DOI:
10.1039/C2NR32311G
(Review Article)
Nanoscale, 2013,
5, 23-37
Upconversion nanoparticles and their composite nanostructures for biomedical imaging and cancer therapy
Received 16th August 2012, Accepted 8th October 2012
First published on 10th October 2012
Abstract
Upconversion nanoparticles (UCNPs), particularly lanthanide-doped nanocrystals, which emit high energy photons under excitation by the near-infrared (NIR) light, have found potential applications in many different fields, including biomedicine. Compared with traditional down-conversion fluorescence imaging, the NIR light excited upconversion luminescence (UCL) imaging relying on UCNPs exhibits improved tissue penetration depth, higher photochemical stability, and is free of auto-fluorescence background, which promises biomedical imaging with high sensitivity. On the other hand, the unique upconversion process of UCNPs may be utilized to activate photosensitive therapeutic agents for applications in cancer treatment. Moreover, the integration of UCNPs with other functional nanostructures could result in the obtained nanocomposites having highly enriched functionalities, useful in imaging-guided cancer therapies. This review article will focus on the biomedical imaging and cancer therapy applications of UCNPs and their nanocomposites, and discuss recent advances and future prospects in this emerging field.
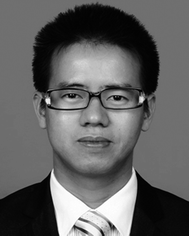 Liang Cheng | Dr Liang Cheng was born in Anhui, China. He received his PhD degree from Institute of Functional Nano & Soft Materials (FUNSOM) at Soochow University in 2012. His PhD thesis was focused on the biomedical applications of upconversion nanoparticles under the supervision of Prof. Zhuang Liu. He is now a lecturer in Prof. Liu's group at Soochow University. His current research interest is the development of multifunctional nanostructures for applications in cancer theranostics. |
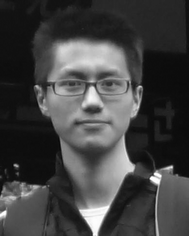 Chao Wang | Mr Chao Wang was born in Jiangsu, China, in 1987. He received his BS degree from Soochow University in 2010. Since then, he has studied as a PhD candidate under the guidance of Prof. Zhuang Liu in FUNSOM at Soochow University. His current research directions include photodynamic therapy based on UCNPs and the applications of UCNPs for cell tracking and cell therapies. |
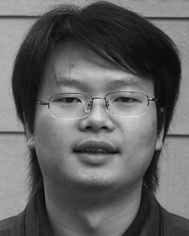 Zhuang Liu | Dr Zhuang Liu received his BS degree from Peking University (China) in 2004 and PhD degree from Stanford University (USA) in 2008. In 2009, Dr Liu joined FUNSOM at Soochow University in China as a principal investigator. Dr Liu's research in the past few years has been mostly focused on the development of functional nanomaterials including carbon nanomaterials, upconversion nanoparticles, and other composite nanostructures, for applications in biomedical imaging, drug delivery, phototherapy of cancer, and stem cell research. Starting from 2005, Dr Liu has authored over 70 peer-reviewed papers, many of which were published in top chemistry, materials and biomedicine journals (total citation > 5500 times). The awards Dr Liu received include MRS silver award in 2008 and SCOPUS young researcher award (China) in 2012. |
1. Introduction
Rare earth (RE)-containing UCNPs are able to emit high-energy photons under NIR excitation, resulting from a nonlinear optical upconversion (UC) process in which the sequential absorption of two or more photons leads to the emission of a single photon at the shorter wavelength. UC processes are mainly divided into three classes of mechanisms: excited state absorption (ESA), energy transfer upconversion (ETU), and photo avalanche (PA).1 Since its discovery in the mid-1960s,2 it has attracted wide interest because of its potential applications in many different areas, including solid state lasers,3 3D flat-panel displays,4 low-intensity IR imaging,5 and bioprobes.6–14 With the development of nanotechnology, a variety of methods have been developed to synthesize different kinds of UCNPs, many of which are lanthanide-doped nanocrystals with fine crystalline structures and controlled sizes.7,15–18 Compared with the traditionally used down-conversion fluorescent organic dyes and quantum dots (QDs), optical probes based on UCNPs have a number of advantages, such as sharp emission bandwidth, long lifetime, tunable emission, high photostability, low cytotoxicity, and importantly, little background autofluorescence, making them attractive contrast agents in optical biomedical imaging.15,19–22In the past few years, a large number of groups have investigated the potential applications of UCNPs in biomedicine.19,20,23 Nyk et al. reported in vivo whole body UCL imaging of mice injected with UCNPs for the first time.24 Using targeting ligand conjugated UCNPs, Li and coworkers achieved efficient in vivo tumor targeting and UCL imaging.25 UCNPs could be further engineered to be contrasts agents in magnetic resonance (MR) imaging, positron emission tomography (PET), and computer tomography (CT), for in vitro and in vivo multimodal imaging, as demonstrated by a number of groups.26–30 UCNPs may also be combined with anti-cancer drugs,31 photosensitizers (PS)32,33 or gold nanostructures34 for potential therapeutic applications including chemotherapy, photodynamic therapy (PDT), and photothermal therapy (PTT). Although a few recent review articles have summarized the synthesis of UCNPs and the use of UCNPs for small animal imaging, as well as for biomedical applications in general,19–21,35 to our best knowledge there is no such article which systemically discussed the therapeutic applications of UCNPs, as well as in vivo imaging-guided therapies using UCNPs and their nanocomposites. In this review paper, we will thus summarize recent progress regarding the use of UCNPs and their nanocomposites for in vivo biomedical imaging and cancer therapy, and discuss the future challenges and promises of using UCNPs as novel theranostic nano-platforms.
2. Synthesis and functionalization of UCNPs and their nanocomposites
2.1 Synthetic approaches for UCNPs
Generally speaking, UCNPs are composed of three components: a host matrix, a sensitizer and an activator. An ideal host matrix needs to have low lattice photon energies, which is a requirement to minimize non-radiative losses and maximize the radiative emission. Among the available types of UC host materials, fluorides such as NaYF4,7,15–18,36–41 NaGdF4,27,42–46 NaLuF4,47–50 KYF4,51 NaYbF4,52 LaF3,53 CaF2,54 KMnF3,55 YF3,56 KGdF4 (ref. 10) and a few others, have proved to be the ideal host candidates, due to their low photon energies and high chemical stability. Several approaches have been used to synthesize RE-doped UCNPs, including thermal decomposition method,15–18,53,57,58 hydrothermal method,59–64 co-precipitation method,65 and various others.66–71 The various approaches to synthesize UCNPs have already been summarized by a number of previously published review articles,19–21,35 and are thus only briefly summarized here in Table 1. In general, the thermal decomposition and hydrothermal synthetic methods have proved to be effective in the fabrication of monodispersed, single crystalline and phase-pure UCNPs, and thus are the two most widely adopted methods in UCNP synthesis.
Table 1 Synthesis and functionalization of UCNPs and their nanocomposites
Methods | Examples |
---|
UCNPs synthesis |
Thermal decomposition method | NaYF4, Yb, Er (Tm) (ref. 15, 16, 18, 57 and 58) |
NaGdF4, Yb, Er (Tm) (ref. 17) |
NaYbF4, Er, Tm, Ho (ref. 52) |
NaLuF4, Yb, Er (Tm) (ref. 47) |
Hydrothermal/solvothermal method | NaYF4, Yb, Er (Tm) (ref. 7) |
NaGdF4, Yb, Er (Tm) (ref. 61–63) |
NaY(Mn)F4, Yb, Er (Tm) (ref. 141) |
Other methods |
Co-precipitation method | NaYF4, Yb, Er (Tm) (ref. 65) |
Sol–gel processing | ZrO2 (Er3+) (ref. 66) |
Combustion synthesis | Y2O3, La2O2S, and Gd2O3 (ref. 68–70) |
Flame synthesis | Y2O3:Yb/Er (Tm, Ho) (ref. 71) |
Surface modifications of UCNPs |
Ligand exchange | PAA,12,34,41,72–78 PEG,15,79 HDA,80 AHA,81 TGA,82 3-MA,24,43,83 DMSA,84 citrate,85 DDA,86 MUA86 |
Ligand oxidation reaction | Lemieux-von Rudloff reagent,87,88 ozone oxidation89 |
Host–guest interaction | β-CD/Ad (ref. 91 and 92) |
Coating with amphiphilic polymers | DSPE–PEG,79 C18PMH–PEG31 |
Layer-by-layer method | PAH,7 PSS7 |
Nanocomposites based on UCNPs |
Silica coating | UCNP@SiO2 (ref. 171) |
UCNP–Dye@SiO2 (ref. 94 and 95) |
UCNP–QDs@SiO2 (ref. 95) |
UCNP–Fe3O4@SiO2 (ref. 28, 105 and 106) |
UCNP–Ag@SiO2 (ref. 100) |
Chemical conjugation & in situ growth | UCNP–QDs (ref. 97 and 98) |
UCNP–Au (ref. 99) |
UCNP–Ag (ref. 82) |
UCNP–Fe3O4 (ref. 86 and 103) |
Layer-by-layer method | UCNP–Fe3O4–Au (ref. 34 and 107) |
2.2 Surface modifications of UCNPs
UCNPs synthesized by the above-mentioned protocols are usually capped with organic ligands and thus not soluble in water. Surface modification is the critical step to fabricate UCNP-based bio-probes for various biomedical applications. To date, several approaches have been used to modify UCNPs including ligand exchange, ligand oxidation reaction, host–guest interaction, layer-by-layer (LBL) self-assembly method, and coating with amphiphilic polymers (Table 1).Ligand exchange is an important method to modify UCNPs.12,15,24,34,41,43,72–86 In this method, hydrophobic organic molecules such as oleic acid and oleylamine on the surface of as-made UCNPs are exchanged for various hydrophilic ligands, such as polyacrylic acid (PAA),12,34,41,72–78 poly(ethylene glycol) (PEG),15,79 hexanedioic acid (HDA),80 6-aminohexanoic acid (AHA),81 thioglycolic acid (TGA),82 3-mercaptopropionic acid (3 MA),24,43,83 dimercaptosuccinic acid (DMSA),84 citrate,85 10-decanedicarboxylic acid (DDA),86 and 11-mercaptoundecanoic (MUA),86 offering water soluble UCNPs which retain their morphology and crystallization. Ligand oxidation reaction is another method to render nanoparticles water-soluble and to provide reactive functional groups for subsequent bio-conjugation.87–89 However, this method is applicable only to a specific class of UCNPs capped with ligand molecules containing unsaturated carbon–carbon bonds in their long alkyl chains. Apart from ligand engineering, the self-assembly approach has also been widely used to functionalize UCNPs by coating the nanoparticles with multiple layers of polymers with counter charges (e.g. polyallylamine hydrochloride (PAH), poly sodium 4-styrenesulfonate (PSS)), or with some specially designed host (e.g. β-cyclodextrin, β-CD) and guest (e.g. adamantaneacetic acid, Ad) molecules.7,90–92 Moreover, as-synthesized hydrophobic UCNPs dispersed in the organic phase could be coated with amphiphilic polymers via hydrophobic interactions and then transferred into the aqueous phase.31,79,93 For examples, 1,2-distearoyl-sn-glycero-3-phosphoethanolamine-N-(PEG 5000) (DSPE-PEG), and poly(maleic anhydride-alt-1-octadecene)-mPEG 5000(C18PMH-PEG) have been used to functionalize UCNPs, offering PEGylated UCNPs with excellent stability in physiological solutions and improved biocompatibility.
2.3 Fabrication of UCNP-based nanocomposites
Recently, much attention has been paid to the development of UCNP-based composite nanostructures with multiple functions by coating or integrating UCNPs with other inorganic nanoscale components (Table 1). Silica coating on UCNPs is one of the most popular techniques for the fabrication of nanocomposites,32,94,95 not only to facilitate surface coating with biocompatible polymers and bioconjugation,94 but also to produce more functionalities in the obtained nanocomposites. The size of the coated silica layer can be controlled by adjusting the concentration of added tetraethoxysilane (TEOS), a precursor for silica formation by hydrolysis and polycondensation. Silica is biocompatible and its surface can be easily modified with amines, thiols, and carboxyl groups, allowing the linking of biomolecules. Li et al. prepared core–shell structured UCNP nanospheres with a thin and uniform silica coating,95 into which organic dyes or QDs are encapsulated to tune the UCL spectra by fluorescence resonance energy transfer (FRET). Therapeutic agents such as photosensitizers can also be encapsulated within the silica shell grown on UCNPs for applications in photodynamic therapy.32,96UCNPs may also be coupled with other functional nanomaterials.97 Kim and coworkers were able to couple QDs with UCNPs, and used the obtained nanocomposites for multiplexed NIR excited in vivo imaging.98 Zhang et al. reported a new approach to modulate the UC emission through plasmonic interactions between UCNPs and the attached gold nanostructures, and observed enhanced UCL emission by coating UCNPs with a gold shell of appropriate thickness.99 Stucky at al. reported a facile method to fabricate Ag@SiO2@UCNP nanomaterials based on a core–spacer–shell approach.100 The UC fluorescence enhancements and quenching could be tuned by the thickness of the dielectric silica spacer. Song and coworkers synthesized highly crystalline, monosized core–shell UCNP@Ag nanocomposites with multiple functionalities and used them as a useful photothermal therapy agent, as well as an UC imaging label.82
Super-paramagnetic iron oxide nanoparticles (IONP) have been widely applied in MR imaging.101,102 Shen et al. have developed a cross-linker anchoring process to synthesize Fe3O4–UCNPs hetero-nanoparticles using DDA or MUA as the cross-linker.86 Mi et al. synthesized magnetic/UCNPs multifunctional nanoparticles by covalently linking multiple carboxyl-functionalized IONPs onto individual amino-functionalized silica-coated UCNPs.103 Fe3O4 could also be directly grown on the surface of NaFY4-based UCNPs as reported by Li and coworkers for dual model UCL and MR imaging.104 Very recently, several groups have developed a new strategy to synthesize multifunctional nanoparticles based on UCNPs and IONPs using a silica coating method,104 such as core–shell Fe3O4@nSiO2@mSiO2@UCNPs nanostructures by Lin and coworkers,28 Fe3O4@SiO2@UCNPs nanocomposite by Chen group,105 and Fe3O4&UCNPs@SiO2 by Shi's group.106 The nanocomposites based on silica modified UCNPs showed excellent super-paramagnetic properties and strong UCL emissions, which are useful for in vitro and in vivo imaging. Beyond those, our group used a LBL method to synthesize a novel class of multifunctional nanoparticles (MFNPs) containing a UCNP as the core, closely packed IONPs as the inter-layer, and a thin layer of gold as the shell.34 The obtained UCNP@IONP@Au MFNPs were then coated with PEG via the Au–thiol bond and used for in vitro and in vivo multimodal imaging and therapy.34,107
3. UCNPs and their nanocomposites for in vivo biomedical imaging
As stated in the introduction section, UCNPs have been developed as a new class of fluorescence bio-probes owing to their many intriguing advantages. Since 1999, several groups have started using UCNPs as optical nano-probes for in vitro cell imaging,22,88,108–111 which, however, is not the focus of this review article and will not be discussed in detail. In 2006, Lim et al. studied the use of UCNPs in live organism imaging.112 Upon excitation at 980 nm, the distribution of UCNPs inside live nematode Caenorhabditis elegans could be clearly visualized. In 2008, Nyk et al. for the first time demonstrated in vivo imaging of a BALB/c mouse injected with UCNPs,24 which showed high uptake in the liver and spleen after intravenous injection. Compared with traditional down-conversion fluorescence imaging using QDs or organic dyes, the UCNP-based UCL imaging has no autofluorescence. In a later work, we compared the in vivo imaging sensitivity of the UCNPs and QDs (QD545 and QD625) in a side-by-side experiment79 (Fig. 1). Due to the autofluorescence-free nature of the UCL imaging, a long exposure time was employed in UCL imaging without introducing any obvious background, allowing the in vivo detection limit of UCNPs to be at least one order of magnitude lower than that of QDs using our imaging system. In recent years, UCNPs have thus been widely used for in vivo multicolor imaging, tumor-targeted imaging, multimodal imaging, and in vivo cell tracking.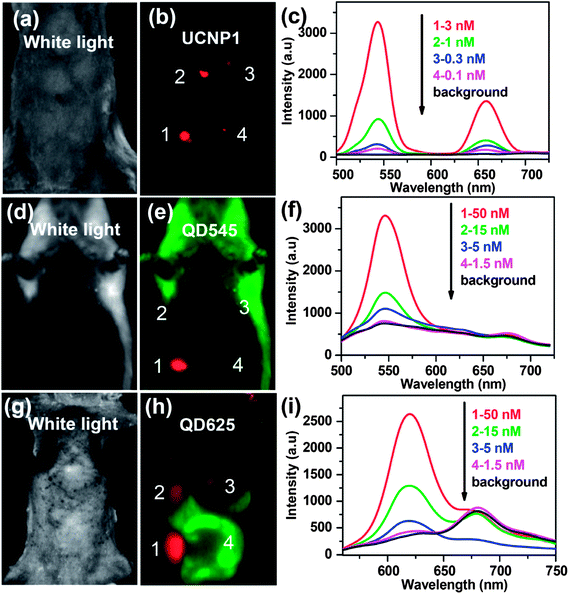 |
| Fig. 1 Comparison of imaging sensitivities between UCNPs and QDs. (a, d, and g) White light images of mice subcutaneously injected with various concentrations of UCNP solutions and QD solutions. (b, e, and h) In vivo images of the injected mice. (c, f, and i) UCL emission spectra and fluorescence spectra recorded at the injection sites. Copyright 2010, Springer.79 | |
3.1 Multicolor imaging
By varying the Ln3+ dopants during UCNP synthesis, the UCL emission spectra of nanoparticles could be well tuned, enabling multicolor UCL imaging in biological systems.16,52,113–115 In a study by our group, we synthesized a series of PEGylated UCNPs (NaY0.78Yb0.2Er0.02F4, NaY0.69Yb0.3Er0.01F4, NaY0.78Yb0.2Tm0.02F4) with different UCL emission spectra79 (Fig. 2). On 980 nm laser excitation, three types of UCNPs were easily differentiated after spectral deconvolution. Multicolor in vivo UCL imaging was demonstrated by imaging subcutaneously injected UCNPs and applied in multiplexed in vivo lymph node mapping as well as multicolor in vivo cell labeling and tracking.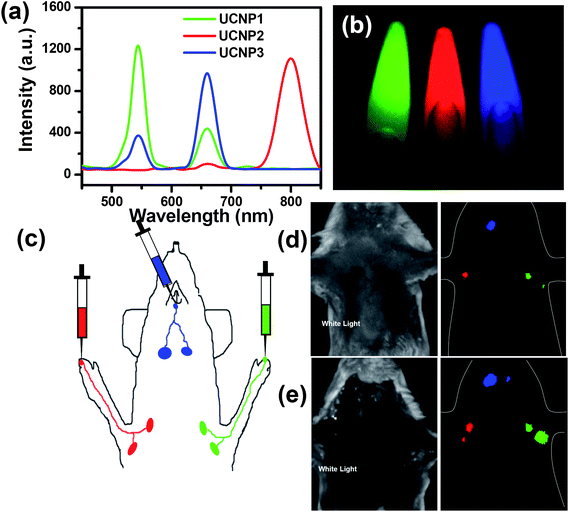 |
| Fig. 2 Multicolor UCL imaging. (a) UCL emission spectra of three UCNP solutions under 980 nm NIR laser excitation. (b) A multicolor UCL image of three solutions obtained by in vivo imaging system (CRi, Inc.) after spectral unmixing. (c) A schematic illustration of UCNP based multi-color lymph node mapping. (d) White light and in vivo UCL images of a mouse injected with UCNPs. (e) White light and UCL images of the same mouse after dissection. Copyright 2010, Springer.79 | |
FRET is another approach to modulate the UCL spectra of UCNPs for multi-color imaging. In 2008, Zhang et al. fabricated the UCNP–silica core–shell structure by encapsulating organic dyes or QDs into the silica shell.95 The upconversion emission spectra under 980 nm excitation were tuned by FRET from the UCNP core to organic dyes or QDs inside the silica shell, generating UCNP-composites with different UCL ‘colors’. In our recent work, we showed that PEGylated UCNPs physically loaded with organic dyes via hydrophobic force could also serve as a FRET nanosystem for in vivo multicolor UCL imaging.116 As many as five UCL ‘colors’ could be clearly separated after spectral deconvolution in the imaging of mice.
3.2 Tumor-targeted molecular UCL imaging
Tumor-targeted molecular imaging plays an important role in tumor diagnosis and prognosis. Recently, much attention has been paid to investigating in vivo targeted imaging using UCNP-based nano-probes.25,117–119 In 2009, Li and co-workers realized in vivo tumor targeting for the first time, using folic acid (FA) modified UCNPs and argine-glycine-asparatic (RGD) peptide conjugated UCNPs in their two separate studies.25,118 After intravenous injection of FA-modified UCNPs into HeLa tumor-bearing nude mouse over a day, obvious UCL signals were observed in the tumor, while no significant luminescence was observed in the control group. The RGD peptide has a high binding affinity with the αvβ3 integrin receptor which plays a pivotal role in tumor angiogenesis. Xiong et al. showed that PEGylated UCNPs conjugated with RGD could effectively target U87 MG human glioblastoma tumors in mice as revealed by in vivo UCL imaging 25 (Fig. 3). In a later work, Yu et al. reported the development of neurotoxin-mediated UCNP nanoprobes for tumor targeting and visualization in living animals.120 The nanoprobes were synthesized by conjugating polyethylenimine (PEI) coated UCNPs with recombinant chlorotoxin, a typical peptide neurotoxin which could bind to many types of cancer cells with high specificity. Those UCNP-based nano-probes with high imaging sensitivity and specific tumor-binding ability may have unique potential in molecular cancer imaging in future explorations.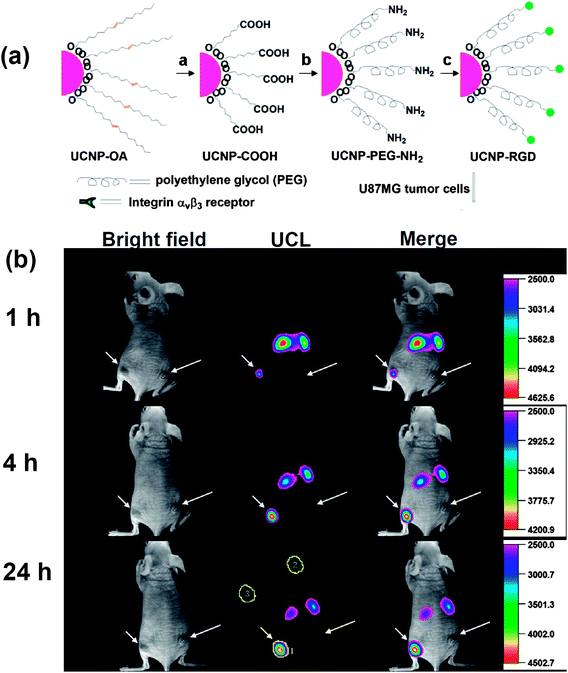 |
| Fig. 3 In vivo tumor-targeted UCL imaging. (a) A scheme showing synthesis of UCNP–RGD. (b) Time-dependent in vivo UCL images of a mouse bearing a U87MG tumor (left hind leg, indicated by short arrows) and a MCF-7 tumor (right hind leg, indicated by long arrows) after intravenous injection of UCNP-RGD. Copyright 2009, American Chemical Society.25 | |
3.3 Multimodal imaging of UCNPs
Multimodal imaging, which combines several different imaging modalities to overcome the limitations of each single imaging approach, has received tremendous attention in the area of in vivo biomedical imaging.121,122 Recently, great efforts have been made to obtain multimodal imaging probes based on UCNPs and their nanocomposites.26,27,30,34,42–44,123,124Gadolinium (Gd3+) is a paramagnetic relaxation agent extensively used in MR imaging. By doping light-emitting rare earth ions into a Gd3+ containing host matrix, such as NaGdY4, many groups synthesized Gd-based UCNPs that offered both UCL emission and T1-weighted MR contrast. In a representative example, Hyeon and coworkers demonstrated the use of PEG–phospholipid functionalized NaGdF4:Yb/Er nanoparticles for optical and MR imaging in breast cancer cells (SK-BR3) for the first time.42 Combined optical and MR imaging of cells using NaGdF4:Yb/Er nanocrystals was also reported by Kumar et al.43 However, the NaGdF4:Yb/Er nanoparticles were only used for in vitro multimodal imaging of cells in these two studies. Starting from 2011, Li's group published a series of studies on in vivo multimodal imaging with UCNPs in small animals.26,30,125,126 They first used hydrophilic azelaic acid functionalized NaGdF4 UCNPs as a dual-modal in vivo imaging probe for combined UCL and MR imaging in mice.125 After that, 18F-labeled NaGdF4, Yb/Er UCNPs were further fabricated and used for triple-modal PET, UCL, and MR imaging in animal experiments26,30 (Fig. 4). Several other related approaches to develop UCNP-based multimodal imaging probes have also been reported by Li's group,127 Cui's group29 and Shi's group.27 Shi and coworkers reported the combination of Gd-doped UCNPs with Au nanoparticles based on a simple electrostatic adsorption mechanism, resulting in a sub-50 nm sized multifunctional nanostructure which was useful for UCL, MR and CT tri-modal imaging modalities.27.
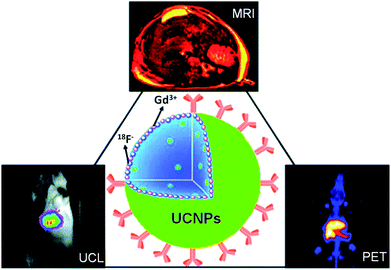 |
| Fig. 4 A scheme showing the use of 18F-labeled Gd-containing UCNPs for in vivo multimodal UCL, PET and MR imaging. Copyright 2011, American Chemical Society.30 | |
Besides Gd-based UCNPs, several groups have also developed IONP–UCNP nanocomposites for multimodal T2-weighted MR and UCL imaging. Lin's group proposed a novel and facile strategy for the fabrication of multifunctional Core–shell Fe3O4@nSiO2@mSiO2@UCNP nanostructures using silica coated Fe3O4 spheres as the core, mesoporous silica as the inner-shell, and deposited UCNPs as the outer shell.28 Shi's group developed a “neck-formation” strategy to combine IONPs and UCNPs into hetero-nanoparticles by silica-shielding for both T2-weighted MR and UCL imaging.106 Wu reported a facile method for the fabrication of core–shell structured UCNP@SiO2@Fe3O4 nanocomposite particles with good superparamagnetic and luminescent properties for cell imaging.128 Li and coworkers developed a step-wise synthetic method to synthesize core–shell Fe3O4@UCNP nanostructures with multifunctional properties for multimodal imaging.127 Recently, our group developed a novel class of MFNPs based on UCNP@IONP@Au with combined optical and magnetic properties useful in multi-modality in vitro and in vivo imaging.107
3.4 UCNPs for cell labeling and in vivo tracking
Owing to the auto-fluorescence free nature during UCL imaging, UCNPs have also been used in cell labeling and in vivo tracking with great sensitivity. In an earlier work, Zhang's reported the use of silica coated UCNP to label myoblasts cells for in vivo dynamic tracking in mice.129 In 2010, we used UCNPs as a novel class of cell labeling agents for in vivo tracking and multi-color imaging of cancer cells also in mice.79 In a recent study, Li and coworkers fabricated sub 10 nm hexagonal NaLuF4:Gd3+, Yb3+, Tm3+ with strong UCL emission to label cancer cells. Excellent in vivo tracking sensitivities with detection limits down to 50 and 1000 UCNP-labeled cells after subcutaneous and intravenous injection, respectively, were achieved in this work47 (Fig. 5a and b).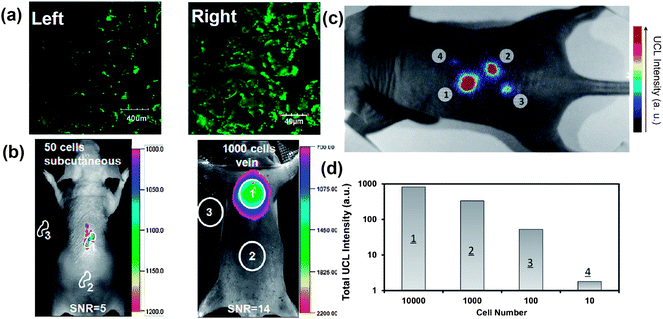 |
| Fig. 5 UCNPs for cell labeling and in vivo tracking. (a) Confocal UCL image (Left) and its overlay with a bright field image (Right) of cells stained with 200 μg ml−1 NaLuF4 UCNPs for 3 h at 37 °C. (b) In vivo UCL imaging of athymic nude mice after subcutaneous injection of 50 human nasopharyngeal epidermal carcinoma KB cells (left) and tail-vein injection of 1000 KB cells (Right). The KB cells were pre-incubated with 200 μg ml−1 NaLuF4 UCNPs for 3 h at 37 °C before injection. (c and d) In vivo detection of UCNP-labeled mMSCs. (c) A UCL image of a mouse subcutaneously injected with various numbers of mMSCs (10 × 104) labeled with UCNPs. (d) Quantification of UCL signals in (c). Copyright 2011, American Chemical Society47 and 2012, Elsevier.134 | |
In the recent years, mesenchymal stem cells (MSCs) have been extensively explored for potential applications in tissue engineering, immunotherapy, and gene therapy because they can differentiate into a variety of cell types including fat, bone, and cartilage under suitable conditions.130–133 Owing to the above mentioned unique optical properties, a number of groups, including ours, have used UCNPs to label stem cells for in vitro and in vivo tracking.111,134 In our recent study, we used UCNPs as an exogenous contrast agent to track mouse MSCs (mMSCs) in vivo. Oligo-arginine was conjugated to PEG coated UCNPs to enhance the uptake of nanoparticles by mMSCs134 (Fig. 5c). It was found that the proliferation and differentiation of mMSCs were not notably affected by UCNP-labeling. Thanks to the absence of auto-fluorescence during UCL imaging, we were able to detect as few as ∼10 cells injected into a mouse, achieving an ultra-high sensitivity which is many orders of magnitude higher than currently used exogenous stem cell labeling nano-agents, such as QDs and IONPs (Fig. 5d). In vivo translocation of UCNP-labeled mMSCs after intravenous injection could be well monitored by UCL imaging. In our latest study, we further used multifunctional UCNP@IONP@Au composite MFNPs previously developed for labeling and in vivo multimodal tracking of mMSCs.135 More interestingly, by utilizing the strong magnetism of those MFNPs, we discovered that MFNP-labeled mMSCs were able to specifically migrate towards a wound near a magnet, offering enhanced tissue repairing efficacy. Thus, UCNPs and their nanocomposites show great potential in highly sensitive stem cell tracking and manipulation, with promise for future imaging-guided cell therapy applications.
4. UCNPs and their nanocomposites for therapeutic applications and imaging-guided cancer therapies
Besides biomedical imaging, UCNPs and their nanocomposites have also attracted increasing attention for therapeutic applications, including chemotherapy, photodynamic therapy, and photothermal therapy. The imaging capability of UCNPs may be further utilized during cancer treatment, for imaging-guided therapies aiming at personalized medicine, as well as real-time monitoring of therapeutic responses.136,1374.1 Drug and gene delivery
Various kinds of nanomaterials with unique properties and functions have been used as nano-carriers of drugs and genes.138–140 Recently, UCNPs combined with other functionalities have also been explored for multifunctional biomedical imaging and drug delivery.31,93,141–144 In our study, we showed that UCNPs could be loaded with chemotherapy drug molecules for imaging and therapy31 (Fig. 6). It was found that an anti-cancer drug, doxorubicin (DOX), was physically adsorbed on the surface of PEGylated UCNPs via hydrophobic interactions and could be released under slightly acidic pH. Targeted drug delivery was realized by conjugating UCNPs with targeting ligands such as folic acid (FA). In another work, Lin's team reported a new controlled drug release system by coating UCNPs with a smart hydrogel poly(N-isopropylacrylamide-co-(methacrylic acid)) shell.142 It was discovered that the drug release behavior was pH-triggered as well as thermally sensitive. Change of pH to a mildly acidic condition at the physiological temperature could deform the shell structure and induce the release of loaded DOX from the microspheres.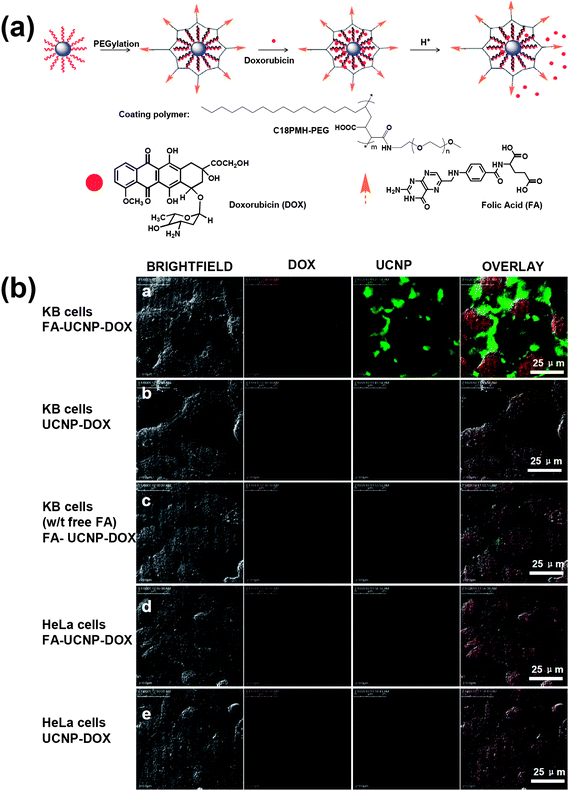 |
| Fig. 6 UCNPs for cell imaging and drug delivery. (a) A schematic illustration of the UCNP-based drug delivery system showing the loading and release of DOX from UCNPs. (b) Confocal images of folate receptor (FR) positive KB cells and FR negative HeLa cells incubated with DOX loaded UCNPs with FA conjugation (FA–UCNP–DOX) or without FA conjugation (UCNP–DOX). Copyright 2011, Elsevier.31 | |
Gene therapy by transfecting selected cells of interest with gene-encoding DNA or small interfering RNA (siRNA) has shown great promise in treating various gene-related diseases, including cancer.145,146 However, safe, efficient and preferably trackable, as well as controllable, gene delivery carriers remain to be developed in this area. In two studies by Zhang and coworkers, dye-conjugated siRNA was attached to UCNPs functionalized with anti-Her2 antibody for targeted delivery.147,148 The occurrence and disappearance of FRET between UCNPs and dye molecules on siRNA provided an interesting approach for real-time monitoring of siRNA release from nanoparticles in live cells. Recently, the same group designed a rather smart strategy to remotely control gene expression by linking photocaged compounds onto NIR-to-UV UCNPs.149 Under NIR excitation, those UCNPs emitted UV light, which could then activate photocaged plasmid DNA or siRNA to induce specific gene expression or down-regulation, respectively. Compared to traditional light-controllable gene therapy using UV light, the use of UCNPs and NIR excitation is anticipated to increase not only the depth of penetration, but also its associated therapeutic efficiency.
4.2 PDT
PDT is known as a non-invasive medical method to treat diseases such as cancer. Upon excitation by light with appropriate wavelengths, the activation of the PS molecules in the presence of oxygen is able to produce singlet oxygen or reactive oxygen species to kill nearby cancer cells. Current PDT uses visible or even UV light to excite photosensitizers, and thus suffers from the rather limited light-penetration in biological tissues.Under NIR light excitation, UCNPs are able to emit visible light, which can activate surrounding PS molecules to produce singlet oxygen and kill cancer cells. Several groups, including ours, have presented this strategy and shown the promise of UCNP-based PDT for cancer destruction.32,33,150,151 As early as 2007, Zhang's team coated UCNPs with a porous, thin layer of silica doped with merocyanine-540 PS. The obtained nanostructure was functionalized with a tumor targeting antibody for targeted PDT cancer cell killing under NIR light, which displayed deeper tissue penetration compared to visible light induced PDT.152 In later studies by Zhang and co-wokers, zinc phthalocyanine (ZnPC) PS was attached on the surface of UCNPs96 (Fig. 7a). Incident NIR laser light was upconverted by UCNPs into red light, which excited PS ZnPC to produce singlet oxygen from dissolved molecular oxygen in the micro-environment. Mesoporous silica coated UCNPs with PS molecules incorporated into the silica shell have also been developed by a few different groups for NIR light induced PDT, showing encouraging in vitro cancer killing results.32,151,153 In a recent study, Liu et al. covalently bonded Rose Bengal (RB), another PS molecule, to UCNPs.154 Compared with the generally adopted adsorption approach, both the PS loading capacity and the energy transfer efficiency from nanoparticles to PS were significantly improved. Simultaneous UCL imaging and PDT treatment were demonstrated in vitro using this UCNP–PS nanoplatform.
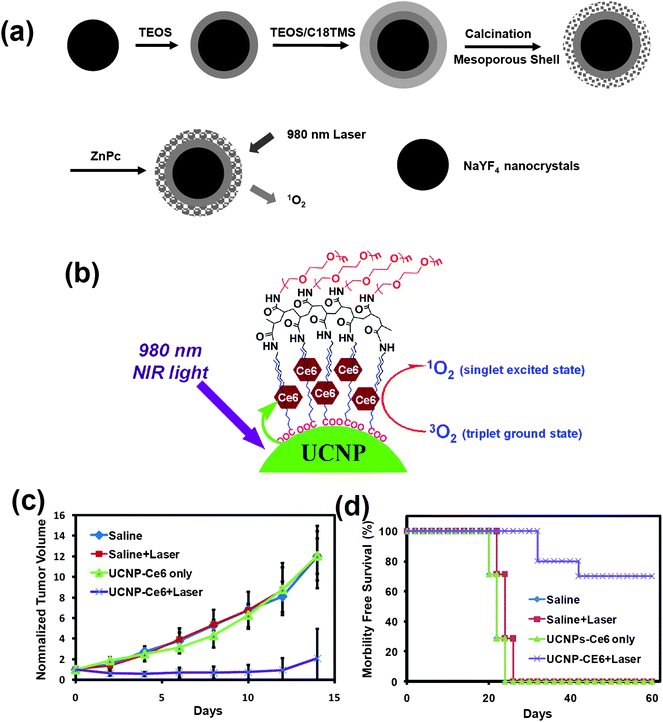 |
| Fig. 7 Photodynamic therapy based on the UCNP–PS system. (a) A schematic illustration showing mesoporous silica shelled UCNPs loaded with ZnPC for use in NIR-induced PDT. (b) A schematic drawing showing the PEGylated UCNP–Ce6 nanocomplex. (c and d) In vivo PDT treatment of tumor-bearing mice. The growth of 4T1 tumors (c) and the survival of mice (d) after the various indicated treatments were recorded every other day. Ten mice were used for each group. Copyright 2009, Wiley-VCH32 & Copyright 2011, Elsevier.33 | |
In recent work by our group, we discovered that chlorine 6 (Ce6), a PS molecule, could be adsorbed onto PEGylated UCNPs, forming a UCNP–Ce6 nanocomplex33 (Fig. 7b), which was able to enter cancer cells and induce obvious cell death after NIR light irradiation. For the first time, we realized highly efficient NIR induced PDT treatment of tumors in a mouse model upon intratumoral injection of UCNP–Ce6, followed by NIR light exposure (Fig. 7c and d). It was found that 70% of tumors were completely eliminated after UCNP-based PDT treatment, without showing re-growth within 2 months. We also observed gradual clearance of injected UCNPs from mice, without noticing any appreciable toxicity to the treated animals. The penetration depths of the NIR-induced and visible light-induced PDT was compared side-by-side. Remarkably, the NIR light-triggered PDT using UCNP–Ce6 showed a much deeper tissue penetration depth than direct excitation of Ce6 by visible light, as evidenced by both in vitro and in vivo experiments. Our results suggest that UCNP-based PDT induced by NIR light may have great potential in photodynamic cancer treatment, especially in treating large or internal tumors. In vivo PDT using UCNP–PS nanocomplexes has also been demonstrated by Gu's group in a later work,155 again achieving encouraging therapeutic effect.
UCNP-based in vivo PDT upon systemic administration has also been reported in two very recent studies. Hyeon and co-workers developed a novel theranostic probe by loading Ce6 on PEGylated UCNPs via both physical adsorption and covalent conjugation, obtaining UCNP–Ce6 conjugate for simultaneous in vivo dual-modal imaging and PDT.156 Both UCL and MR imaging uncovered that UCNP–Ce6 nanoparticles were readily accumulated in the tumor after intravenous injection, as a result of the enhanced permeability and retention (EPR) effect. On irradiation by a 980 nm laser, the tumor growth on UCNP–Ce6 injected mice was significantly inhibited. Almost simultaneously, Zhang's team157 used mesoporous silica coated UCNPs to load two types of PS molecules, ZnPc and merocyanine 540 (MC540), whose absorbance peaks overlapped with the two UCL emission peaks of UCNPs. Compared with UCNP–PS systems loaded with a single type of PS molecules, those ZnPc/MC540 co-loaded UCNPs offered greater PDT efficacy, as demonstrated by the enhanced generation of singlet oxygen and reduced cell viability after NIR laser irradiation. Importantly, by conjugating ZnPc/MC540 co-loaded UCNPs with folic acid (FA), in vivo targeted PDT treatment of melanoma tumors was demonstrated in their animal experiments, showing notably delayed tumor growth after intravenous injection of nanoparticles and NIR laser treatment. This is the first proof-of-concept study of using UCNPs for tumor-specific targeted PDT treatment, and promises further exploration of this approach for photodynamic cancer treatment.
4.3 PTT
PTT is a treatment regime involving irradiation of diseased tissue with electromagnetic radiation to cause thermal damage. The spatial specificity and minimal-invasiveness make PTT an attractive therapeutic model, as compared to surgery or other invasive therapeutic procedures.158–160 Recently, a variety of nanomaterials, such as different gold nanostructures,161–163 carbon based nanomaterials (e.g. carbon nanotubes, nano-graphene),164,165 palladium nanosheets,166 and even organic nanoparticles containing conductive polymers,90,167 which all show high absorbance in the tissue transparent NIR optical window, have been widely explored as photothermal agents for PTT ablation of cancer.MFNPs based on UCNPs have been also synthesized and used for imaging guided PTT. In a recent study, Song and coworkers reported UCNP@Ag nanocomposites used for photothermal ablation of HepG2 cells and BCap-37 cells upon exposure to 980 nm NIR light.82 This class of core–shell nanoparticles is expected to be an attractive therapeutic agent for tumor ablation with bio-imaging and thermal detection in real time. We also developed a novel class of MFNPs based on UCNPs with combined optical and magnetic properties useful in multimodality imaging and therapy,34via a LBL self-assembly strategy followed by seed-induced gold shell growth. Those UCNP@IONP@Au MFNPs were successfully used for in vivo dual-modal imaging guided and magnetically targeted PTT107 (Fig. 8). By placing a magnet close to the tumor, MFNPs intravenously injected into mice would accumulate in the tumor region by magnetic attraction as revealed by dual modal UCL and MR imaging. Owing to the strong NIR optical absorption of those Au shelled MFNPs and the highly efficient magnetic tumor targeting, tumors on mice after MFNP-injection and magnetic tumor-targeting were completely eliminated upon exposure to the 808 nm NIR laser. Our work highlights the promise of using UCNP-based multifunctional nanostructures for novel cancer theranostics.
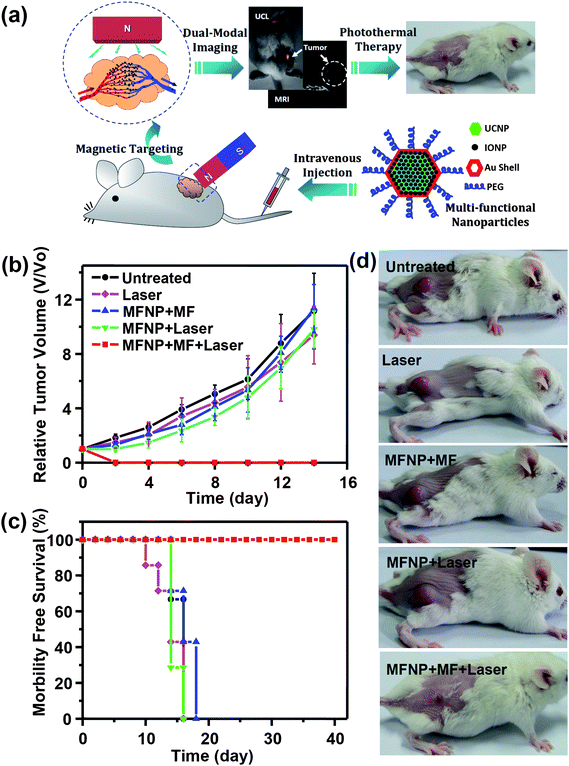 |
| Fig. 8 Multifunctional composite nanoparticles used for imaging-guided cancer therapy. (a) A schematic illustration showing the composition of a PEGylated MFNPs (MFNP-PEG) and the concept of in vivo imaging-guided magnetically targeted photothermal therapy. (b–d) In vivo magnetically targeted photothermal therapy. (b) The growth of 4T1 tumors in different groups of mice after treatment. (c) Survival curves of mice bearing 4T1 tumors after various indicated treatments. MFNP–PEG injected mice with magnetic tumor-targeting after PTT treatment survived over 40 days without any single death. (d) Representative photos of mice after various indicated treatments. Copyright 2012, Elsevier.107 | |
5. Summary and outlook
This review discusses the recent developments of UCNPs, focusing on their applications in bio-imaging and therapy. Despite the tremendous number of exciting results reported in the past few years in this field, there are still many challenges ahead in applications of UCNP-based imaging and therapy in the clinic.First of all, the quantum yield (QY) of UCL emission of UCNPs is usually less than 1%, and becomes even lower as their sizes reduce, significantly limiting the use of these nano-probes in optical imaging and photodynamic therapy.168 The development of core–shell structures during UCNP synthesis has proved able to enhance the UCL emissions of those nanoparticles.44,169 Recently, Li and coworkers reported ultra-small NaLuF4 nanoparticles with a QY of 0.47 ± 0.06% under continuous-wave excitation. They suggested that NaLuF4 appeared to a better host matrix to fabricate brighter UCNPs compared to the traditionally used NaYF4. However, further increasing the QY of UCNPs while maintaining their small sizes, which may be preferred for a number of in vivo applications, still requires tremendous future efforts.
Secondly, the potential long-term toxicity of Ln3+ doped UCNPs is another significant concern. Although previous studies have shown that functionalized NaYF4-based UCNPs with biocompatible coatings (e.g. PEG) appear to be safe to cells at certain concentration ranges and not obviously toxic to the treated animals over months,72,111,170 the effects this class of nanomaterials would have on animals over an even longer time period, how they interact with immune systems, and whether they may interfere with the reproductive system and affect the next generation, remain essentially unknown. Much more systematic investigations are still demanded before those nanomaterials can finally be used in clinics.
Thirdly, the effects of surface modifications and size on the in vivo behaviors of UCNPs also need more investigation. Most of the currently used UCNPs would accumulate in reticuloendothelial systems (RES), such as the liver and spleen, after systemic administration. Optimization of surface coatings and sizes of UCNPs is required to improve their pharmacokinetics and tumor targeting, as well as to reduce their RES retention and potential toxicity.
Lastly, though multimodal imaging and therapies based on UCNPs and their nanocomposites have been demonstrated in several studies, better design of novel multifunctional agents based on UCNPs for simultaneous medical diagnosis and combined cancer therapies is still needed in the future in order to realize real-time monitoring of treatment progress, as well as achieving synergistic therapeutic effects.
Despite the above challenges, UCNPs with unique physical properties do indeed have significant potential in biomedicine. The absence of auto-fluorescence, which enables ultra-sensitive in vivo tracking and imaging, as well as the ability to trigger phototherapies under highly penetrating NIR light are likely two of the most attractive features of applying UCNPs in imaging and therapies. Future advances in this field require the synergistic efforts of scientists from both materials science and clinical medicine to further push forward the potential clinical theranostic applications of UCNPs.
Acknowledgements
This work was partially supported by the National Basic Research Programs of China (973 Program) (2012CB932600, 2011CB911002), the National Natural Science Foundation of China (51002100), and a Project Funded by the Priority Academic Program Development of Jiangsu Higher Education Institutions.References
- M.-F. Joubert, Opt. Mater., 1999, 11, 181 CrossRef CAS.
- F. Auzel, Chem. Rev., 2004, 104, 139 CrossRef CAS.
- T. Sandrock, H. Scheife, E. Heumann and G. Hube, Opt. Lett., 1997, 22, 808 CrossRef CAS.
- E. Downing, L. Hesselink, J. Ralston and R. Macfarlane, Science, 1996, 273, 1185 CAS.
- S. Shionoya and W. M. Yen, Phosphor Handbook, 1999 Search PubMed.
- F. v. d. Rijke, H. Zijlmans, S. Li, T. Vail, A. K. Raap, R. S. Niedbala and H. J. Tanke, Nat. Biotechnol., 2001, 19, 273 CrossRef.
- L. Wang, R. Yan, Z. Huo, L. Wang, J. Zeng, J. Bao, X. Wang, Q. Peng and Y. Li, Angew. Chem., Int. Ed., 2005, 44, 6054 CrossRef CAS.
- M. Wang, W. Hou, C.-C. Mi, W.-X. Wang, Z.-R. Xu, H.-H. Teng, C.-B. Mao and S.-K. Xu, Anal. Chem., 2009, 81, 8783 CrossRef CAS.
- J. Liu, Y. Liu, Q. Liu, C. Li, L. Sun and F. Li, J. Am. Chem. Soc., 2011, 133, 15276 CrossRef CAS.
- Q. Ju, D. Tu, Y. Liu, R. Li, H. Zhu, J. Chen, Z. Chen, M. Huang and X. Chen, J. Am. Chem. Soc., 2012, 134, 1323 CrossRef CAS.
- Q. Liu, J. Peng, L. Sun and F. Li, ACS Nano, 2011, 5, 8040 CrossRef CAS.
- C. Liu, Z. Wang, H. Jia and Z. Li, Chem. Commun., 2011, 47, 4661 RSC.
- F. Zhang, Q. Shi, Y. Zhang, Y. Shi, K. Ding, D. Zhao and G. D. Stucky, Adv. Mater., 2011, 23, 3775 CAS.
- Y. Wang, P. Shen, C. Li, Y. Wang and Z. Liu, Anal. Chem., 2012, 84, 1466 CrossRef CAS.
- G. S. Yi and G. M. Chow, Adv. Funct. Mater., 2006, 16, 2324 CrossRef CAS.
- F. Wang and X. Liu, J. Am. Chem. Soc., 2008, 130, 5642 CrossRef CAS.
- F. Wang, Y. Han, C. S. Lim, Y. Lu, J. Wang, J. Xu, H. Chen, C. Zhang, M. Hong and X. Liu, Nature, 2010, 463 Search PubMed.
- H. Mai, Y. Zhang, R. Si, Z. Yan, L. Sun, L. You and C. Yan, J. Am. Chem. Soc., 2006, 128, 6426 CrossRef CAS.
- J. Zhou, Z. Liu and F. Li, Chem. Soc. Rev., 2012, 41, 1323 RSC.
- D. K. Chatterjee, M. K. Gnanasammandhan and Y. Zhang, Small, 2010, 6, 2781 CrossRef CAS.
- F. Wang, D. Banerjee, Y. Liu, X. Chen and X. Liu, Analyst, 2010, 135, 1839 RSC.
- S. Wu, G. Han, D. J. Milliron, S. Aloni, V. Altoe, D. V. Talapin, B. E. Cohen and P. J. Schuck, Proc. Natl. Acad. Sci. U. S. A., 2009, 106, 10917 CrossRef CAS.
- F. Wang and X. Liu, Chem. Soc. Rev., 2009, 38, 976 RSC.
- M. Nyk, R. Kumar, T. Y. Ohulchanskyy, E. J. Bergey and P. N. Prasad, Nano Lett., 2008, 8, 3834 CrossRef CAS.
- L. Xiong, Z. Chen, Q. Tian, T. Cao, C. Xu and F. Li, Anal. Chem., 2009, 81, 8687 CrossRef CAS.
- J. Zhou, M. Yu, Y. Sun, X. Zhang, X. Zhu, Z. Wu, D. Wu and F. Li, Biomaterials, 2011, 32, 1148 CrossRef CAS.
- H. Xing, W. Bu, S. Zhang, X. Zheng, M. Li, F. Chen, Q. He, L. Zhou, W. Peng, Y. Hua and J. Shi, Biomaterials, 2012, 33, 1079 CrossRef CAS.
- S. Gai, P. Yang, C. Li, W. Wang, Y. Dai, N. Niu and J. Lin, Adv. Funct. Mater., 2010, 20, 1166 CrossRef CAS.
- M. He, P. Huang, C. Zhang, H. Hu, C. Bao, G. Gao, R. He and D. Cui, Adv. Funct. Mater., 2011, 21, 4470 CrossRef CAS.
- Q. Liu, Y. Sun, C. Li, C. Li, J. Zhou, C. Li, T. Yang, X. Zhang and F. Li, ACS Nano, 2011, 5, 3146 CrossRef CAS.
- C. Wang, L. Cheng and Z. Liu, Biomaterials, 2011, 32, 1110 CrossRef CAS.
- H. S. Qian, H. C. Guo, P. C.-L. Ho, R. Mahendran and Y. Zhang, Small, 2009, 5, 2285 CrossRef CAS.
- C. Wang, H. Tao, C. Liang and L. Zhuang, Biomaterials, 2011, 32, 6145 CAS.
- L. Cheng, K. Yang, Y. Li, J. Chen, C. Wang, M. Shao, S.-T. Lee and Z. Liu, Angew. Chem., Int. Ed., 2011, 50, 7385 CrossRef CAS.
- M. Wang, G. Abbineni, A. Clevenger, C. Mao and S. Xu, Nanomed.: Nanotechnol., Biol. Med., 2011, 7, 710 CrossRef CAS.
- J.-C. Boyer, F. Vetrone, L. A. Cuccia and J. A. Capobianco, J. Am. Chem. Soc., 2006, 128, 7444 CrossRef CAS.
- X. Liang, X. Wang, J. Zhuang, Q. Peng and Y. Li, Adv. Funct. Mater., 2007, 17, 2757 CrossRef CAS.
- L. Wang, Y. Zhang and Y. Zhu, Nano Res., 2010, 3, 317 CrossRef CAS.
- F. Zhang, Y. Wan, T. Yu, F. Zhang, Y. Shi, S. Xie, Y. Li, L. Xu, B. Tu and D. Zhao, Angew. Chem., Int. Ed., 2007, 46, 7969 CrossRef.
- F. Zhang and D. Zhao, ACS Nano, 2009, 3, 159 CrossRef CAS.
- C. Liu, H. Wang, X. Li and D. Chen, J. Mater. Chem., 2009, 19, 3546 RSC.
- Y. I. Park, J. H. Kim, K. T. Lee, K.-S. Jeon, H. B. Na, J. H. Yu, H. M. Kim, N. Lee, S. H. Choi, S.-I. Baik, H. Kim, S. P. Park, B.-J. Park, Y. W. Kim, S. H. Lee, S.-Y. Yoon, I. C. Song, W. K. Moon, Y. D. Suh and T. Hyeon, Adv. Mater., 2009, 21, 4467 CrossRef CAS.
- R. Kumar, M. Nyk, T. Y. Ohulchanskyy, C. A. Flask and P. N. Prasd, Adv. Funct. Mater., 2009, 19, 853 CrossRef CAS.
- F. Chen, W. Bu, S. Zhang, X. Liu, J. Liu, H. Xing, Q. Xiao, L. Zhou, W. Peng, L. Wang and J. Shi, Adv. Funct. Mater., 2011, 21, 4285 CrossRef CAS.
- G. Chen, T. Y. Ohulchanskyy, S. Liu, W.-C. Law, F. Wu, M. T. Swihart, H. Ågren and P. N. Prasad, ACS Nano, 2012, 6, 2969 CrossRef CAS.
- Y. Wei, F. Lu, X. Zhang and D. Chen, Chem. Mater., 2006, 18, 5733 CrossRef CAS.
- Q. Liu, Y. Sun, T. Yang, W. Feng, C. Li and F. Li, J. Am. Chem. Soc., 2011, 133, 17122 CrossRef CAS.
- A. Xia, M. Chen, Y. Gao, D. Wu, W. Feng and F. Li, Biomaterials, 2012, 33, 5394 CrossRef CAS.
- T. Yang, Y. Sun, Q. Liu, W. Feng, P. Yang and F. Li, Biomaterials, 2012, 33, 3733 CrossRef CAS.
- J. Zhou, X. Zhu, M. Chen, Y. Sun and F. Li, Biomaterials, 2012, 33, 6201 CrossRef CAS.
- H. Schäfe, P. Ptacek, O. Zerzouf and M. Haase, Adv. Funct. Mater., 2008, 18, 2913 CrossRef.
- O. Ehlert, R. Thomann, M. Darbandi and T. Nann, ACS Nano, 2007, 2, 120 CrossRef.
- Y.-W. Zhang, X. Sun, R. Si, L.-P. You and C.-H. Yan, J. Am. Chem. Soc., 2005, 127, 3260 CrossRef CAS.
- N. Dong, M. Pedroni, F. Piccinelli, G. Conti, A. Sbarbati, J. R. Hernandez, L. M. Maestro, M. C. I. d. l. Cruz, F. Sanz-Rodriguez, A. Juarranz, F. Chen, F. Vetrone, J. A. Capobianco, J. A. G. Sole, M. Bettinelli, D. Jaque and A. Speghini, ACS Nano, 2011, 5, 8665 CrossRef CAS.
- J. Wang, F. Wang, C. Wang, Z. Liu and X. Liu, Angew. Chem., Int. Ed., 2011, 50, 10369 CrossRef CAS.
- A. C. Yanes, A. Santana-Alonso, J. Méndez-Ramos, J. del-Castillo and V. D. Rodríguez, Adv. Funct. Mater., 2011, 21, 3136 CrossRef CAS.
- H. Mai, Y. Zhang, L. Sun and C. Yan, J. Phys. Chem. C, 2007, 111, 13721 CAS.
- H.-X. Mai, Y.-W. Zhang, L.-D. Sun and C.-H. Yan, J. Phys. Chem. C, 2007, 111, 13730 CAS.
- X. Wang, J. Zhuang, Q. Peng and Y. Li, Nature, 2005, 437, 121 CrossRef CAS.
- L. Wang, P. Li, J. Zhuang, F. Bai, J. Feng, X. Yan and Y. Li, Angew. Chem., Int. Ed., 2008, 47, 1054 CrossRef CAS.
- M. Wang, J.-L. Liu, Y.-X. Zhang, W. Hou, X.-L. Wu and S.-K. Xu, Mater. Lett., 2009, 63, 325 CrossRef CAS.
- M. Wang, C.-C. Mi, J.-L. Liu, X.-L. Wu, Y.-X. Zhang, W. Hou, F. Li and S.-K. Xu, J. Alloys Compd., 2009, 485, L24 CrossRef CAS.
- Y. Sun, Y. Chen, L. Tian, Y. Yu, X. Kong, J. Zhao and H. Zhang, Nanotechnology, 2007, 18, 275609 CrossRef.
- G. Tian, Z. Gu, L. Zhou, W. Yin, X. Liu, L. Yan, S. Jin, W. Ren, G. Xing, S. Li and Y. Zhao, Adv. Mater., 2012, 24, 1226 CrossRef CAS.
- G. Yi, H. Lu, S. Zhao, Y. Ge, W. Yang, D. Chen and L.-H. Guo, Nano Lett., 2004, 4, 2191 CrossRef CAS.
- A. Patra, C. S. Friend, R. Kapoor and P. N. Prasad, J. Phys. Chem. B, 2002, 106, 1909 CrossRef CAS.
- A. Patra, C. S. Friend, R. Kapoor and P. N. Prasad, Chem. Mater., 2003, 15, 3650 CrossRef CAS.
- F. Vetrone, J.-C. Boyer, J. A. Capobianco, A. Speghini and M. Bettinelli, J. Appl. Phys., 2004, 96, 661 CrossRef CAS.
- X.-X. Luo and W.-H. Cao, J. Alloys Compd., 2008, 460, 529 CrossRef CAS.
- L. Xu, Y. Yu, X. Li, G. Somesfalean, Y. Zhang, H. Gao and Z. Zhang, Opt. Mater., 2008, 30, 1284 CrossRef CAS.
- X. Qin, T. Yokomori and Y. Ju, Appl. Phys. Lett., 2007, 90, 073104 CrossRef.
- L. Xiong, T. Yang, Y. Yang, C. Xu and F. Li, Biomaterials, 2010, 31, 7078 CrossRef CAS.
- R. Naccache, F. Vetrone, V. Mahalingam, L. A. Cuccia and J. A. Capobianco, Chem. Mater., 2009, 21, 717 CrossRef CAS.
- G. Chen, T. Y. Ohulchanskyy, W. C. Law, H. Ågren and P. N. Prasad, Nanoscale, 2011, 3, 2003 RSC.
- S. J. Budijono, J. Shan, N. Yao, Y. Miura, T. Hoye, R. H. Austin, Y. Ju and R. K. Prud'homme, Chem. Mater., 2010, 22, 311 CrossRef CAS.
- S. A. Hilderbrand, F. Shao, C. Salthouse, U. Mahmood and R. Weissleder, Chem. Commun., 2009, 4188 RSC.
- G.-S. Yi and G.-M. Chow, Chem. Mater., 2007, 19, 341 CrossRef CAS.
- T. Rantanen, M.-L. Järvenpää, J. Vuojola, K. Kuningas and T. Soukka, Angew. Chem., Int. Ed., 2008, 47, 3811 CrossRef CAS.
- L. Cheng, K. Yang, S. Zhang, M. Shao and S. Lee, Nano Res., 2010, 3, 722 CrossRef CAS.
- Q. Zhang, K. Song, J. Zhao, X. Kong, Y. Sun, X. Liu, Y. Zhang, Q. Zeng and H. Zhang, J. Colloid Interface Sci., 2009, 336, 171 CrossRef CAS.
- F. Meiser, C. Cortez and F. Caruso, Angew. Chem., Int. Ed., 2004, 43, 5954 CrossRef CAS.
- B. Dong, S. Xu, J. Sun, S. Bi, D. Li, X. Bai, Y. Wang, L. Wang and H. Song, J. Mater. Chem., 2011, 21, 6193 RSC.
- Z.-L. Wang, J. Hao, H. L. W. Chan, G.-L. Law, W.-T. Wong, K.-L. Wong, M. B. Murphy, T. Su, Z. H. Zhang and S. Q. Zeng, Nanoscale, 2011, 3, 2175 RSC.
- Q. Chen, X. Wang, F. Chen, Q. Zhang, B. Dong, H. Yang, G. Liu and Y. Zhu, J. Mater. Chem., 2011, 21, 7661 RSC.
- T. Cao, T. Yang, Y. Gao, Y. Yang, H. Hu and F. Li, Inorg. Chem. Commun., 2010, 13, 392 CrossRef CAS.
- J. Shen, L.-D. Sun, Y.-W. Zhang and C.-H. Yan, Chem. Commun., 2010, 46, 5731 RSC.
- Z. Chen, H. Chen, H. Hu, M. Yu, F. Li, Q. Zhang, Z. Zhou, T. Yi and C. Huang, J. Am. Chem. Soc., 2008, 130, 3023 CrossRef CAS.
- H. Hu, M. Yu, F. Li, Z. Chen, X. Gao, L. Xiong and C. Huang, Chem. Mater., 2008, 20, 7003 CrossRef CAS.
- H.-P. Zhou, C.-H. Xu, W. Sun and C.-H. Yan, Adv. Funct. Mater., 2009, 19, 3892 CrossRef CAS.
- L. Cheng, K. Yang, Q. Chen and Z. Liu, ACS Nano, 2012, 6, 5605 CrossRef CAS.
- Q. Liu, C. Li, T. Yang, T. Yi and F. Li, Chem. Commun., 2010, 46, 5551 RSC.
- Q. Liu, M. Chen, Y. Sun, G. Chen, T. Yang, Y. Gao, X. Zhang and F. Li, Biomaterials, 2011, 32, 8243 CrossRef CAS.
- L.-L. Li, R. Zhang, L. Yin, K. Zheng, W. Qin, P. R. Selvin and Y. Lu, Angew. Chem., Int. Ed., 2012, 51, 6121 CrossRef CAS.
- H. Hu, L. Xiong, J. Zhou, F. Li, T. Cao and C. Huang, Chem.–Eur. J., 2009, 15, 3577 CrossRef CAS.
- Z. Li, Y. Zhang and S. Jiang, Adv. Mater., 2008, 20, 4765 CrossRef CAS.
- D. K. Chatterjee and Y. Zhang, Nanomedicine, 2008, 3, 73 CrossRef CAS.
- C. Yan, A. Dadvand, F. Rosei and D. F. Perepichka, J. Am. Chem. Soc., 2010, 132, 8868 CrossRef CAS.
- S. Jeong, N. Won, J. Lee, J. Bang, J. Yoo, S. G. Kim, J. A. Chang, J. Kime and S. Kim, Chem. Commun., 2011, 47, 8022 RSC.
- H. Zhang, Y. Li, I. A. Ivanov, Y. Qu, Y. Huang and X. Duan, Angew. Chem., Int. Ed., 2010, 49, 2865 CrossRef CAS.
- F. Zhang, G. B. Braun, Y. Shi, Y. Zhang, X. Sun, N. O. Reich, D. Zhao and G. Stucky, J. Am. Chem. Soc., 2010, 132, 2850 CrossRef CAS.
- O. Veiseha, J. W. Gunna and M. Zhang, Adv. Drug Delivery Rev., 2010, 62, 284 CrossRef.
- G. Mikhaylov, U. Mikac, A. A. Magaeva, V. I. Itin, E. P. Naiden, I. Psakhye, L. Babes, T. Reinhecke, C. Peters, R. Zeiser, M. Bogyo, V. Turk, S. G. Psakhye, B. Turk and O. Vasiljeva, Nat. Nanotechnol., 2011, 6, 594 CrossRef CAS.
- C. Mi, J. Zhang, H. Gao, X. Wu, M. Wang, Y. Wu, Y. Di, Z. Xu and S. Xu, Nanoscale, 2010, 2, 1141 RSC.
- A. Xia, Y. Gao, J. Zhou, C. Li, T. Yang, D. Wu, L. Wu and F. Li, Biomaterials, 2011, 32, 7200 CrossRef CAS.
- X. Yu, Y. Shan, G. Li and K. Chen, J. Mater. Chem., 2011, 21, 8104 RSC.
- F. Chen, S. Zhang, W. Bu, X. Liu, Y. Chen, Q. He, M. Zhu, L. Zhang, L. Zhou, P. W. Peng and J. Shi, Chem.–Eur. J., 2010, 16, 11254 CrossRef CAS.
- L. Cheng, K. Yang, Y. Li, X. Zeng, M. Shao, S.-T. Lee and Z. Liu, Biomaterials, 2012, 33, 2215 CrossRef CAS.
- H. J. M. A. A. Zijlmans, J. Bonnet, J. Burton, K. Kardos, T. Vail, R. S. Niedbala and H. J. Tanke, Anal. Biochem., 1999, 267, 30 CrossRef CAS.
- M. Yu, F. Li, Z. Chen, H. Hu, C. Zhan, H. Yang and C. Huang, Anal. Chem., 2009, 81, 930 CrossRef CAS.
- J.-C. Boyer, M.-P. Manseau, J. I. Murray and C. J. M. v. Veggel, Langmuir, 2010, 26, 1157 CrossRef CAS.
- R. A. Jalil and Y. Zhang, Biomaterials, 2008, 29, 4122 CrossRef.
- S. F. Lim, R. Riehn, W. S. Ryu, N. Khanarian, C.-K. Tung, D. Tank and R. H. Austin, Nano Lett., 2006, 6, 169 CrossRef CAS.
- H. Kobayashi, N. Kosaka, M. Ogawa, N. Y. Morgan, P. D. Smith, C. B. Murray, X. Ye, J. Collins, G. A. Kumar, H. Bell and P. L. Choyke, J. Mater. Chem., 2009, 19, 6481 RSC.
- W. Niu, S. Wu and S. Zhang, J. Mater. Chem., 2010, 20, 9113 RSC.
- X. Yu, M. Li, M. Xie, L. Chen, Y. Li and Q. Wang, Nano Res., 2010, 3, 51 CrossRef CAS.
- L. Cheng, K. Yang, M. Shao, S.-T. Lee and Z. Liu, J. Phys. Chem. C, 2011, 115, 2686 CAS.
- M. Wang, C.-C. Mi, W.-X. Wang, C.-H. Liu, Y.-F. Wu, Z.-R. Xu, C.-B. Mao and S.-K. Xu, ACS Nano, 2009, 3, 1580 CrossRef CAS.
- L.-Q. Xiong, Z.-G. Chen, M.-X. Yu, F.-Y. Li, C. Liu and C.-H. Huang, Biomaterials, 2009, 30, 5592 CrossRef CAS.
- X.-F. Yu, Z. Sun, M. Li, Y. Xiang, Q.-Q. Wang, F. Tang, Y. Wu, Z. Cao and W. Lia, Biomaterials, 2010, 31, 8724 CrossRef CAS.
- X.-F. Yu, Z. Sun, M. Li, Y. Xiang, Q.-Q. Wang, F. Tang, Y. Wu, Z. Cao and W. Li, Biomaterials, 2010, 31, 8724 CrossRef CAS.
- S. T. Selvan, P. K. Patra, C. Yen Ang and J. Y. Ying, Angew. Chem., Int. Ed., 2007, 46, 2448 CrossRef CAS.
- J. Kim, J. E. Lee, S. H. Lee, J. H. Yu, J. H. Lee, T. G. Park and T. Hyeon, Adv. Mater., 2008, 20, 478 CrossRef CAS.
- Y. Liu, K. Ai, J. Liu, Q. Yuan, Y. He and L. Lu, Angew. Chem., Int. Ed., 2012, 51, 1437 CrossRef CAS.
- H. Xu, L. Cheng, C. Wang, X. Ma, Y. Li and Z. Liu, Biomaterials, 2011, 32, 9364 CrossRef CAS.
- J. Zhou, Y. Sun, X. Du, L. Xiong, H. Hua and F. Li, Biomaterials, 2010, 31, 3287 CrossRef CAS.
- Y. Sun, M. Yu, S. Liang, Y. Zhang, C. Li, T. Mou, W. Yang, X. Zhang, B. Li, C. Huang and F. Li, Biomaterials, 2011, 32, 2999 CrossRef CAS.
- X. Zhu, J. Zhou, M. Chen, M. Shi, W. Feng and F. Li, Biomaterials, 2012, 33, 4618 CrossRef CAS.
- D. Hu, M. Chen, Y. Gao, F. Li and L. Wu, J. Mater. Chem., 2011, 21, 11276 RSC.
- N. M. Idris, Z. Li, L. Ye, E. K. W. Sim, R. Mahendran, P. C.-L. Ho and Y. Zhang, Biomaterials, 2009, 30, 5104 CrossRef CAS.
- H. Wang, F. Cao, A. De, Y. Cao, C. Contag, S. S. Gambhir, J. C. Wu and X. Chen, Stem Cells, 2009, 27, 1548 CrossRef CAS.
- D. J. Prockop, C. A. Gregory and J. L. Spees, Proc. Natl. Acad. Sci. U. S. A., 2003, 100, 11917 CrossRef CAS.
- G. Chamberlain, J. Fox, B. Ashton and J. Middleton, Stem Cells, 2007, 25, 2739 CrossRef CAS.
- T. Kim, E. Momin, J. Choi, K. Yuan, H. Zaidi, J. Kim, M. Park, N. Lee, M. T. McMahon, A. Quinones-Hinojosa, J. W. M. Bulte, T. Hyeon and A. A. Gila, J. Am. Chem. Soc., 2011, 133, 2955 CrossRef CAS.
- C. Wang, L. Cheng, H. Xu and Z. Liu, Biomaterials, 2012, 33, 4872 CrossRef CAS.
- L. Cheng, C. Wang, X. Ma, Q. Wang, Y. Cheng, H. Wang, Y. Li and Z. Liu, Adv. Funct. Mater., 2012 DOI:10.1002/adfm.201201733.
- J. P. Celli, B. Q. Spring, I. Rizvi, C. L. Evans, K. S. Samkoe, S. Verma, B. W. Pogue and T. Hasan, Chem. Rev., 2010, 110, 2795 CrossRef CAS.
- J. Kim, Y. Piao and T. Hyeon, Chem. Soc. Rev., 2009, 38, 372 RSC.
- P. Yang, S. Gai and J. Lin, Chem. Soc. Rev., 2012, 41, 3679 RSC.
- M. Colombo, S. Carregal-Romero, M. F. Casula, L. Gutiérrez, M. P. Morales, I. B. Böhm, J. T. Heverhagen, D. Prosperi and W. J. Parak, Chem. Soc. Rev., 2012, 41, 4306 RSC.
- M. Elsabahy and K. L. Wooley, Chem. Soc. Rev., 2012, 41, 2545 RSC.
- G. Tian, Z. Gu, L. Zhou, W. Yin, X. Liu, L. Yan, S. Jin, W. Ren, G. Xing, S. Li and Y. Zhao, Adv. Mater., 2012, 24, 1226 CrossRef CAS.
- Y. Dai, P. a. Ma, Z. Cheng, X. Kang, X. Zhang, Z. Hou, C. Li, D. Yang, X. Zhai and J. Lin, ACS Nano, 2012, 6, 3327 CrossRef CAS.
- Z. Hou, C. Li, P. Ma, Z. Cheng, X. Li, X. Zhang, Y. Dai, D. Yang, H. Lian and J. Lin, Adv. Funct. Mater., 2012, 22, 2713 CrossRef CAS.
- Z. Xu, C. Li, P. a. Ma, Z. Hou, D. Yang, X. Kang and J. Lin, Nanoscale, 2011, 3, 661 RSC.
- H. Herweijer and J. A. Wolff, Gene Ther., 2003, 10, 453 CrossRef CAS.
- C. Zhu, L. Liu, Q. Yang, F. Lv and S. Wang, Chem. Rev., 2012, 112, 4687 CrossRef CAS.
- S. Jiang, Y. Zhang, K. M. Lim, E. K. W. Sim and L. Ye, Nanotechnology, 2009, 20, 155101 CrossRef.
- S. Jiang and Y. Zhang, Langmuir, 2010, 26, 6689 CrossRef CAS.
- M. Kumar, G. Jayakumar, N. M. Idris and Y. Zhang, Proc. Natl. Acad. Sci. U. S. A., 2012, 109, 8483 CrossRef.
- B. Ungun, R. K. Prud'homme, S. J. Budijon, J. Shan, S. F. Lim, Y. Ju and R. Austin, Opt. Express, 2009, 17, 80 CrossRef CAS.
- X.-F. Qiao, J.-C. Zhou, J.-W. Xiao, Y.-F. Wang, L.-D. Sun and C.-H. Yan, Nanoscale, 2012, 4, 4611 RSC.
- P. Zhang, W. Steelant, M. Kumar and M. Scholfield, J. Am. Chem. Soc., 2007, 129, 4526 CrossRef CAS.
- H. Guo, H. Qian, N. M. Idris and Y. Zhang, Nanomed.: Nanotechnol., Biol. Med., 2010, 6, 486 CrossRef CAS.
- K. Liu, X. Liu, Q. Zeng, Y. Zhang, L. Tu, T. Liu, X. Kong, Y. Wang, F. Cao, S. A. G. Lambrechts, M. C. G. Aalders and H. Zhang, ACS Nano, 2012, 6, 4054 CrossRef CAS.
- S. Cui, H. Chen, H. Zhu, J. Tian, X. Chi, Z. Qian, S. Achilefuc and Y. Gu, J. Mater. Chem., 2012, 22, 4861 RSC.
- Y. I. Park, H. M. Kim, J. H. Kim, K. C. Moon, B. Yoo, K. T. Lee, N. Lee, Y. Choi, W. Park, D. Ling, K. Na, W. K. Moon, S. H. Choi, H. S. Park, S.-Y. Yoon, Y. D. Suh, S. H. Lee and T. Hyeon, Adv. Mater., 2012, 24, 5755–5761 CrossRef CAS.
- N. M. Idris, M. K. Gnanasammandhan, J. Zhang, P. C. Ho, R. Mahendran and Y. Zhang, Nat. Med., 2012, 18, 1580–1585 CrossRef CAS.
- P. K. Jain, X. Huang, I. H. El-Sayed and M. A. El-Sayed, Acc. Chem. Res., 2008, 41, 1578 CrossRef CAS.
- S. Lal, S. E. Clare and N. J. Halas, Acc. Chem. Res., 2008, 41, 1842 CrossRef CAS.
- Z. Liu, S. Tabakman, K. Welsher and H. Dai, Nano Res., 2009, 2, 85 CrossRef CAS.
- G. V. Maltzahn, J.-H. Park, A. Agrawal, N. K. Bandaru, S. K. Das, M. J. Sailor and S. N. Bhatia, Cancer Res., 2009, 69, 3892 CrossRef.
- H. Ke, J. Wang, Z. Dai, Y. Jin, E. Qu, Z. Xing, C. Guo, X. Yue and J. Liu, Angew. Chem., Int. Ed., 2011, 50, 3017 CrossRef CAS.
- H. Liu, T. Liu, X. Wu, L. Li, L. Tan, D. Chen and F. Tang, Adv. Mater., 2012, 24, 755 CrossRef CAS.
- J. T. Robinson, S. M. Tabakman, Y. Liang, H. Wang, H. S. Casalongue, D. Vinh and H. Dai, J. Am. Chem. Soc., 2011, 133, 6825 CrossRef CAS.
- K. Yang, S. Zhang, G. Zhang, X. Sun, S.-T. Lee and Z. Liu, Nano Lett., 2010, 10, 3318 CrossRef CAS.
- X. Huang, S. Tang, X. Mu, Y. Dai, G. Chen, Z. Zhou, F. Ruan, Z. Yang and N. Zheng, Nat. Nanotechnol., 2011, 6, 28 CrossRef CAS.
- K. Yang, H. Xu, L. Cheng, C. Sun, J. Wang and Z. Liu, Adv. Mater., 2012, 24, 5586–5592 CrossRef CAS.
- J.-C. Boyer and F. C. J. M. V. Veggel, Nanoscale, 2010, 2, 1417 RSC.
- F. Wang, R. Deng, J. Wang, Q. Wang, Y. Han, H. Zhu, X. Chen and X. Liu, Nat. Mater., 2011, 10, 968 CrossRef CAS.
- L. Cheng, K. Yang, X. Lu, M. Shao and Z. Liu, Nanomedicine, 2011, 6, 1327 CrossRef CAS.
- F. Zhang, R. Che, X. Li, C. Yao, J. Yang, D. Shen, P. Hu, W. Li and D. Zhao, Nano Lett., 2012, 12, 2852 CrossRef CAS.
|
This journal is © The Royal Society of Chemistry 2013 |
Click here to see how this site uses Cookies. View our privacy policy here.