DOI:
10.1039/C2RA21085A
(Review Article)
RSC Adv., 2012,
2, 10110-10124
Three-dimensional fibrous scaffolds with microstructures and nanotextures for tissue engineering
Received
30th May 2012
, Accepted 16th August 2012
First published on 17th August 2012
Abstract
Much effort has been dedicated to developing scaffolds that can mimic native microenvironments to promote tissue regeneration. A natural tissue scaffold provides not only a three-dimensional (3-D) structural support but also nanotextured surfaces comprising of a fibrous network for cell adhesion and signaling. In addition to its function as a structural template, the scaffold also increases cell–cell and cell–matrix interactions, which in turn directs cell proliferation and differentiation. Microfabrication techniques can create 3-D scaffolds with microporous structures, which are important to cell infiltration and nutrients transport. Nanofabrication techniques can be used to create surfaces with desirable chemistry and nanotopography, which has led to remarkable findings on how surfaces, through their nanoscale features, affect cellular behaviors. Tissue regeneration requires 3-D scaffolds with both microporous structures and nanotextured surfaces. However, scaffolds created by microfabrication usually lack a nanotextured surface, while nanotextured scaffolds generated from nanofabrication lack a 3-D microenvironment. Recent research in tissue engineering has paid great attention to combining these two scaffold features and developing novel methods for their fabrication. In this review paper, we first give a brief introduction on the influence of 3-D microstructures and nanotopographies on cellular functions, including cell adhesion, proliferation, morphogenesis and differentiation. Recent development of fabrication methods to produce 3-D fibrous scaffolds with microporous structures and nanotextures is then discussed with some examples of their applications.
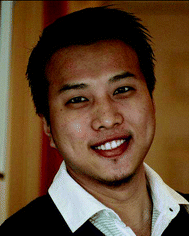 Robin Ng | Robin Ng received his PhD in Chemical and Biomolecular Engineering from The Ohio State University, focusing on three-dimensional cell culture and process development. Robin recently joined Life Technologies where he builds technical credibility for the company’s BioProduction cell culture products and service business. Previously, he worked at Shire Human Genetic Therapies and Goodwin Biotechnology, Inc. leading several cell culture process development projects. Robin currently serves on the advisory board of the Society for Biological Engineering and as a scientific advisor for Bioprocessing Summit conference. |
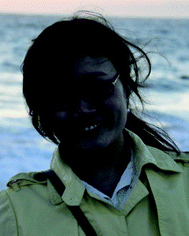 Ru Zang | Ru Zang obtained her BS and MS in Chemical Engineering in China and recently graduated from The Ohio State University with a PhD in Chemical and Biomolecular Engineering under the supervision of Dr S. T. Yang. She is currently a postdoctoral research scientist at The Ohio State University working on the development of microbioreactor platforms for cell-based high throughput screening (HTS) for drug discovery. Her research interests also include scale-down HTS platforms for bioprocess development, nanoengineered scaffolds for nerve tissue engineering, and embryotoxicity and cytotoxicity assay development. |
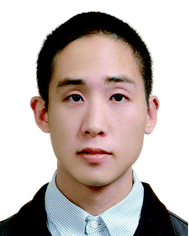 Kevin K. Yang | Kevin Yang graduated from The Ohio State University in 2011 with a BS in Chemical and Biomolecular Engineering and a minor in music. While at OSU, he worked in Dr Michael Paulaitis' research group. Kevin is currently a Teach for America (TFA) corps member and is teaching math and physics at Green Dot’s Animo Inglewood Charter High School. After his two-year commitment to TFA, Kevin plans to attend the California Institute of Technology to pursue his PhD in Chemical Engineering. |
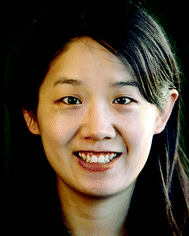 Ning Liu | Ning Liu is a senior scientist at Irvine Scientific. She is experienced in bioreactor process and cell culture medium development and optimization, human embryonic and induced pluripotent stem cell technology, in-process assays, fermentation, and design of experiment. Her current responsibility is focused on the new product development in cell culture media for industrial bioprocess and cell therapy. Prior to her current employment, she held positions in Cellular Dynamics International and Bioprocessing Innovative Company. She holds a PhD in Chemical Engineering from The Ohio State University, and BS in Chemical Engineering and MS in Biochemical Engineering from Zhejiang University in China. |
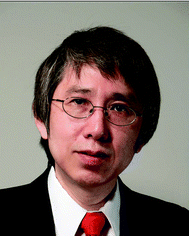 Shang-Tian Yang | S. T. Yang received his PhD in Biochemical Engineering from Purdue University and currently is a professor at The Ohio State University and the director of Ohio Bioprocessing Research Consortium. His research involves metabolic engineering, cell and tissue engineering, and microbioreactors for high-throughput screening and biodiagnostics. He has more than 200 publications and 16 patents. He is an elected fellow of American Institute of Medical and Biological Engineering, Associate Editor for the journal Process Biochemistry, and past chair of Division 15 Food, Pharmaceutical and Bioengineering of American Institute of Chemical Engineers (AIChE). |
1. Introduction
Tissue engineering has emerged as a multidisciplinary field encompassing chemical engineering, material science, chemistry and biology; it empowers the improvement of the health and quality of life by restoring, maintaining, and enhancing tissue and organ functions.1,2 The goal of tissue engineering is to construct functional substitutes for tissues and organs that may afford a permanent solution to damaged organs or tissues without relying on supplementary therapies. Cells and scaffolds are the two major components of tissue engineering. Cells isolated during a biopsy typically grow in a biomimetic scaffold under controlled conditions to self-regenerate, remodel, and adapt.3 Scaffolds, which are used to facilitate cell adhesion, proliferation, extracellular matrix (ECM) production, morphogenesis and differentiation, must mimic in vivo microenvironments and offer chemical and physical cues to regulate cellular functions. In general, 3-D scaffolds can provide a better link between single cells and organs than conventional 2-D cultures because they offer spatial cell organization.4,5 3-D scaffolds can be divided into four major categories: amorphous foam-like scaffolds, gel-like scaffolds, fibrous scaffolds and scaffolds with controlled geometry and structures. Each type of scaffold offers its own advantages and disadvantages in mimicking the organization of native tissue structures. Considerations of scaffold design include the shape of the original tissue, mechanical properties, ability to direct cell–matrix and cell–cell interactions, and porous structures for efficient mass transport.3 Fibrous scaffolds, in particular, are favorable as a replacement for natural scaffolds because of their high porosities (up to 95%), isotropic structures, and homogeneous fiber size and pore distribution.6–8
3-D fibrous scaffolds can support cell adhesion and proliferation, but generally lack the topographical features needed to resemble native ECM. Natural ECM proteins, such as laminin and collagen, exhibit nanoscale characteristics.9 Laminin and collagen molecules are structurally bundled together to form fibrils with diameters ranging from 260 to 410 nm.9 These sub-micron fibrils with nanoscale features are important to many cellular functions. Recent research efforts have thus focused on mimicking the nanostructural features of ECM to improve scaffold functionality.3,10–12 Successes in directing cell towards certain cellular morphologies, functions and differentiations have been reported using different types of nanofibers.10 Furthermore, studies have shown that cells cultured in scaffolds with different surface properties, including surface chemistry, geometry and topography behaved differently.9,13,14
Therefore, in order to simulate in vivo microenvironments, the next generation of tissue scaffolds should incorporate both 3-D microporous structures and nanoscale architectures to enhance cellular functions. This review highlights the current state of the art techniques in fabricating 3-D nanoengineered fibrous scaffolds and their effects on cell growth, tissue development and functions. The important effects of microscale and nanoscale structures and surface topography on cellular behaviors are briefly discussed, followed with the recent development of 3-D scaffolds with both microscale structures and nanoscale features.
2. Scaffold effects on cellular behaviors
Cellular behaviors may change depending on the type of culturing methods and scaffold design. For 2-D cultures or cells cultivated on a surface, surface properties including the chemistry, geometry or topography (e.g., roughness, patterning and curvature) and functionalization with biological molecules (e.g., growth factors and adhesion proteins) can affect cell adhesion, migration, proliferation, differentiation and morphogenesis.9,13,14 For 3-D cultures, in addition to the surface properties, which are on the nanoscale or less than 1 μm in scale, many microscale (1–1000 μm) parameters, including porosity, pore size, pore configuration and fiber diameter, and macroscale (>1 mm) parameters, including scaffold dimension and configuration, can affect cell proliferation, differentiation and performance of the engineered tissue for in vivo and in vitro applications.5–8 In addition, the material properties of the scaffold such as degradability, ionic charges, mechanical strength and electrical conductivity, may also play an important role in tissue development. However, the effects of these scaffold parameters could vary greatly with cell types and other culture conditions. A brief review on 3-D microstructural and nanostructural effects on cellular behaviors important to tissue engineering is given below.
2.1 3-D microstructural effects
A tissue scaffold should be biocompatible and provide the mechanical and functional support necessary for cell growth and survival. Cells grown in a 3-D scaffold behave differently from those on flat surfaces.5,15,16 The third dimension in the 3-D scaffold provides another direction for cell–cell interactions, cell migration and cell morphogenesis, which are all important in regulating the cell cycle and tissue functions. 3-D scaffolds can support a higher cell density than 2-D cultures.16 In addition, 3-D scaffolds can provide not only the template for cells to adhere and grow, and the interconnectivity within the 3-D construct allows nutrients and metabolites to be transported in and out of the engineered tissue. 3-D microenvironments and interconnectivity are also important for cellular motility and re-organization, which induce proper differentiation and morphogenesis.17 The profound effects of 3-D microstructures have been extensively studied, and are summarized in this section. More in-depth reviews on this topic can be found elsewhere.5,12
2.1.1 Cell morphology, adhesion and spatial organization.
The majority of cells inside a 3-D scaffold attach and spread on the available surface inside the scaffold. However, spatial organization and migration patterns inside the scaffold are also unique when cells are cultured in 3-D scaffolds. In a fibrous scaffold, cells not only attach to the surface of the scaffold, but also form bridges and aggregates between fibers.15,16 Previous studies have revealed that the scaffold architecture, including pore structure and size, affects cell attachment, spreading and spatial organization within the scaffold.18,19 When the space or distance between adjacent surfaces is large, cells only attach and stretch on the 2-D surfaces of the 3-D scaffold. However, when adjacent surfaces are nearby, cell bridging between surfaces occurs. For example, MCF-7 breast cancer cells, with an average cell diameter of 6 μm, would bridge between 60 μm wide ridges, but would not bridge across 120 μm wide ridges (see Fig. 1A).19 In contrast, astrocytes, with an average diameter of 30 μm, could bridge across 120 μm wide ridges (see Fig. 1B).19 This bridging pattern, as opposed to confined surface attachment, was also observed when hepatocytes were cultured inside a polyethylene terephthalate (PET) fibrous scaffold with large pores, within which cells were found to cluster around the fiber surfaces (Fig. 1C). The formation of cell bridges could be due to the mechanical stress transmitted over cell surface receptors, which physically couple the cytoskeleton to the extracellular matrix. Biochemical responses to force-dependent changes in scaffold geometry or molecular mechanics could also influence cellular responses.20
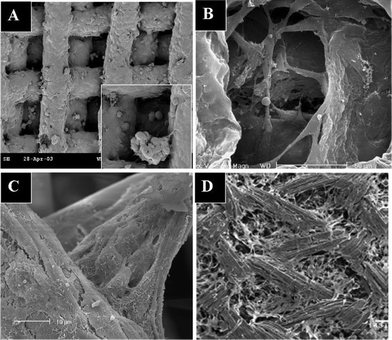 |
| Fig. 1 Scanning electron micrographs of cells cultured in 3-D scaffolds: (A) MCF-7 breast cancer cells covering the surface of and forming aggregates in a PLGA scaffold; (B) astrocytes bridging in a PLGA scaffold; (C) hepatocytes bridging and forming aggregates in a PET scaffold; (D) mesenchymal stem cells growing on collagen fibers in a knitted PLGA–collagen scaffold. (A and B are reproduced from ref. 19, and D is from ref. 21, with permission). | |
In addition, 3-D cultures promote cell–cell interactions and agglomerations. In one study, mesenchymal stem cells were able to attach to and spread between thinner collagen fibers situated between knitted poly(lactic-co-glycolic acid) (PLGA) fibers, filling up all the microporous spaces inside the 3-D scaffold (see Fig. 1D).21 It has also been reported that cells cultured in 3-D scaffolds are more spherical and usually smaller in size than those cultured on flat surfaces.22 This phenomenon can be attributed to a reduction of surface spreading and more efficient production and sharing of ECM among cells.
2.1.2 Cell proliferation, protein production and differentiation.
The distinctive advantages of 3-D cultures over 2-D cultures in cell growth, spatial organization and migration23 lead to differences in cell proliferation,24 cellular function,25 gene expression26 and differentiation.26 Compared to cells on flat surfaces, cell proliferation in 3-D fibrous scaffolds is slower because fewer cells are directly attached to the fiber surfaces.27 Differences in spatial organization and distribution also contribute to the difference in cell growth. The high specific surface area offered by 3-D scaffolds allows for long-term cell culturing in vitro. In addition, compared to 2-D cultures, hybridoma and astrocyte cells in 3-D cultures produced more monoclonal antibody28 and glial cell-derived neurotrophic factor (GDNF),19 respectively. In another study, chondrocytes produced glycosaminoglycan (GAG) more rapidly when cultured in 3-D scaffolds than in 2-D scaffolds.29
Compared to 2-D cultures, more cells are present in the G1/G0 phase in 3-D cultures. The 3-D fibrous matrix used in long-term cultures supported a higher cell density with smaller cells than the 2-D scaffold and selectively retained healthy, non-apoptotic cells.28 Also, distinct cellular interaction patterns and intracellular signaling were observed in 3-D scaffolds.25 These phenomena could be caused by more efficient ECM production and sharing between neighboring cells in 3-D scaffolds, which are not feasible in 2-D scaffolds and the 3-D cellular arrangement inside the scaffolds. The observed difference in the expression of cell adhesion related proteins, such as E-cadherin and vinculin, also demonstrated the difference in functionality of cells cultured in 3-D scaffolds.
The proliferation and differentiation patterns of embryonic stem (ES) cells could also be directed by altering the physical and biochemical properties of 3-D matrices. By culturing mouse ES cell-derived embryoid bodies in various semi-interpenetrating polymer matrices, it was found that endothelial cell differentiation and vascularization were enhanced by the introduction of fibronectin to 3-D collagen scaffolds.30
2.2 Nanostructural effects
In addition to mimicking the 3-D structural microenvironments of native tissues, it is important for the scaffold to incorporate tissue-specific microenvironments in order to properly maintain and regulate cellular behavior and function. In vivo, cells are surrounded by ECM that is characterized by a natural web of hierarchically organized nanofibers. The nanofibers play a vital role in directing cellular behaviors via cell–surface interactions.31 Cell–surface interactions can be influenced by differences in surface chemistry, mechanical properties and topography. Cell differentiation can be directed by controlling the mechanical properties of the surface as well as surface topography. Surfaces with different topographies have been fabricated to mimic the in vivo microenvironments and shown to affect cell attachment.32 ECM provides cells with both biological and mechanical supports, and serves as a cell–cell interaction mediator in the cell adhesion process. Structurally, native ECM comprises various nanoscale fibers and molecules. The nanoscale nature of ECM generally provides two benefits to cells: a high specific surface area and structural/mechanical support. Nanotopographical surfaces may not necessarily be favorable for all cell types. Different cells respond differently to nanosurfaces,33 and some cells are not beneficially affected by the nanotopography. Although extensive studies have been conducted to investigate the influence of nanosurfaces on cellular behaviors and activities,13,14,34,36,37 the underlying mechanisms of how nanofibers or nanotopographical surfaces affect cells are not well understood.35 This section provides a brief review on nanotopographical effects reported in the literature.
2.2.1 Cell morphology, migration and adhesion.
The most reported cellular effects of nanofibrous topography are on cellular attachment and spreading.36 Using osteoblasts as the study model, it was found that the stress fibers formed on the peripheral of the cells changed from dot-like to fiber-like when the cells were cultured on nanotextured surfaces, confirming that the stress fibers were highly activated and more likely to immobilize the cells.38 The formation of stress fibers is also confirmed by the concentration of vinculin on the cytoplasmic side of the cell–substrate surface, indicating the presence of focal contacts.39 Furthermore, as the cells spread on the surface, they flatten and stretch their microfilaments and cytoskeleton.40
Various types of nanofibers have been fabricated and used to increase cell affinity to surfaces.41 Several findings support the proposition that surface topography affects cellular activities and morphology.42,43 Some unique morphologies observed in cells cultured on nanotopographical surfaces are shown in Fig. 2. When cultured on conventional flat surfaces, cells usually are flatly and irregularly spread out on the surface. However, when cultured on a nanofibrous surface, numerous filopodia were entangled with the tips of the nanosurfaces (see Fig. 2A).44 Cells cultured on nanosurfaces also exhibited a more 3-D morphology (Fig. 2B).45
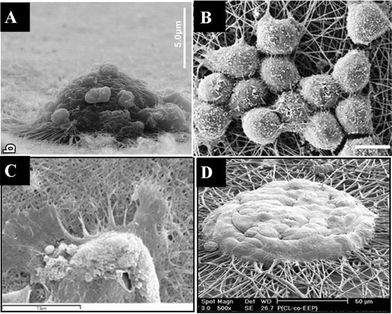 |
| Fig. 2 Scanning electron micrographs of cells cultured on nanofibrous surfaces: (A) osteosarcoma cells spread on a carbon nanotubes (CNTs)-coated surface with many filopodia extended and entangled with the CNTs; (B) rat neural stem cells cultured on polyether sulfone nanofibers with a “stand-up” posture instead of spreading on the surface; (C) keratinocytes on electrospun silk fibroin nanofibers; (D) spheroid-like hepatocytes spread on galactose-grafted nanofibers showing that the aggregates engulfed the functional nanofibers. (Reproduced from ref. 44, 13, 47 and 48, respectively, with permission). | |
Cells cultured on nanotextured surfaces tend to make focal contacts with the tips of the nanotextured surface instead of local contacts, in which most of the cell cytoplasm is in contact with the surface. These focal contacts influence the mechanotransduction process inside the cytoplasm and affect cell attachment and cellular activities. Therefore, cells formed a looser and more orderly layer on nanotextured surfaces than on flat surfaces, and variations in surface channel distribution density and size significantly affected cell attachment and morphology.46 This phenomenon was observed through the difference in the ECM spreading between cells cultured on a 2-D surface and a nanotextured surface (see Fig. 2C).47 Hepatocytes formed large clusters or aggregates on the nanofibers (see Fig. 2D) and showed increased cell–surface interactions, attachment, and albumin synthesis.48 In addition, cells were also shown to align in the direction of the surface nanotopography.14 The differences on cell morphology caused by surface properties may be a result of cell migration. Surface topography may modulate cell migration through contact guidance, as the responses of cells to topographical discontinuities could lead to cell polarization, lamellipodial and filopodial extension, actin bundle alignment and focal adhesion formation preferentially along these surface features.49
Increased cell attachment and growth can be attributed to protein adsorption. Surface energy increased with an increase in the surface roughness, and proteins would be attracted first to favorably adsorb onto the surface before cells can be physically laid on top of it.41 The high surface energy on the ridges of rough surfaces could also assist cell proliferation by improving protein adsorption.50–52 It has been shown that surface roughness and electrostatic interactions between the bone implant and bone can influence cell adhesion and subsequent promotion of bone growth.53 Furthermore, both the amount of ECM proteins available on the surface and the surface roughness (in terms of anchor size and interfiber distances) affected cell spreading.54
The orientation and alignment of brain-related cells, including neurons and astrocytes, are necessary for proper cellular communication and signal transduction. As observed in our studies, astrocytes were elongated in a certain direction and orientation when cultured on nanostructured surfaces.55 Neuron-like cells cultured on nanotextured surfaces also exhibited a substantially higher degree of neurite extension.56 In addition, neurite outgrowth, which usually requires basal proteins, was also demonstrated on top of PLLA (poly-L-lactic acid) nanostructural surfaces.57 These studies demonstrate that nanofeatured scaffolds have profound effects on cell adhesion and morphology critical to nerve regeneration.
2.2.2 Cell proliferation, cell differentiation and protein production.
Changes in cellular attachment and spreading not only dictate the mobility of the cells, but also affect cellular growth, arrangement and ECM production.58 When cultured on surfaces with mono-directional nanofibers, human ligament fibroblast (HLF) cells were more sensitive to strain in the direction of their longitudinal axis, i.e., parallel to fiber orientation.58 In addition, interactions between cells and nanosurfaces led to slower cell growth because of increased cytoskeleton rearrangement and cell immobilization on the surface.59–62 The interaction of cellular cytoskeleton with the surface can influence signal transduction within the cell, which is mediated by the Ca2+ level in the cytoplasm of the cell.63 These changes in intracellular signal transduction can lead to changes in cell proliferation and differentiation.
Nanofibrous scaffolds have been shown to favorably support adipogenic, chondrogenic and osteogenic differentiation in human mesenchymal stem cells.64,65 In addition, nanofibrous scaffolds promoted neuronal differentiation in nerve stem cells and murine neural progenitor cells.66,67 Also, the expression of various proteins, including ECM proteins, proteoglycans and collagen digestible proteins (CDP), in osteoblast-like cells was found to be affected by nanosurfaces.68
3. Fabrication of 3-D fibrous scaffolds with nano- and microstructures
In tissue engineering, it requires a 3-D scaffold with a large surface area and volume to support high cell density and long-term culture, which is also required for most biological studies to observe significant effects.69 Fibrous scaffolds can be fabricated from synthetic polymers to mimic the fibrous structure of collagen and gelatin present in native extracellular matrices.70 The ideal polymeric material should be biocompatible, economical, easy to fabricate, and with appropriate mechanical and biological properties.71 Among the commonly used polymeric materials for scaffold fabrication are PLGA,19 poly(lactic acid) (PLA),72 poly(hydroxyalkanoates) (PHA),73 polycaprolactone (PCL),74 poly(ethylene terephthalate) (PET),75 polysaccharide,76 and poly(ethylene glycol) (PEG).77Table 1 lists some commonly used tissue scaffold materials, both natural and synthetic, and their pros and cons. The direction of tissue scaffold fabrication is leaning towards 3-D scaffolds with nanotextures (resembling extracellular matrices), structural penetration and good mechanical (i.e. strength) and physical properties (i.e. pore size and porosity). Various approaches and methods used to fabricate 3-D fibrous scaffolds and nanotopographical features are discussed in this section.
Table 1 Natural and synthetic polymeric materials commonly used in tissue scaffold constructs and their advantages and disadvantages
Types of scaffold materials |
Comments |
Examples |
Natural materials |
Advantages
|
Alginates |
Low toxicity |
Chitosan |
Low chronic inflammatory response |
Collagen |
Biological recognition and biodegradable |
Fibrin |
|
Fibronectin |
Disadvantages
|
Gelatin |
Complexities associated with purification, immunogenicity and pathogen transmission |
Glycosaminoglycan |
Poor mechanical strength |
Laminin |
Difficult manipulation |
Poly(hydroxyalkanoates) |
Easy denaturation |
Polysaccharides |
Not available in large quantities |
Silk |
Batch-to-batch variations |
|
|
Synthetic materials |
Advantages
|
Polycaprolactone |
Large-scale and reproducible production |
Poly(ethylene glycol) |
Controllable design with desired mechanical properties, geometries and degradation time |
Poly(ethylene terephthalate) |
|
Poly(lactic acid) |
Disadvantages
|
Poly(lactic-co-glycolic acid) |
Lack of cell-recognition signals |
Poly(propylene fumarate) |
|
Polyurethane |
3.1 Methods to fabricate 3-D fibrous scaffolds
Different fabrication techniques can be used to design and optimize distinct fibrous scaffold properties, such as pore size and structure, suitable for culturing the desired types of cells. Most of the scaffolds used in tissue engineering have random pore shapes and sizes that are of the same order as the cell. These pores contain high interconnectivity in order to ensure cell infiltration during seeding, cell interaction and nutrient/waste transport.78 Although the amorphous shape of scaffolds could contribute to the overall mechanical performance of the scaffolds, a scaffold with a defined pore size could help thoroughly explore the effect of pore size on cellular functions in the scaffold.79
Table 2 lists methods for fabricating 3-D scaffolds with different structures and microscale pores. Nonwoven fibrous matrices with micron-sized fibers possess isotropic structure with a high porosity (usually >90%), good mechanical strength and good thermal and chemical stability. In particular, needle-punched nonwoven fibrous matrices have a wide range of pore size distribution. These nonwoven fibrous matrices can be made from both biodegradable (e.g., PGA, PLA and collagen) and non-biodegradable (e.g., PET, polypropylene and polyethylene) polymers. To fabricate fibrous scaffolds with tightly controlled pore sizes, microembossing19 and 3-D printing80 have been developed, but they usually have a relative small porosity of less than 0.8.
Table 2 Fabrication methods for various types of 3-D scaffolds
Fabrication method |
Matrix structure |
Porosity |
Ref. |
Microembossing |
Grid with controlled pores |
0.5−0.8 |
17
|
3-D printing |
Lattice with controlled pores |
0.5 |
77
|
Electrospinning |
Fibrous |
0.7−0.9 |
78
|
Fiber bonding |
Fibrous |
0.81 |
79
|
Needle punch |
Fibrous |
0.93 |
80
|
Phase separation |
Porous |
0.90 |
81
|
Gas foaming |
Spongeous |
0.93 |
82
|
Solid-free fabrication |
Spongeous |
0.9 |
83
|
Particulate leaching |
Spongeous |
0.87 |
84
|
Other methods commonly used in fabricating fibrous matrices include fiber bonding and electrospinning. In fiber bonding, individual unbounded fibers are fabricated and bonded to form scaffolds comprising the network of bonded fibers. Fiber bonding can be done with a thermal treatment81 or treatment with supercritical carbon dioxide.82 In electrospinning, a voltage is applied to a droplet of polymer solution so that the electrostatic repulsion causes a stream of liquid to erupt from the droplet towards a collecting plate. The polymer's solvent evaporates in flight, and the polymer forms fibers on the collecting plate. The fiber size is controlled by controlling the voltage and the distance between the needle and the collecting plate. Although the pore structure is usually amorphous, the porosity of electrospun scaffolds can be easily controlled.83 Due to its practicality and flexibility, electrospinning has also been used to generate nanofibrous materials, which will be further discussed in the next section.
Matrices with spongeous or foam-like structures are also widely used in tissue engineering. They can be produced by phase separation,84 gas foaming,85 solid-free fabrication,86 particulate leaching,87 or particulate leaching combined with overrun processes.88 However, none of these fabrication methods is capable of producing scaffolds with desirable nanoscale features without compromising the 3-D microscale structure.
3.2 Methods to fabricate nanostructured scaffolds
Table 3 lists methods available for fabricating nanotextured surfaces.89 Photolithography, microstereolithography (MSTL) and electron beam lithography (EBL) can create surface nanotopographies with well-ordered and geometrically precise patterns. However, they require expensive equipment and a high level of expertise, and are difficult to scale up. Polymer demixing, colloidal lithography and chemical etching provide inexpensive and scalable methods for creating nanoscale topographies. Although polymer demixing and colloidal lithography can generate nanopits and nanocolumns, they cannot produce specific feature geometries. In general, all aforementioned methods can only introduce nanostructured surface on a flat, planar surface.
Table 3 Nanoscale fabrication methodsa and their feature sizes
Fabrication method |
Feature size |
Comments |
Note: conventional photolithography can only create features at the micrometer scale (>0.5 μm) (modified from ref. 89).
|
Electrospinning |
>30 nm |
Limited to only fiber formation |
Colloidal lithography |
>20 nm |
Uncontrollable geometries |
Micro stereolithography |
330–360 nm |
Controllable geometries and adequate pore size100 |
E-beam lithography |
3–40 nm |
Precise geometry and patterns can be created |
Polymer demixing |
>13 nm |
Limited sample features can be created |
Phase separation |
Pore sizes >1 nm |
Uncontrollable patterns |
Self assembly |
Tailored by molecular design |
Limited to only molecules that will undergo self-assembly |
Chemical etching |
>1 nm |
Uncontrollable geometries |
Nanoporous membrane |
Pore sizes >1 nm |
Lack of mechanical and physical properties |
Nanofibers can recreate an environment that mimics the natural ECM around cells. They can be generated using various methods, including molecular self-assembly, phase separation and electrospinning. Molecular self-assembly can produce highly ordered nanofibrous scaffolds. However, its applications are limited to molecules that can be engineered for self-assembly. Phase separation can be used to create 3-D scaffolds with fibers in the sub-micron range. However, this method can only produce randomly distributed fibers. Electrospinning can generate fibers, from nanometer to micrometer size, with tunable properties including controllable pore size, fiber size, fiber stiffness and matrix turnover.10 It can also create aligned nanofibers on a surface, forming a simple, ordered nanotopography.
Although electrospinning has been widely used to generate nanofibrous materials with an average fiber diameter of less than 0.5 μm,90 the electrospun nanofibrous materials have only pseudo-3-D structures with a relatively low porosity and only nanosized pores. The 3-D nanofibrous structures generated by electrospinning are 3-D relative to the size of the nanofibers, and the pores in these nanofibers are on a scale similar to that of the fibers. With a typical cell size of 10 μm, these nanofibrous substrates are 2-D surfaces to cells. Even though they do have interconnected pores, cell infiltration inside nanofibrous scaffolds is limited by the small pore size and consequently, cells can only grow in a 2-D, rather than 3-D, pattern. For instance, chondrocytes cultured in nonwoven PLLA nanofibers either wrapped around and encapsulated the nanofibers or were suspended among fibers, forming globular-shaped cells.91 A similar phenomenon was also observed using the Ultra-Web™ Synthetic Surface (Corning).67 Furthermore, studies on the differentiation of human mesenchymal stem cells cultured in nanofibrous scaffolds showed that nanofibers deficient in 3-D structures could not mimic in vivo microenvironments.92
3.3 Introduction of nanotopographies into 3-D fibrous scaffolds
The ideal tissue scaffolds resembling in vivo conditions should have both 3-D microstructures and nanotextured surfaces. One approach for fabricating such scaffolds is to use 3-D microfibrous scaffolds as the starting material and modify the surface of the microfibers by chemical etching or deposition of nanoparticles or nanofibers to create nanofeatures.
3.3.1 Chemical etching.
Chemical etching can create nanotopographies on the surface of 3-D fibrous scaffolds by soaking the scaffolds in etchants such as hydrofluoric acid (HF)63 and sodium hydroxide (NaOH).7 As the material is etched away, the nanoroughness of surface is enhanced with nanoscale pits and protrusions. For example, PLGA and poly(ether urethane) (PU) membranes with nanofeatures (50–100 nm) were obtained by soaking the films in 1 M NaOH for 1 h. The viability and function of cells cultured on these surfaces increased with decreased feature size, confirming that nanotopographies are beneficial to cells.93 In our recent work, we used NaOH to etch a highly porous and interconnected PET scaffold.8 While a short treatment of ∼1 h with NaOH increased the hydrophilicity of the fiber surfaces, longer treatment caused hydrolysis, and the etching process generated a nanostructured surface (see Fig. 3B). In this approach, the degree of nanoroughness present on the surface of the microfibers is dependent on the crystallinity of the microfiber and the hydrolysis time, which also affects the resulting porosity and pore size of the treated PET scaffolds. The increased nanoroughness, surface area and space in the treated scaffolds also increased cell growth and improved cellular functions. However, this method can only fabricate submicron-scale features and cannot achieve uniform surface modification.
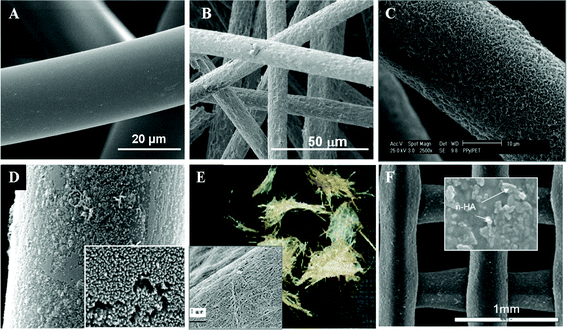 |
| Fig. 3 3-D microfibrous scaffolds with nanofeatures on their surfaces created by various methods: (A) pristine PET fibers with smooth surface; (B) PET fibers with rough surface after etching with NaOH; (C) PET fibers coated with polypyrrole nanofibers via sol–gel polymerization; (D) PET fibers coated with polyaniline nanofibers via sol–gel polymerization; (E) bacterial cellulose nanofibers attached on sisal fibers after fermentation; (F) composite PCL scaffold with HA nanoparticles obtained by extrusion of the mixture of nano-HA and PCL and stacked in layers to form the 3-D scaffold. (E and F are reproduced from ref. 96 and 99, respectively, with permission). | |
3.3.2 Polymer deposition.
The sol–gel process is a low-cost and versatile method for preparing nanofeatured 3-D scaffolds.94 In our study, polypyrrole nanofibers were formed using a sol–gel method and then coated on the surface of nonwoven PET scaffolds as the 3-D substrate. This method produced a thin layer of nanofibers uniformly coated on the surface of the PET fibers without significantly changing the original scaffold pore size or porosity (see Fig. 3C). Another method to control the rate of polymer deposition is through dilute polymerization.95 Similarly, a thin layer of aligned polyaniline nanofibers fabricated using a dilute polymerization method was coated on PET fibers (Fig. 3D). Cells cultured on these nanofibers-coated PET fibers showed different behaviors and morphologies from those on the pristine PET fibers.55
3.3.3 Bacterial cellulose deposition.
A simple method to introduce nanotopographies into 3-D fibrous scaffolds was developed by directly depositing bacterial cellulose nanofibrils onto natural fibers during bacterial cellulose fermentation.96 The mechanical properties of the modified fibers with cellulose nanofibrils (see Fig. 3E) were not affected, and may be used as scaffolds in tissue engineering. However, no tissue engineering research has been conducted with this scaffold material yet.
3.3.4 Nanoparticle deposition.
Nanoparticles with diameters less than 100 nm and a high surface to volume ratio can be used to produce nanofeatured scaffolds. Polyelectrolyte complex fibrous scaffolds were developed using poly(galacturonic acid) and chitosan via freeze drying. The resulting scaffolds had highly interconnected pores of 5–20 μm in diameter, and each individual fiber had a nanogranular structure, which promoted cell adhesion and cell proliferation.97 Freeze drying technology can be used to control porosity and pore size. However, it is limited in its capacity to yield a variety of pore structures.
Hydroxyapatite (HA), a synthetic calcium phosphate, has a similar structure to bone mineral. HA nanoparticles can reinforce composite materials for tissue engineering and can also be used as bimolecular carriers. A novel 3-D PCL/HA composite scaffold with a porosity of 73% and pore sizes of 500 μm was developed for bone regeneration using a layer-manufacturing process.98 A paste extruding deposition process was also developed to evenly disperse HA nanoparticles with diameters ranging from 50 to 100 nm on the surface of PCL micro-fibers (see Fig. 3F), and the PCL/HA scaffolds were successfully used for in vivo bone regeneration.99
Although the merits of using nano/microscale composite structures to promote cell proliferation are established, controlling the shape of composite structures cannot be achieved using conventional methods. Solid-free form (SFF) fabrication permits shape control. Among the SFF technologies, micro-stereolithography (MSTL) has the highest resolution, allowing fabricating structures with a resolution of 10 μm.100 During MSTL, an ultraviolet (UV) laser irradiates the free surface of a UV-curable liquid photopolymer, causing it to solidify to form a nano/microscale composite scaffold exhibiting nanotopographies on the scaffold surface due to the incorporation of HA nanopowder. The resulting pores, with diameters ranging from 330–360 nm, are well connected and have regular shapes. The scaffold with HA nanoparticles showed great potential in bone regeneration, significantly increasing cell proliferation and attachment as compared to the control because of the increased nanoroughness.100 In addition to HA nanoparticles, other nanoparticles have also been used to modify the surface of scaffolds. 3-D nanoengineered fibrous scaffolds were also developed by incorporating tripolyphosphate (TPP) into PLGA fibrous scaffolds using an emulsion followed by melt-spinning. The TPP nanoparticles, roughly 100 nm in diameter, solved the acidic degradation problems present in PGLA scaffolds.101
Although, 3-D fibrous scaffolds incorporated with nanoparticles have great potential in tissue engineering, it is necessary to understand the secondary effects and cytotoxicity of nanoparticles once they enter the body. The high surface to volume ratio of nanoparticles makes them highly reactive with surrounding tissues in vivo and their nanosize makes them more likely to penetrate cell membranes in the lungs, skin and intestinal tract.102
3.3.5 Composites of nanofibers and microfibers.
Electrospinning can be used to fabricate nanofibers and microfibers. It can also be used with pre-fabricated scaffolds to generate composites of nanofibers and microfibers. Nanoengineered 3-D fibrous scaffolds were fabricated by impregnating starch/PCL microfiber meshes with electrospun starch/PCL nanofibers (see Fig. 4A).103 The microfibers served as the structural support, whereas the nanofibers filling the large pores served as the networking anchors for the cells. A similar construct with electrospun type I collagen nanofibers and starch/PCL microfibers in the same structure was fabricated using a two-step process. In this process, the starch/PCL fibrous matrix was first fabricated using a wet spinning method and collagen nanofibers were then electrospun and deposited on the matrix, followed with crosslinking with saturated glutaraldehyde vapor at room temperature for 48 h. Cells cultured in this scaffold showed increased metabolic activity and growth rate.104 In addition, a multilayer nano/microfiber scaffold was produced by depositing multiple layers of electrospun PCL nanofibers on the top of microfibrous scaffolds (see Fig. 4B). The presence of nanofibers did not affect cell seeding in the microfiber scaffold; however, a difference in cell spreading on the surface of the scaffold was observed.
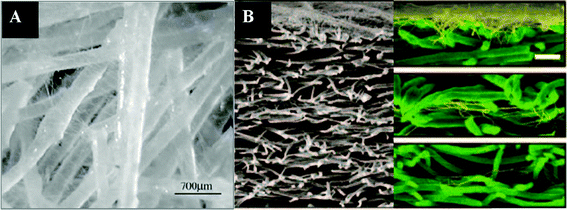 |
| Fig. 4 Composites of nanofibers and microfibers produced by electrospinning of nanofibers to existing microfibers: (A) nanofibers impregnated in a microfibrous scaffold; (B) cross-sections of layered scaffolds generated by sequential electrospinning of nanofibers on top of microfibers. (Reproduced from ref. 103 and 105, respectively, with permission). | |
The aforementioned scaffolds with uneven distribution of nanofibers and microfibers are not ideal for tissue engineering as cell migration is limited by the nanofibrous matrices with only nanoscale pores. It is thus desirable to create “micro-voids” in nanofibrous scaffolds to enhance cell migration.105 Another way to overcome this problem is to electrospin nanofibers onto single microfibers (see Fig. 5A). Electrospun nanofibers can be homogeneously deposited onto individual microfibers (Fig. 5B) and then the nanofiber-coated microfibers can be made into scaffolds with desirable shapes and tunable porosity (see Fig. 5C). Cellular infiltration and spreading along the nanofibers-coated microfibers were observed in the scaffolds, which also maintained their surface and structural properties.106
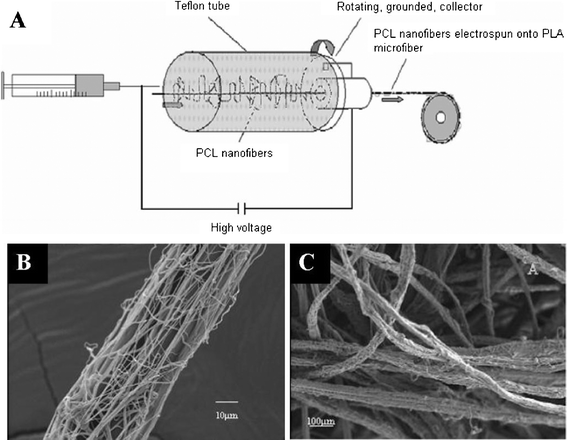 |
| Fig. 5 Electrospinning of PCL nanofibers onto a single PLA microfiber: (A) schematic diagram illustrating the process; (B) a single PLA microfiber with electrospun PCL nanofibers coated on its surface; (C) 3-D scaffold made of PLA microfibers coated with PCL nanofibers. (Reproduced from ref. 106 with permission). | |
3.4 Introduction of 3-D structures into nanofibrous scaffolds
In contrast to the previous approach of adding nanoscale properties to a 3-D scaffold, various approaches to develop 3-D structures in nanofibrous scaffolds have also been attempted and are discussed below.
3.4.1 Post-processing of electrospun nanofibers.
Nanofibrous scaffolds produced by electrospinning exhibit similar dimensions to collagen fibrils. However, cells cannot infiltrate into the nanofibrous meshes and generally sit on top of the electrospun fibers. The pore size of these nanofibrous scaffolds can be modified and increased through further processing with UV irradiation or assembling.
3.4.1.1 UV irradiation.
Although UV irradiation is usually ineffective for scaffold sterilization,107 it can degrade nanotextured materials and thus could be used to fabricate microporous scaffolds from electrospun nanofibers.108 By masking the nanofibers with a porous mesh and subjecting them to UV irradiation, the porosity and pore size of the scaffolds can be controlled by controlling the pore size of the porous mask. Degrading nanofibers using UV is a promising tool for producing tissue-specific microenvironments to regulate cellular behaviors. However, there are some issues about the lack of interconnectivity of the micropores and impaired structural or mechanical integrity and potential cytotoxicity caused by the degradation of and sintering in the scaffold induced by UV irradiation.109
3.4.1.2 Assembly of nanofibers.
Electrospun nanofibrous scaffolds can provide a nanoscale niche for cell arrangement but are limited by their low volume capacity. The assembly of nanofibers into 3-D structures can be used to overcome this limitation. For instance, a 3-D construct was produced by stacking multiple layers of electrospun nanofibrous membranes seeded with human bone marrow-derived mesenchymal stem cells.110 The multilayered construct met the need for a 3-D construct with a larger volume containing adequate cells for the in vivo implantation. However, the 3-D nanostructured scaffolds generated from the electrospun membranes may be too soft to be used as tissue scaffolds in bone regeneration.
Nanofibers can also be assembled by rolling into 3-D tubular scaffolds111 or using the layer-by-layer method to form 3-D structures that can support uniform cell distribution in the multilayered nanofibrous scaffolds.112 Polymer blends are widely used in this approach. A tubular scaffold for engineering small diameter blood vessels was fabricated by electrospinning polymer solution blends of pNSR32 (recombinant spider silk protein), PCL and gelatin.111 Also, PCL/collagen nanofibers were used to fabricate multilayered 3-D scaffolds, which showed a layered structure with uniform distribution.112
3.4.2 Direct introduction of 3-D structures into nanofibrous scaffolds.
3.4.2.1 Electrospinning with leaching.
Electrospinning with particulate leaching can be used to produce nanofibrous 3-D scaffolds.113 When electrospinning a salt-containing polyelectrolyte, the pore size of the resulting scaffold can be tuned by controlling the particle size of the salts. The nanofibrous polymer also contributes to the roughness of the scaffolds. In this system, although the porosity of the scaffolds could ensure appropriate mass transfer for nutrient transport and waste removal, the interconnectivity of the micropores has to be controlled to ensure cell infiltration inside the scaffolds.
Co-electrospinning of two different polymers followed by leaching of a polymer can also be used to produce a nanostructured scaffold with large pores.114 The pores of the scaffolds would, therefore, depend on the ratio between the nanofibers and the compactness of the nanofibers. Although this method can generate hybrid scaffolds with amorphous pore structures, the thickness of the scaffolds has to be properly controlled to ensure appropriate mechanical strength for structural support of the scaffold.
3.4.2.2 Cryogenic electrospinning.
Cryogenic electrospinning can control the pore size of electrospun scaffolds, which is impossible to do with conventional electrospinning processes.115 By lowering the temperature of the collection plate during electrospinning, the pore size of the electrospun scaffold can be increased. In this approach, the electrospun fibers were collected with the ice crystals formed within the fibrous matrix on the plate because of the higher humidity environment caused by the lower temperature. Macro-sized pores or voids could be formed in the nanofibrous network after the drying.115 However, the size of the ice crystals, which was critical to the control of the scaffold pore size, could not be controlled to produce scaffolds with homogeneous structures.
3.4.2.3 Wet electrospinning.
Wet spinning or electrospinning with a liquid reservoir, such as a coagulation bath, to collect the nanofibers before being drawn into a rotating roller can be used to produce continuous and bundled yarns.116 A 3-D nanofibrous scaffold with large pores and a high porosity was fabricated using silk fibroin (SF) via wet electrospinning (Fig. 6A). The SF nanofibers were dropped directly into a coagulation bath containing methanol below the spinneret, and a SF nanofiber foam was obtained after freeze-drying (see Fig. 6B), which can be molded into foams with desirable size and shape (see Fig. 6C). Cells in the 3-D SF nanofibrous foam proliferated well.117 However, scaffolds with desirable pore sizes are difficult to fabricate using this method.
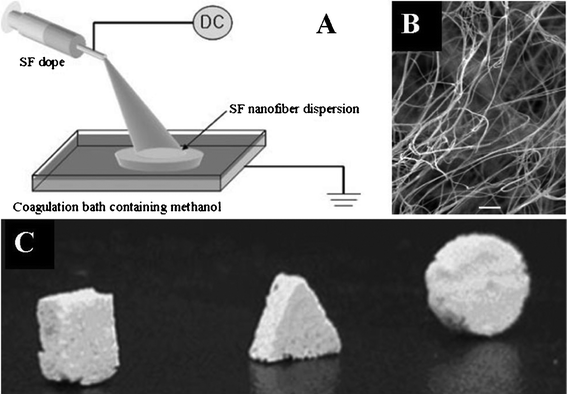 |
| Fig. 6 Wet electrospinning of silk fibroin (SF) nanofibers: (A) schematic diagram illustrating the process; (B) nanofibrous scaffold from the wet spinning (×1000); (C) various foam shapes made from the electrospun fibrous scaffolds. (Reproduced from ref. 117 with permission). | |
3.4.2.4 Phase separation.
Phase separation with gelatinization can generate microporous scaffolds with nanotextures.118 In this system, the nanotextures were generated as the result of phase separation, while larger pores were caused by gelatinization. This approach yielded a scaffold with surface roughness that improved protein adsorption and cell attachment. Thermally induced phase separation (TIPS) has been widely used to fabricate porous composite structures. By changing the temperature, a homogeneous polymer solution can be separated into a polymer-rich phase and a polymer-lean phase. After the removal of solvent using freeze-drying or vacuum, a 3-D porous scaffold can be formed. A variety of 3-D nanofibrous scaffolds have been fabricated using the phase separation process. For example, a PLLA solution was thermally induced to phase separation and then self-assembled into a 3-D interconnected fibrous network with a fiber diameter ranging from 50 to 500 nm and a high porosity up to 98.5%.
One drawback of the scaffolds generated by phase separation is the lack of interconnected macropores. To overcome this problem, TIPS combined with sugar sphere template leaching were developed to produce a nanofibrous PLLA scaffold with interconnected spherical macropores (Fig. 7A and B).119 The macropore shape and size can be tuned by controlling the sugar spheres. In addition, phase separation with salt particle leaching was also developed to produce nanofibrous scaffolds with tunable pore size and porosity (Fig. 7C and D). The nanofibrous scaffolds consisting of interconnected microscale pores could mimic the physical architecture and chemical composition of natural ECM, which significantly promoted cell differentiation and neuritis outgrowth.120 However, phase separation is limited to a few polymers and cannot produce long and continuous fibers with control over fiber orientation.
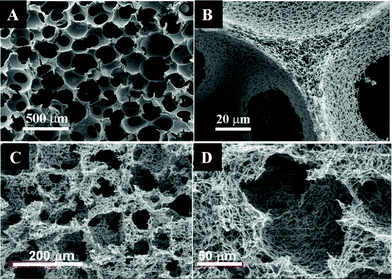 |
| Fig. 7 PLLA nanofibrous scaffolds produced by phase separation followed with particles bleaching; A and B are SEM images of the scaffolds from sugar spheres bleaching and C and D are from salt particles bleaching. (A and B are modified from ref. 119 and C and D form ref. 120, with permission). | |
3.5 Carbon nanotubes
Carbon nanotubes (CNTs) have attracted great attention due to their mechanical strength and excellent thermal, magnetic and electrical properties. Their dimensions, diameter and length, are analogous to major components in natural ECM, and their mechanical properties are similar to those of the ECM proteins.121 These unique characteristics have led to them finding numerous applications in biomedical materials, biosensors, drug delivery and tissue engineering.
Thin-film networks of carbon nanotubes with 3-D sieve architecture consisting of aligned CNTs with cavity sizes ranging from 5 to 65 μm (see Fig. 8A) were fabricated and used to culture human fibroblasts, which adsorbed and spread on the surface of the carpet-like carbon nanotubes.122 Microchannel porous scaffolds composed of multiwall carbon nanotubes (89 wt%) and a small portion of chitosan (see Fig. 8B) increased the differentiation of mouse myoblasts (C2C12) to osteoblastic cells.123 However, the lack of interconnectivity of these nanostructured 3-D materials hindered oxygen supply, nutrient transfer and cell migration. To overcome this limitation, a variety of nanoengineered 3-D polymer materials have been developed by attaching CNTs, using eletrophoretic deposition (EPD), to porous scaffolds, such as poly(propylene fumarate) (PPF),124 collagen,44,125 and polyurethane foams.126 In our recent work, CNTs were deposited on the surface of nonwoven PET matrices (see Fig. 8C) to provide nanofeatures, resulting in increased cell proliferation and differentiation. Although CNTs can be used to improve the performance of 3-D scaffolds, their applications in tissue engineering can be hampered by their potential adverse effects on human life and the environment, which remain to be further investigated.127,128
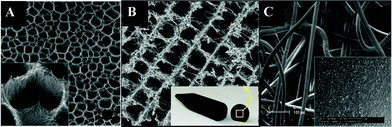 |
| Fig. 8 3-D scaffolds made from carbon nanotubes (CNTs): (A) vertically aligned CNTs on surface with micron-sized cavity; (B) CNTs/chitosan scaffold with a chamber-like structure; insert shows the monolithic scaffold prepared by the ice segregation induced self-assembly (ISISA) process; (C) PET fibers coated with CNTs via deposition. (A and B are reproduced from ref. 122 and 123, respectively, with permission). | |
4. Conclusions and future perspectives
The recent advancements in scaffold fabrication methods have provided biologists and tissue engineers the scaffolds needed for better understanding of cell-scaffold interactions. Scaffolds with nanoscale features offer great promise for enhancing the biological performance of cell and tissue cultures and will play an increasingly important role in tissue engineering.3 Several fabrication methods have been developed to produce 3-D scaffolds with microporous structures and tunable ‘surface’ properties with nanostructures similar to those present in natural extracellular matrices. These fabrication methods take different approaches, including bulk processing, incorporation of nanostructures onto the surface of microstructures and incorporation of microstructures into nanoscaffolds. Each of them has brought us one step closer to creating an ideal scaffold for tissue engineering applications. Although nanoengineered 3-D scaffolds have shown advantages in engineering various tissues and organs, including bone, cartilage, liver, blood vessels and stem cells, their development and applications in tissue engineering is still in an early stage. This review highlights the recent progress and challenges in developing fabrication methods for generating fibrous microscaffolds with nanofeatures for tissue engineering applications.129–131 Although some modular tissue fabrication methods such as tissue printing and direct assembly are promising,132 more work still needs to be done. How to scale up the fabrication processes capable of producing macro-scale scaffolds with high precision, control and homogeneity in micro- and nanostructures suitable for various clinical applications remains a major challenge.133 Besides, in-depth understanding and control of cellular functioning through topographical and microstructural modifications are critical to the design and development of a functional synthetic tissue scaffold. To obtain such a scaffold, the balance and synergistic interactions between the micro- and nanotopographical effects to the cells should be considered.134
In addition to providing the structural cue resembling the morphology of native ECM, a tissue scaffold should also provide relevant chemical and biological cues that are critical to tissue performance and cell guidance. Several successful attempts to provide specific biologically recognizable patterns by incorporating oligopeptides or other ECM proteins, such as laminin, fibronectin, collagen and vitronectin, onto the scaffold surface have been reported.135 Thus, the next-generation scaffolds also need to be functionalized with growth factors and other biomolecules that can accelerate tissue regeneration and enhance regenerated tissue function through temporal and spatial control of the release of these biomolecules.136
In addition, incorporating microfluidics in scaffold fabrication and tissue engineering studies has also been widely reported and has become one of the major research areas in the field of tissue engineering. The incorporation of microfluidics affords tissue constructs the ability to precisely secrete cytokines of interest at a desired rate and stage during tissue growth.137 Microfluidics can be used to create a unique microenvironment around the cells in the scaffold, such as to generate a gradient of cytokines and different flow patterns138 to induce directional cell growth,139 migration,140 and differentiation.141 Microfluidics can also provide a powerful tool and solution to the challenges in next-generation tissue scaffold design: how to induce vascularization142 or incorporate blood-vessel like conduits for nutrient supply and metabolite transport in a large-scale scaffold.143 Finally, it may be necessary to integrate scaffolds in a bioreactor that can provide a complex and dynamic environment with control of physiological parameters (e.g., pH, temperature, etc.) and exert appropriate mechanical forces such as shear stress and hydrodynamic pressure to guide cell growth, differentiation and functional assembly,144 which is important for in vitro applications, including mass production of stem cells for regenerated medicine and drug screening.145
In summary, an ideal synthetic tissue scaffold should provide structural, chemical, mechanical and biochemical signals that are synergistic to the intended tissue formation.146 It will require a collaborative effort from biologists, chemists, material scientists, engineers and clinical surgeons to design and assemble functional 3-D nanoengineered scaffolds that are clinically and economically feasible for tissue regeneration applications.
Acknowledgements
This work was supported in part by the Ohio State University Alumni Grants for Graduate Research and Scholarship (AGGRS) fund. Some of the research work by the authors mentioned in this paper was supported by the NSF Center for Affordable Nanoengineering of Polymeric Biomedical Devices (Grant No. EEC-0425626).
References
- R. Langer and J. P. Vacanti, Tissue Engineering, Science, 1993, 260, 920–926 CAS.
- M. Sittinger, J. Bujia, N. Rotter, D. Reitzel, W. W. Minuth and G. R. Burmester, Tissue engineering and autologous transplant formation: practical approaches with resorbable biomaterials and new cell culture techniques, Biomaterials, 1996, 17, 237–242 CrossRef CAS.
- D. Coutinho, P. Costa, N. Neves, M. E. Gomes and R. L. Reis, Micro- and nanotechnology in tissue engineering, Tissue Eng., 2011, part 1, 3–29 CrossRef.
- J. W. Haycock, 3D cell culture: Methods and protocols, Methods Mol. Biol., 2011, 695, 1–14 CAS.
- Y. Li and S. T. Yang, Effects of three-dimensional scaffolds on cell organization and tissue development, Biotechnol. Bioprocess Eng., 2001, 6, 311–325 CrossRef CAS.
- Y. Li, T. Ma, D. A. Kniss, S. T. Yang and L. C. Lasky, Human cord cell hematopoiesis in three-dimensional nonwoven fibrous matrices: In vitro simulation of the marrow microenvironment, Stem Cell Res., 2001, 10, 355–368 CAS.
- R. N.g, X. Zhang, N. Liu and S. T. Yang, Modifications of nonwoven polyethylene terephthalate fibrous matrices via NaOH hydrolysis: Effects on pore size, fiber diameter, cell seeding and proliferation, Process Biochem., 2009, 44, 992–998 CrossRef CAS.
- L. Bozec, G. van der Heijden and M. Horton, Collagen fibrils: Nanoscale ropes, Biophys. J., 2007, 92, 70–75 CrossRef CAS.
- A. Abbott, Cell culture: Biology's new dimension, Nature, 2003, 424, 870–872 CrossRef CAS.
- J. K. Hong and S. V. Madihally, Next generation of electrosprayed fibers for tissue regeneration, Tissue Eng., Part B: Rev., 2011, 17, 125–142 CrossRef CAS.
- J. Igwe, A. Amini, P. Mikael, C. Laurencin and S. Nukavarapu, Nanostructured scaffolds for bone tissue engineering, Studies in Mechanobiology, Tissue Eng. Biomater., 2011, 8, 169–192 Search PubMed.
- E. Carletti, A. Motta and C. Migliaresi, Scaffolds for tissue engineering and 3D cell culture, Methods Mol. Biol., 2011, 695, 17–39 CAS.
- H. G. C.raighead, S. W. Turner, R. C. Davis, C. James, A. M. Perez, L. Kam, W. Shain, J. N. Turner and G. Banker, Chemical and topographical surface modification for control of central nervous system cell adhesion, Biomed. Microdevices, 1998, 1, 49–64 CrossRef CAS.
- S. H. Hsu, C. Y. Chen, P. S. Lu, C. S. Lai and C. J. Chen, Oriented Schwann cell growth on microgrooved surfaces, Biotechnol. Bioeng., 2005, 92, 579–588 CrossRef CAS.
-
S. Basu, Dissertation: Effects of three dimensional structure of tissue scaffolds on animal cell culture, The Ohio State University, p. 2004.
- S. Basu and S. T. Yang, Astrocyte growth and glial cell line-derived neurotrophic factor secretion in three-dimensional polyethylene terephthalate fibrous matrices, Tissue Eng., 2005, 11, 940–952 CrossRef CAS.
- M. E. Gomes and R. L. Reis, Tissue engineering: key elements and some trends, Macromol. Biosci., 2004, 4, 737–742 CrossRef CAS.
- R. Ng, J. S. Gurm and S. T. Yang, Centrifugal seeding of mammalian cells in nonwoven fibrous matrices, Biotechnol. Prog., 2010, 26, 239–245 CrossRef CAS.
- Y. Yang, S. Basu, D. L. Tomasko, L. J. Lee and S. T. Yang, Fabrication of well-defined PLGA scaffolds using novel microembossing and carbon dioxide bonding, Biomaterials, 2005, 26, 2585–2594 CrossRef CAS.
- D. E. Ingber, Tensegrity: The architectural basis of cellular mechanotransduction, Annu. Rev. Physiol., 1997, 59, 575–599 CrossRef CAS.
- G. Chen, D. Liu, M. Tadokoro, R. H.irochika, H. Ohgushi, J. Tanaka and T. Tatishi, Chondrogenic differentiation of human mesenchymal stem cells cultured in a cobweb-like biodegradable scaffold, Biochem. Biophys. Res. Commun., 2004, 322, 50–55 CrossRef CAS.
- Y. Lai, A. Asthana and W. S. Kisaalita, Biomarkers for simplifying HTS, 3D cell culture platforms for drug discovery: the case for cytokines, Drug Discovery Today, 2011, 16, 293–297 CrossRef CAS.
- P. Friedl and E. B. Brocker, The biology of cell locomotion within three-dimensional extracellular matrix, Cell. Mol. Life Sci., 2000, 57, 41–64 CrossRef CAS.
- E. Cukierman, R. Pankov and K. M. Yamada, Cell interactions with three-dimensional matrices, Curr. Opin. Cell Biol., 2002, 14, 633–640 CrossRef CAS.
- H. C. Feigel, J. Havers, U. Kneser, M. K. Smith, T. Moeller, D. Kluth, D. J. Mooney, X. Rogiers and P. M. Kaufmann, Influence of flow conditions and matrix coatings on growth and differentiation of three-dimensionally cultured rat hepatocytes, Tissue Eng., 2004, 10, 165–174 CrossRef.
- K. Cheng, Y. Lai and W. S. Kisaalita, Three-dimensional polymer scaffolds for high throughput cell-based assay systems, Biomaterials, 2008, 29, 2802–2812 CrossRef CAS.
- W. L. Grayson, T. Ma and B. Bunnell, Human mesenchymal stem cells tissue development in 3D PET matrices, Biotechnol. Prog., 2004, 20, 905–912 CrossRef CAS.
- J. Luo and S. T. Yang, Effects of three-dimensional culturing in a fibrous matrix on cell cycle, apoptosis, and MAb production by hybridoma cells, Biotechnol. Prog., 2008, 20, 306–315 CrossRef.
- L. E. Freed, G. V. Novakoniv and R. Langer, Cultivation of cell-polymer cartilage implants in bioreactors, J. Cell. Biochem., 1993, 51, 257–264 CrossRef CAS.
- S. Battista, D. Guarnien, C. Borselli, S. Zeppetelli, A. Borzacchiello, L. Mayol, D. Gerbasio, D. R. Keene, L. Ambrosio and P. A. Netti, The effect of matrix composition of 3D constructs on embryonic stem cell differentiation, Biomaterials, 2005, 26, 6194–6207 CrossRef CAS.
- J. Shi, A. R. Votruba, O. C. Farokhzad and R. Langer, Nanotechnology in drug delivery and tissue engineering: from discovery to applications, Nano Lett., 2010, 10, 3223–3230 CrossRef CAS.
- M. Köunönen, M. Hormia, J. Kivilahti, J. Hautaniemi and I. Thesleff, Effect of surface processing on the attachment, orientation, and proliferation of human gingival fibroblasts on titanium, J. Biomed. Mater. Res., 1992, 26, 1325–1341 CrossRef.
- J. Y. Lim and H. J. Donahue, Cell sensing and response to micro- and nanostructured surfaces produced by chemical and topographic patterning, Tissue Eng., 2007, 13, 1879–1891 CrossRef CAS.
- J. Y. Wong, R. Langer and D. E. Ingber, Electrically conducting polymers can noninvasively control the shape and growth of mammalian cells, Proc. Natl. Acad. Sci. U. S. A., 1994, 91, 3201–3204 CrossRef CAS.
- R. L. Williams and P. J. Doherty, A preliminary assessment of poly (pyrrole) in nerve guide studies, J. Mater. Sci.: Mater. Med., 1994, 5, 429–433 CrossRef CAS.
- J. Y. Wong, J. B. Leach and X. Q. Brown, Balance of chemistry, topography, and mechanics at the cell-biomaterial interface: Issues and challenges for assessing the role of substrate mechanics on cell response, Surf. Sci., 2004, 570, 119–133 CrossRef CAS.
- L. A. Smith, X. Liu and P. X. Ma, Tissue engineering with nano-fibrous scaffolds, Soft Matter, 2008, 4, 2144–2149 RSC.
- H. Zhu, J. Ji and J. Shen, Biomacromolecules electrostatic self-assembly on 3-dimensional tissue engineering scaffold, Biomacromolecules, 2004, 5, 1933–1939 CrossRef CAS.
- T. V. Kumari, U. Vasudev, A. Kumar and B. Menon, Cell surface interactions in the study of biocompatibility, Trends Biomater. Artif. Organs, 2002, 15, 37–41 Search PubMed.
- R. Jain and A. F. von Recum, Fibroblast attachment to smooth
and microtextured PET and thin cp-Ti films, J. Biomed. Mater. Res., 2004, 68A, 296–304 CrossRef CAS.
- K. M. Woo, V. J. Chen and P. X. Ma, Nano-fibrous scaffolding architecture selectively enhances protein adsorption contributing to cell attachment, J. Biomed. Mater. Res., 2003, 67A, 531–537 CrossRef CAS.
- J. B. McGrohon, W. D. Holder Jr, L. W. Grimes, C. B. Thomas and K. J. L. Burg, Evaluation of smooth muscle cell response using two types of porous polylactide scaffolds with differing pore topography, Tissue Eng., 2004, 10, 505–514 CrossRef.
- M. T. Concini, S. Lora, S. Baiguera, E. Boscolo, M. Folin, R. Scienza and P. Rebuffat, In vitro culture of rat neuromicrovascular endothelial cells on polymeric scaffolds, J. Biomed. Mater. Res., 2004, 71A, 669–674 CrossRef.
- E. H. irata, M. Uo, Y. N. odasaka, H. T. akita, N. Ushijima, T. Akasaka, F. Watari and A. Yokoyama, 3D collagen scaffolds coated with multiwalled carbon nanotubes: Initial cell attachment to internal surface, J. Biomed. Mater. Res. B: Appl. Biomater., 2010, 93, 544–550 Search PubMed.
- G. T. Christopherson, H. Song and H. Q. Mao, The influence of fiber diameter of electrospun substrates on neural stem cell differentiation and proliferation, Biomaterials, 2009, 30, 556–564 CrossRef CAS.
- S. Petronis, C. Gretzer, B. Kasemo and J. Gold, Model porous surfaces for systematic studies of material-cell interactions, J. Biomed. Mater. Res., 2003, 66A, 707–712 CrossRef CAS.
- B. Y. Min, G. Lee, S. M. Kim, Y. S. Nam, T. K. Lee and W. H. Park, Electrospinning of silk fibroin nanofibers and its effect on the adhesion and spreading of normal human keratinocytes and fibroblasts in vitro, Biomaterials, 2004, 25, 1289–1297 CrossRef CAS.
- K. N. Chua, W. S. Lim, P. C. Zhang, H. F. Lu, J. Wen, S. Ramakrishna, K. W. Leong and H. Q. Mao, Stable immobilization of rat hepatocyte spheroids on galactosylated nanofiber scaffold, Biomaterials, 2005, 26, 2537–2547 CrossRef CAS.
- K. A. Diel, J. D. Foley, P. F. Nealey and C. J. Murphy, Nanoscale topography modulates corneal epithelial cell migration, J. Biomed. Mater. Res., Part A, 2005, 75A, 603–611 CrossRef.
- P. Van der Valk, A. W. J. Pelt, H. J. Busscher, H. P. de Jong, C. R. H. Wildevuur and J. Arends, Interaction of fibroblasts and polymer surfaces: relationship between surface free energy and fibroblast spreading, J. Biomed. Mater. Res., 1983, 17, 807–817 CrossRef CAS.
- J. M. Schakenraad, H. J. Busscher, C. R. H. Wildevuur and J. Arens, The influence of substratum surface free energy on growth and spreading of human fibroblasts in the presence and absence of serum proteins, J. Biomed. Mater. Res., 1986, 20, 773–784 CrossRef CAS.
- G. Z.hao, Z. Schwartz, M. Wieland, F. Rupp, J. GEis-Gerstorfer, D. L. Cochran and B. D. Boyan, High surface energy enhances cell response to titanium substrate microstructure, J. Biomed. Mater. Res., Part A, 2005, 74A, 49–58 CrossRef CAS.
- I. O. Smith, M. J. Baumann and L. R. McCabe, Electrostatic interactions as a predictor for osteoblast attachment to biomaterials, J. Biomed. Mater. Res., 2004, 70A, 436–441 CrossRef CAS.
- D. Lehnart, B. Wehrle-Haller, C. David, U. Weiland, C. Ballestrem, B. A. Imhof and M. Bastmeyer, Cell behaviour on micropatterned substrata: limits of extracellular matrix geometry for spreading and adhesion, J. Cell Sci., 2004, 117, 41–52 CrossRef.
-
R. Ng, Novel tissue scaffolds comprising nano- and micro- structures, Dissertation, The Ohio State University, 2007.
- F. Yang, R. Murugan, S. Ramakrishna, X. Wang, Y. X. Wang and S. Wang, Fabrication of nano-structured porous PLLA scaffold intended for nerve tissue
engineering, Biomaterials, 2004, 25, 1891–1900 CrossRef CAS.
- H. S. Koh, T. Yong, C. K. Chan and S. Ramakhrisna, Enhancement of neurite outgrowth using nano-structured scaffolds coupled with laminin, Biomaterials, 2008, 29, 3574–3582 CrossRef CAS.
- C. H. Lee, H. J. Shin, I. H. Cho, Y. M. Kang, I. A. Kim, K. D. Park and J. W. Shin, Nanofiber alignment and direction of mechanical strain affect the ECM production of human ACL fibroblast, Biomaterials, 2005, 26, 1261–1270 CrossRef CAS.
- M. J. Dalby, M. O. Riehle, D. S. Sutherland, H. Agheli and A. S. G. Curtis, Fibroblast response to a controlled nanoenvironment produced by colloidal lithography, J. Biomed. Mater. Res., 2004, 69A, 314–322 CrossRef CAS.
- R. J. Vance, D. C. Miller, A. Thapa, K. M. Habersroth and T. J. Webster, Decreased fibroblast cell density on chemically degraded poly-lactic-co-glycolic acid, polyurethane, and polycaprolactone, Biomaterials, 2004, 25, 2095–2103 CrossRef CAS.
- G. T. Christopherson, H. Song and H. Q. Mao, The influence of fiber diameter of electrospun substrates on neural stem cell differentiation and proliferation, Biomaterials, 2009, 30, 556–564 CrossRef CAS.
- J. L. McKenzie, M. C. Waid, R. Shi and T. J. Webster, Decreased functions of astrocytes on carbon nanofiber materials, Biomaterials, 2004, 25, 1309–1317 CrossRef CAS.
- L. Yin, H. Bien and E. Entcheva, Scaffold topography alters intracellular calcium dynamics in cultured cardiomyocyte networks, Am. J. Heart Circ. Physiol., 2004, 287, H1267–H1285 Search PubMed.
- W. J. Li, R. Tuli, X. Huang, P. Laquerriere and R. S. Tuan, Multilineage differentiation of human mesenchymal stem cells in a three-dimensional nanofibrous scaffold, Biomaterials, 2005, 26, 5158–5166 CrossRef CAS.
- L. G. M.obarakeh, M. P. Prabhakaran, M. Morshed and S. Ramakrishna, Electrospun poly(ε-caprolactone)/gelatin nanofibrous scaffolds for nerve tissue engineering, Biomaterials, 2008, 29, 4532–4539 CrossRef.
- N. S. Binulal, M. Deepthy, N. Selvamurugan and S. V. Nair, Role of nanofibrous poly(caprolactone) scaffolds in human mesenchymal stem cell attachment and spreading for in vitro bone tissue engineering—Response to osteogenic regulators, Tissue Eng. A, 2010, 16, 393–405 CrossRef CAS.
- A. Nur-E-Kamal, I. Ahmed, J. Kamal, M. Schindler and S. Meiners, Three dimensional nanofibrillar surfaces induce activation of Rac, Biochem. Biophys. Res. Commun., 2005, 331, 428–434 CrossRef CAS.
- J. Y. Martin, Z. Schwartz, T. W. Hummert, D. M. Scharaub, J. Lankford Jr, D. D. Dean, D. L. Cochran and B. D. Boyan, Effect of titanium surface-roughness on proliferation, differentiation, and protein-synthesis of human osteoblast-like cells (Mg63), J. Biomed. Mater. Res., 1995, 29, 389–401 CrossRef CAS.
- S. T. Yang and R. Ng, A new dimension to biomaterials, Mater. Today, 2007, 10, 64 CrossRef.
- L. A. Smith, X. Liu and P. X. Ma, Tissue engineering with nano-fibrous scaffolds, Soft Matter, 2008, 4, 2144–2149 RSC.
- M. N. Rahaman, D. E. Day, B. S. Bal, Q. Fu, S. B. Jung, L. F. Bonewald and A. P. Tomsia, Bioactive glass in tissue engineering, Acta Biomater., 2011, 7, 2355–2373 CrossRef CAS.
- F. Yang, R. Murugan, S. Wang and S. Ramakhrisna, Electrospinning of nano/micro scale poly (l-lactic acid) aligned fibers and their potential in neural tissue engineering, Biomaterials, 2005, 26, 2603–2610 CrossRef CAS.
- N. Grabow, K. Schmohl, A. Khosravi, M. Philipp, M. Scharfschwerdt, B. Graf, C. Stamm, A. Haubold, K. P. Schmitz and G. Steinhoff, Mechanical and Structural Properties of a Novel Hybrid Heart Valve Scaffold for Tissue Engineering, Artif. Organs, 2004, 28, 971–979 CrossRef CAS.
- X. Ma and H. J. Weber, Electrospinning P(LLA-CL) nanofiber: A tubular scaffold fabrication with circumferential alignment, Macromol. Symp., 2004, 217, 413–316 CrossRef.
- T. Ma, Y. Li, S. T. Yang and D. A. Kniss, Effects of pore size in 3-D fibrous matrix on human trophoblast tissue development, Biotechnol. Bioeng., 2000, 70, 606–618 CrossRef CAS.
- R. Barbucci and G. Leone, Formation of defined microporous 3D structures starting from cross-linked hydrogels, J. Biomed. Mater. Res., 2004, 68B, 117–126 CrossRef CAS.
- L. Almany and D. Seliktar, Biosynthetic hydrogel scaffolds made from fibrinogen and polyethylene glycol for 3D cell cultures, Biomaterials, 2005, 26, 2467–2477 CrossRef CAS.
- E. A. Botchwey, M. A. Dupree, S. R. Pollack, E. M. Levine and C. T. Laurencin, Tissue engineered bone: Measurement of nutrient transport in three-dimensional matrices, J. Biomed. Mater. Res., 2003, 67A, 357–367 CrossRef CAS.
- F. J. O'Brien, B. A. Harley, I. V. Yannas and L. J. Gibson, The effect of pore size on cell adhesion in collagen-GAG scaffolds, Biomaterials, 2005, 26, 433–441 CrossRef CAS.
- C. X. F. Lam, X. M. Mo, S. H. Teoh and D. W. Hutmacher, Scaffold development using 3D printing with a starch-based polymer, Mater. Sci. Eng., C, 2002, 20, 49–56 CrossRef.
- A. G. Mikos, Y. Bao, L. Cima, D. E. Ingber, J. P. Vacanti and R. Langer, Preparation of poly(glycolic acid) bonded fiber structures for cell attachment and transplantation, J. Biomed. Mater. Res., 1993, 27, 183–189 CrossRef CAS.
- S. P. Nalawade, F. Picchioni and L. P. B. M. Janssen, Supercritical carbon dioxide as a green solvent for processing polymer melts: Processing aspects and applications, Prog. Polym. Sci., 2006, 31, 19–43 CrossRef CAS.
- T. J. Sill and H. A. von Recum, Electrospinning: Applications in drug delivery and tissue engineering, Biomaterials, 2008, 29, 1989–2006 CrossRef CAS.
- Y. S. Nam and T. G. Park, Porous biodegradable polymeric scaffolds prepared by thermally induced phase separation, J. Biomed. Mater. Res., 1999, 47, 8–17 CrossRef CAS.
- Y. S. Nam, J. J. Yoon and T. G. Park, A novel fabrication method of macroporous biodegradable polymer scaffolds using gas foaming salt as a porogen additive, J. Biomed. Mater. Res., 2000, 53, 1–7 CrossRef CAS.
- J. M. Taboas, R. D. Maddox, P. H. Krebsbach and S. J. Hollister, Indirect solid free form fabrication of local and global porous, biomimetic and composite 3D polymer-ceramic scaffolds, Biomaterials, 2003, 24, 181–194 CrossRef CAS.
- V. P. Shastri, I. Martin and R. Langer, Macroporous polymer foams by hydrocarbon templating, Proc. Natl. Acad. Sci. U. S. A., 2000, 97, 1970–1975 CrossRef CAS.
- H. G. Kang, S. Y. Kim and Y. M. Lee, Novel porous gelatin scaffolds by overrun/particle leaching process for tissue engineering applications, J. Biomed. Mater. Res., Part B, 2006, 79B, 388–397 CrossRef CAS.
- J. J. Norman and T. A. Desai, Methods for fabrication of nanoscale topography for tissue engineering scaffolds, Ann. Biomed. Eng., 2006, 34, 89–101 CrossRef.
- Q. P. Pham, U. Sharma and A. G. Mikos, Electrospinning of polymeric nanofibers for tissue engineering applications, Tissue Eng., 2006, 12, 1197–1211 CrossRef CAS.
- I. A.hmed, A. S. Ponery, A. Nur-E-Kamal, J. Kamal, A. S. Meshel, M. P. Sheets, M. Schindler and S. Meiners, Morphology, cytoskeletal organization, and myosin dynamics of mouse embryonic fibroblasts cultured on nanofibrillar surfaces, Mol. Cell. Biochem., 2007, 301, 241–249 CrossRef CAS.
- W. J. Li, R. Tuli, C. Okafor, A. Derfoul, K. G. Danielson, D. J. Hal and R. S. Tuan, A three-dimensional nanofibrous scaffold for cartilage tissue engineering using human mesenchymal stem cells, Biomaterials, 2005, 26, 599–609 CrossRef CAS.
- A. Thapa, D. C. Miller, T. J. Webster and K. M. Haberstroh, Nano-structured polymers enhance bladder smooth muscle cell function, Biomaterials, 2003, 24, 2915–2926 CrossRef CAS.
-
C. J. Brinker and G. W. Scherer, Sol–Gel Science: The physics and chemistry of sol–gel processing, Academic Press, San Diego, CA, 1990, p. 907 Search PubMed.
-
N. R. Chiou, Aligned and oriented polyaniline nanofibers: fabrications and applications, Dissertation, The Ohio State University, 2006.
- M. Pommet, J. Juntaro, J. Y. Y. Heng, A. Mantalaris, A. F. Lee, K. Wilson and A. Bismarck, Surface modification of natural fibers using bacteria: Depositing bacterial cellulose onto natural fibers to create hierarchical fiber reinforced nanocomposites, Biomacromolecules, 2008, 9, 1643–1651 CrossRef CAS.
- D. Verma, K. S. Katti and D. R. Katti, Polyelectrolyte-complex nanostructured fibrous scaffolds for tissue engineering, Mater. Sci. Eng., C, 2009, 29, 2079–2084 CrossRef CAS.
- S. J. Heo, S. E. Kim, J. Wei, D. H. Kim, Y. T. Hyun, H. S. Yun, H. K. Kim, T. R. Yoon, S. H. Kim, S. A. Park and J. W. Shin, In vitro and animal study of novel nano-hydroxyapatite/poly(ε-caprolactone) composite scaffolds fabricated by layer manufacturing process, Tissue Eng. A, 2009, 15, 977–989 CrossRef CAS.
- S. E. Kim, H. S. Yun, Y. T. Hyun, J. W. Shin and J. J. Song, Nano-hydroxyapatite/poly ε-caprolactone composite 3D scaffolds for mastoid obliteration, J. Phys.: Conf. Ser., 2009, 165, 012083 CrossRef.
- J. W. Lee, G. S. Ahn, D. S. Kim and D. W. Cho, Development of nano- and microscale composite 3D scaffolds using PPF/DEF-HA and micro-stereolithography, Microelectron. Eng., 2009, 86, 1465–1467 CrossRef CAS.
- S. Xie, Q. Zhu, B. W.ang, H. Gu, W. L.iu, L. Cui and Y. Cao, Incorporation of tripolyphosphate nanoparticles into fibrous poly(lactide-co-glycolide) scaffolds for tissue engineering, Biomaterials, 2010, 31, 5100–5109 CrossRef CAS.
- E. Engel, A. M.ichiardi, M. Navarro, D. Lacroix and J. A. Planell, Nanotechnology in regenerative medicine: the materials side, Trends Biotechnol., 2008, 26, 39–47 CrossRef CAS.
- K. Tuzlakoglu, N. Bolgen, A. J. Salgado, M. E. Gomes, E. Piskin and R. L. Reis, Nano- and micro-fiber combined scaffolds: A new architecture for bone tissue engineering, J. Mater. Sci.: Mater. Med., 2005, 16, 1099–1104 CrossRef CAS.
- K. Tuzlakoglu, M. I. Santos, N. Neves and R. L. Reis, Design of nano- and microfiber combined scaffolds by electrospinning of collagen onto starch-based fiber meshes: A man-made equivalent of natural extracellular matrix, Tissue Eng. A, 2011, 17, 463–473 CrossRef CAS.
- Q. P. Pham, U. Sharma and A. G. Mikos, Electrospun poly(ε-caprolactone) microfiber and multilayer nanofiber/microfiber scaffolds: characterization of scaffolds and measurement of cellular infiltration, Biomacromolecules, 2006, 7, 2796–2805 CrossRef CAS.
- A. Thorvaldsson, H. Stenhamre, P. Gatenholm and P. Walkenstron, Electrospinning of Highly Porous Scaffolds for Cartilage Regeneration, Biomacromolecules, 2008, 9, 1044–1049 CrossRef CAS.
- R. Ng, J. S. Gurm and S. T. Yang, Benzalkonium chloride sterilization of nonwoven fibrous scaffolds for astrocyte culture, Open Biotechnol. J., 2009, 3, 73–78 CrossRef CAS.
- Y. Dong, T. Yong, S. Loai, C. K. Chan and S. Ramakrishna, Degradation of electrospun nanofiber scaffold by short wave length ultraviolet radiation treatment and its potential applications in tissue engineering, Tissue Eng. A, 2008, 14, 1321–1329 CrossRef.
- S. P. Zhong, Y. Z Zhang and C. d. T. Lim, Fabrication of large
pores in electrospun nanofibrous scaffolds for cellular infiltration: A review, Tissue Eng., Part B: Rev., 2012, 18, 77–87 CrossRef CAS.
- S. Srouji, T. Kizhner, E. Suss-Tobi, E. Livne and E. Zussman, 3-D Nanofibrous electrospun multilayered construct is an alternative ECM mimicking scaffold, J. Mater. Sci.: Mater. Med., 2007, 19, 1249–1255 CrossRef.
- P. Xiang, M. Li, C. Zhang, D. Chen and Z. Zhou, Cytocompatibility of electrospun nanofiber tubular scaffolds for small diameter tissue engineering blood vessels, Int. J. Biol. Macromol., 2011, 49, 281–288 CrossRef CAS.
- X. Yang, J. D. Shah and H. Wang, Nanofiber enabled layer-by-layer approach toward three-dimensional tissue formation, Tissue Eng. A, 2009, 15, 945–956 CrossRef CAS.
- Y. H. Lee, J. H. Lee, I. G. An, C. Kim, D. S. Lee, Y. K. Lee and J. D. Nam, Electrospun dual-porosity structure and biodegradation morphology of Montmorillonite reinforced PLLA nanocomposite scaffolds, Biomaterials, 2005, 26, 3165–3172 CrossRef CAS.
- S. Kidoaki, I. K. Kwon and T. Matsuda, Mesoscopic spatial designs of nano- and microfiber meshes for tissue-engineering matrix and scaffold based on newly devised multilayering and mixing electrospinning techniques, Biomaterials, 2005, 26, 37–46 CrossRef CAS.
- M. Simonet, O. D. Schneider, P. Neuenschwander and W. J. Stark, Ultraporous 3D polymer meshes by low-temperature electrospinning: Use of ice crystals as a removable void template, Polym. Eng. Sci., 2007, 47, 2020–2026 CAS.
- E. Smit, U. Buttner and R. D. Sanderson, Continuous yarns from electrospun fibers, Polymer, 2005, 46, 2419–2423 CrossRef CAS.
- C. S. Ki, J. W. Kim, J. H. Hyun, K. H. Lee, M. Hattori, D. K. Rah and Y. H. Park, Electrospun three-dimensional silk fibroin nanofibrous scaffold, J. Appl. Polym. Sci., 2007, 106, 3922–3928 CrossRef CAS.
- P. X. Ma and R. Zhang, Synthetic nano-scale fibrous extracellular matrix, J. Biomed. Mater. Res., 1999, 46, 60–72 CrossRef CAS.
- K. M. Woo, V. J. Chen and P. X. Ma, Nano-fibrous scaffolding architecture selectively enhances protein adsorption contributing to cell attachment, J. Biomed. Mater. Res., 2003, 67A, 531–537 CrossRef CAS.
- K. Cheng and W. S. Kisaalita, Exploring cellular adhesion and differentiation in a micro-/nano-hybrid polymer scaffold, Biotechnol. Prog., 2010, 26, 838–846 CrossRef CAS.
- T. C. hao, S. Xiang, J. F. Lipstate, C. Wang and J. Lu, Poly(methacrylic acid)-grafted carbon nanotube scaffolds enhance differentiation of hESCs into neuronal cell, Adv. Mater., 2010, 22, 3542–3547 CrossRef.
- M. A. C.orrea-Duarte, N. Wagner, J. Rojas-Chapana, C. Morsczeck, M. Thie and M. Giersig, Fabrication and biocompatibility of carbon nanotube-based 3D networks as scaffolds for cell seeding and growth, Nano Lett., 2004, 4, 2233–2236 CrossRef CAS.
- A. Abarrategi, M. C. Gutierrez and C. Moreno-Vicente, Multiwall carbon nanotube scaffolds for tissue engineering purposes, Biomaterials, 2008, 29, 94–102 CrossRef CAS.
- X. Shi, B. Sitharaman, Q. P. Pham, F. Liang, K. Wu, W. E. Billups, J. L. Wilson and A. G. Mikos, Fabrication of porous ultra-short single-walled carbon nanotube nanocomposite scaffolds for bone tissue engineering, Biomaterials, 2007, 28, 4078–90 CrossRef CAS.
- E. H.irata, M. Uo, H. T. akita, T. Akasaka, F. Watari and A. Yokoyama, Development of a 3D collagen scaffold coated with multiwalled carbon nanotubes, J. Biomed. Mater. Res. B: Appl. Biomater., 2009, 90, 629–634 CrossRef.
- E. Zawadzak, M. Bil, J. Ryszkowska, S. N. Nazhat, J. Cho, O. Bretcanu, J. A. Roether and A. R. Boccaccini, Polyurethane foams electrophoretically coated with carbon nanotubes for tissue engineering scaffolds, Biomed. Mater., 2009, 4, 015008–015016 CrossRef.
- D. B. Warheit, B. R. Laurence, K. L. Reed, D. H. Roach, G. A. Reynolds and T. R. Webb, Comparative pulmonary toxicity assessment of single-wall carbon nanotubes in rats, Toxicol. Sci., 2003, 77, 117–125 CrossRef.
- A. Y. okoyama, Y. S.ato, Y. Nodasaka, S. Yamamoto, T. Kawasaki, M. Shindoh, T. Kohgo, T. Akasaka, M. Uo, F. Watari and K. Tohji, Biological behavior of hat-stacked carbon nanofibers in the subcutaneous tissue in rats, Nano Lett., 2005, 5, 157–161 CrossRef.
- E. S. Place, J. H. George, C. K. Williams and M. M. Stevens, Synthetic polymer scaffolds for tissue engineering, Chem. Soc. Rev., 2009, 38, 1139–1151 RSC.
- K. Ariga, X. Hu, S. Mandal and J. P. Hill, By what means should nanoscaled materials be constructed: molecule, medium, or human, Nanoscale, 2010, 2, 198–214 RSC.
- J. M. Holzwarth and P. X. Ma, 3D nanofibrous scaffolds for tissue engineering, J. Mater. Chem., 2011, 21, 10243–10251 RSC.
- J. W. Nichol and A. Khademhosseini, Modular tissue engineering: engineering biological tissues from the bottom up, Soft Matter, 2009, 5, 1312–1319 RSC.
- F. Causa, P. A. Netti and L. Ambrisio, A multi-functional scaffold for tissue regeneration: the need to engineer a tissue analog, Biomaterials, 2007, 28, 5093–5099 CrossRef CAS.
- K. Kulangara and K. W. Leong, Substrate topography shapes cell function, Soft Matter, 2009, 5, 4072–4076 RSC.
- B. D. Ratner and S. J. Bryant, Biomaterials: Where we have been and where we are going, Annu. Rev. Biomed. Eng., 2004, 6, 41–75 CrossRef CAS.
- J. Hu and P. X. Ma, Nano-fibrous tissue engineering scaffolds capable of growth factor delivery, Pharm. Res., 2011, 28, 1273–1281 CrossRef CAS.
- J. T. Borenstein, E. J. Weinberg, B. K. Orrick, C. Sundback, M. R. Kaazempur-Mofrad and J. P. Vacanti, Microfabrication of three-dimensional engineered scaffolds, Tissue Eng., 2007, 13, 1837–1844 CrossRef CAS.
- J. El-Ali, P. K. Sorger and K. F. Jensen, Cells on chips, Nature, 2006, 442, 403–411 CrossRef CAS.
- C. M. Hwang, A. K. hademhosseini, Y. Park, K. Sun and S. H. Lee, Microfluidic chip-based fabrication of PLGA microfiber scaffolds for tissue engineering, Langmuir, 2008, 24, 6845–6851 CrossRef CAS.
- S. Chung, R. Sudo, P. J. Mack, C. R. Wan, V. Vickerman and R. D. Kamm, Cell migration into scaffolds under co-culture conditions in a microfluidic platform, Lab Chip, 2009, 9, 269–275 RSC.
- M. E. Gomes, V. I. Sikavitsas, E. Behravesh, R. L. Reis and A. G. Mikos, Effect of flow perfusion on the osteogenic differentiation of bone marrow stromal cells cultured on starch-based three-dimensional scaffolds, J. Biomed. Mater. Res., 2003, 67A, 87–95 CrossRef CAS.
- K. Domansky, W. Inman, J. Serdy, A. Dash, M. H. M. Lim and L. G. Griffith, Perfused multiwell plate for 3D liver tissue engineering, Lab Chip, 2010, 10, 51–58 RSC.
- N. W. Choi, M. Cabodi, B. Held, J. P. Gleghorn, L. J. Bonassar and A. D. Stroock, Microfluidic scaffolds for tissue engineering, Nat. Mater., 2007, 6, 908–915 CrossRef CAS.
- J. A. Burdick and G. Vunjak-Novakovic, Engineered microenvironments for controlled stem cell differentiation, Tissue Eng. A, 2009, 15, 205–219 CrossRef CAS.
- J. A. King and W. M. Miller, Bioreactor development for stem cell expansion and controlled differentiation, Curr. Opin. Chem. Biol., 2007, 11, 394–398 CrossRef CAS.
- G. Vozzi and A. Ahluwalia, Microfabrication for tissue engineering: rethinking the cells-on-a scaffold approach, J. Mater. Chem., 2007, 17, 1248–1254 RSC.
Footnote |
† These two authors made equal contributions |
|
This journal is © The Royal Society of Chemistry 2012 |