations. These results demonstrate that chemo-photothermal combined treatment based on CS-AuNR-Pt NSs is superior to chemotherapy or photothermal treatment alone. This synergistic effect is ascribed to the enhanced cytotoxicity of cisplatin at elevated temperatures. The body weight of the treated mice is also monitored, since high toxicity usually leads to weight loss (Fig. S5, ESI†). No obvious weight loss is observed over the test time, except for the free Pt (−) group, implying that the toxicity of treatments based on CS-AuNR and CS-AuNR-Pt is not severe. To further assess the toxicity in vivo, hematology analysis and a blood biochemical assay of the mice that received AuNR and CS-AuNR NSs were carried out (Table S2, ESI†). A decrease in MO and TBIL in the AuNR group is observed, suggesting some hematological toxicity. Also, no statistically significant difference among AuNR and CS-AuNR groups is observed in other blood biochemical parameters.
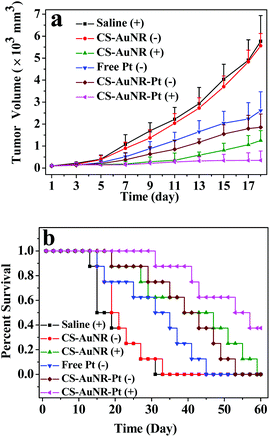 |
| Fig. 5 (a) Tumor volume of H22 tumor bearing mice that received different treatments as indicated. Data are presented as mean ± SD (n = 8); (b) survival curves of H22 tumor bearing mice received different treatments. | |
Apoptosis imaging in vivo
Hyperthermia induced cell death and apoptosis are the main reasons for tumor ablation. To examine the tumor apoptosis/proliferation lever, tumor tissue slips of the mice with different treatments were stained with the TUNEL/PCNA agents on day 3 after treatment (Fig. 6a). It can be seen that only a small number of apoptotic cells are detected in the tumors of the mice receiving saline and NIR irradiation, whereas a significantly large number of apoptotic cells are detected in the tumors of the mice receiving CS-AuNR (+) and CS-AuNR-Pt (+) treatments. In contrast, a high proliferation level is observed in saline-treated and NIR-treated tumors. Meanwhile, the apoptotic cells in tumor sections are counted to record the apoptosis index. As shown in Fig. 6b, the apoptosis index of the samples receiving various treatments displays a huge difference. The treatments with CS-AuNR (+) and CS-AuNR-Pt (+) result in an apoptosis index of 85.2% and 90.1%, respectively, which are much higher than that of the saline and NIR irradiation groups. Furthermore, the treatment with CS-AuNR-Pt (+) leads to more apoptotic cells than that with CS-AuNR (+), indicating the synergistic effect again. On the other hand, the proliferation index is displayed in a contrary manner, that is the proliferation index of the saline or NIR group is much higher than that of the CS-AuNR (+) or CS-AuNR-Pt (+) groups. Also, the cytotoxicity brought by the synergistic effect results in a slight reduction in the proliferation.
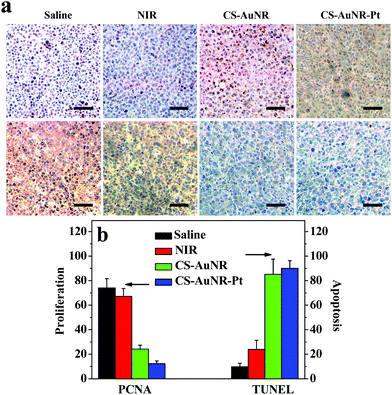 |
| Fig. 6 (a) TUNEL (up) and PCNA (bottom) stain of H22 tumor tissue slips that received different treatments as indicated. Scale bar = 25 μm. (b) The apoptosis and proliferation index calculated as the number of positive hepatocytic nuclei divided by the total number of hepatocytic nuclei in at least five randomly selected fields. | |
To observe the synergistic effect of chemo-photothermal induced apoptosis in vivo, we used an apoptotic imaging agent, Annexin-Vivo 750, to rapidly assess the real-time biological processes based on non-invasive in vivo fluorescence imaging. Annexin-Vivo 750 (λex = 755 nm and λem = 774 nm) is a targeted fluorescence imaging agent comprising a selective protein, Annexin A5, and a near-infrared fluorochrome, and is able to bind to apoptotic cells to reveal the information about immediate cell death. Annexin-Vivo 750 was administrated into the H22 tumor-bearing mouse at 24 h after various treatments. Fig. 7a shows the fluorescence images of the mouse at 2 h post annexin administration. It can be seen that the intensity of the fluorescence signal at the tumor site of various groups shows a significant difference, indicating the differences in apoptosis level in the tumors for various groups. To quantify the relative extent of apoptotic cells in the tumor, the fluorescent signal of each animal is normalized by using the ratio of signal intensity in the tumor to that in the body (Fig. 7b). The apoptotic cell magnitude in the tumors of mice receiving various treatments is in the order CS-AuNR-Pt (+) group > CS-AuNR (+) group > irradiation alone groups > saline group. The enhancement of signal intensity in the tumor can be attributed to an increase in annexin binding at the tumor site. Thus, a synergistic effect resulting from the combined chemotherapy and photothermal ablation is observed in the apoptosis imaging in vivo.
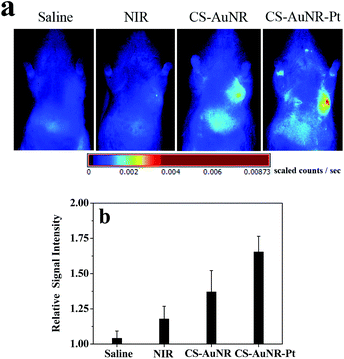 |
| Fig. 7 (a) Fluorescence images of H22 tumor bearing mice treated with different formulations after IV injection Annexin-Vivo 750. (b) The apoptosis index of the tumor quantified as the relative radiance flux ratio. | |
Conclusion
CS-AuNR-Pt NSs, which integrated gold nanorods and cisplatin into chitosan nanospheres, were successfully prepared for the combination of NIR photothermal therapy and chemotherapy for cancer treatment. A sufficient gold nanorod accumulation at the tumor site (about 11% injection dose g−1) and a remarkable temperature rise in the tumor (up to 49 °C on average) upon NIR irradiation were achieved via the hybrid chitosan nanospheres. In vitro cytotoxicity and in vivo antitumor efficiency examinations showed that the local hyperthermia generated by AuNR under NIR irradiation could selectively destroy tumor cells and tissues, and, in turn, enhance the cytotoxicity of cisplatin at elevated temperatures, leading to the achievement of multiple tumor suppression and complete tumor destruction. Non-invasive apoptosis imaging in vivo further demonstrated the synergistic effect of photothermal ablation and chemotherapy in cancer treatment. This multifunctional therapeutic system should hold significant promise in the development of a new generation of cancer treatment systems.
Acknowledgements
This study was supported by NSFC (No. 51033002, 51273090).
Notes and references
- X. H. Huang, P. K. Jain, I. H. El-Sayed and M. A. El-Sayed, Lasers Med. Sci., 2008, 23, 217 CrossRef.
- E. B. Dickerson, E. C. Dreaden, X. H. Huang, I. H. El-Sayed, H. H. Chu, S. Pushpanketh, J. F. McDonald and M. A. El-Sayed, Cancer Lett., 2008, 269, 57 CrossRef CAS.
- L. R. Hirsch, R. J. Stafford, J. A. Bankson, S. R. Sershen, B. Rivera, R. E. Price, J. D. Hazle, N. J. Halas and J. L. West, Proc. Natl. Acad. Sci. U. S. A., 2003, 100, 13549 CrossRef CAS.
- P. Diagaradjane, A. Shetty, J. C. Wang, A. M. Elliott, J. Schwartz, S. Shentu, H. C. Park, A. Deorukhkar, R. J. Stafford, S. H. Cho, J. W. Tunnell, J. D. Hazle and S. Krishnan, Nano Lett., 2008, 8, 1492 CrossRef CAS.
- J. Y. Chen, C. Glaus, R. Laforest, Q. Zhang, M. X. Yang, M. Gidding, M. J. Welch and Y. N. Xia, Small, 2010, 6, 811 CrossRef CAS.
- J. Y. Chen, M. X. Yang, Q. A. Zhang, E. C. Cho, C. M. Cobley, C. Kim, C. Glaus, L. H. V. Wang, M. J. Welch and Y. N. Xia, Adv. Funct. Mater., 2010, 20, 3684 CrossRef CAS.
- X. H. Huang, I. H. El-Sayed, W. Qian and M. A. El-Sayed, J. Am. Chem. Soc., 2006, 128, 2115 CrossRef CAS.
- Y. F. Huang, K. Sefah, S. Bamrungsap, H. T. Chang and W. Tan, Langmuir, 2008, 24, 11860 CrossRef CAS.
- X. W. Liu, H. Q. Tao, K. Yang, S. A. Zhang, S. T. Lee and Z. A. Liu, Biomaterials, 2011, 32, 144 CrossRef.
- J. T. Robinson, K. Welsher, S. M. Tabakman, S. P. Sherlock, H. L. Wang, R. Luong and H. J. Dai, Nano Res., 2010, 3, 779 CrossRef CAS.
- K. Yang, S. A. Zhang, G. X. Zhang, X. M. Sun, S. T. Lee and Z. A. Liu, Nano Lett., 2010, 10, 3318 CrossRef CAS.
- W. I. Choi, J. Y. Kim, C. Kang, C. C. Byeon, Y. H. Kim and G. Tee, ACS Nano, 2011, 5, 1995 CrossRef CAS.
- G. von Maltzahn, J. H. Park, A. Agrawal, N. K. Bandaru, S. K. Das, M. J. Sailor and S. N. Bhatia, Cancer Res., 2009, 69, 3892 CrossRef CAS.
- A. M. Alkilany, L. B. Thompson, S. P. Boulos, P. N. Sisco and C. J. Murphy, Adv. Drug Delivery Rev., 2012, 64, 190 CrossRef CAS.
- M. Ravera, G. Bagni, M. Mascini, J. C. Dabrowiak and D. Osella, J. Inorg. Biochem., 2007, 101, 1023 CrossRef CAS.
- W. T. Wu, J. Shen, P. Banerjee and S. Q. Zhou, Biomaterials, 2010, 31, 7555 CrossRef CAS.
- Y. Kawai, T. Nakao, N. Kunimura, Y. Kohda and M. Gemba, J. Pharmacol. Sci., 2006, 100, 65 CrossRef CAS.
- S. M. Lee, H. Park and K. H. Yoo, Adv. Mater., 2010, 22(36), 4049 CrossRef CAS.
- J. H. Park, G. von Maltzahn, M. J. Xu, V. Fogal, V. R. Kotamraju, E. Ruoslahti, S. N. Bhatia and M. J. Sailor, Proc. Natl. Acad. Sci. U. S. A., 2010, 107, 981 CrossRef CAS.
- H. Y. Liu, D. Chen, L. L. Li, T. L. Liu, L. F. Tan, X. L. Wu and F. Q. Tang, Angew. Chem., Int. Ed., 2011, 50, 891 CrossRef CAS.
- A. Agarwal, M. A. Mackey, M. A. El-Sayed and R. V. Bellamkonda, ACS Nano, 2011, 5, 4919 CrossRef CAS.
- J. Yang, J. Lee, J. Kang, S. J. Oh, H. J. Ko, J. H. Son, K. Lee, J. S. Suh, Y. M. Huh and S. Haam, Adv. Mater., 2009, 21, 4339 CrossRef CAS.
- T. S. Hauck, T. L. Jennings, T. Yatsenko, J. C. Kumaradas and W. C. W. Chan, Adv. Mater., 2008, 20, 3832 CrossRef CAS.
- M. Urano, M. Kuroda and Y. Nishimura, Int. J. Hyperthermia, 1999, 15, 79 CrossRef CAS.
- R. Guo, L. Y. Zhang, H. Q. Qian, R. T. Li, X. Q. Jiang and B. R. Liu, Langmuir, 2010, 26, 5428 CrossRef CAS.
- X. H. Huang, S. Neretina and M. A. El-Sayed, Adv. Mater., 2009, 21, 4880 CrossRef CAS.
- S. Ohno, Z. H. Siddik, Y. Kido, L. A. Zwelling and J. M. C. Bull, Cancer Chemother. Pharmacol., 1994, 34(4), 302 CrossRef CAS.
- A. Gole and C. J. Murphy, Chem. Mater., 2004, 16, 3633 CrossRef CAS.
- X. L. Yan and R. A. Gemeinhart, J. Controlled Release, 2005, 106, 198 CrossRef CAS.
- L. M. Liz-Marzan, M. Giersig and P. Mulvaney, Langmuir, 1996, 12, 4329 CrossRef CAS.
- M. A. Phillips, M. L. Gran and N. A. Peppas, Nano Today, 2010, 5, 143 CrossRef CAS.
- X. H. Huang, P. K. Jain, I. H. El-Sayed and M. A. El-Sayed, Photochem. Photobiol., 2006, 82, 412 CrossRef CAS.
Footnote |
† Electronic supplementary information (ESI) available. See DOI: 10.1039/c2bm00138a |
|
This journal is © The Royal Society of Chemistry 2013 |
Click here to see how this site uses Cookies. View our privacy policy here.