DOI:
10.1039/C2FO30103B
(Paper)
Food Funct., 2012,
3, 1280-1285
Preventive effects of conjugated linoleic acid on obesity by improved physical activity in nescient basic helix-loop-helix 2 knockout mice during growth period
Received
11th May 2012
, Accepted 11th August 2012
First published on 14th August 2012
Abstract
The purpose of this study was to evaluate whether conjugated linoleic acid (CLA) exposure during the developmental period increases voluntary activity, which would influence obesity outcome later in life. The effects of dietary supplementation of 0.5% CLA in a high fat diet were evaluated in nescient basic helix-loop-helix 2 (Nhlh2) knock-out (N2KO) mice, which is a unique animal model representing inactivity-induced obesity in a pre-obese condition. Male wild type and N2KO mice were fed either control or CLA (0.5%) diet for 8 weeks. As expected, control diet fed N2KO animals showed greater body weight with decreased physical activity in the late stage of the experimental period compared with wild type control. Dietary CLA significantly decreased body weight and adipose depots in both wild type and N2KO mice, and the body weights of both genotypes fed CLA were similar during the experimental period. CLA exposure during the developmental period significantly improved the impairment of physical activity in N2KO mice, but the wild type did not show any effect of CLA. In both genotypes, CLA significantly reduced serum triglycerides levels and down-regulated the mRNA expressions of CCAAT/enhancer binding protein α (C/EBPα) and leptin in white adipose tissue. These findings suggest that early CLA exposure could prevent obesity with improved voluntary physical activity in N2KO mice.
Introduction
Conjugated linoleic acid (CLA) was first reported to be an anticancer component from ground beef extract in 1985.1,2 Since then, CLA, as a mixture of multiple geometric and positional isomers, is reported to have a number of other beneficial effects, although its anti-obesity effect has drawn significant attention since the 1990s.1 Multiple mechanisms have been suggested for CLA's effect on body fat reduction; increasing energy expenditure, reducing adipocyte number and size, increasing fatty acid β-oxidation in skeletal muscle, and modulating lipid metabolism by decreasing lipogenesis and increasing lipolysis.1,3 Although it has been known that the trans-10,cis-12 CLA is responsible for CLA's effects on body fat reduction, the overall effects of CLA may result from multiple CLA isomers.1,4
Recently, it was reported that CLA and its 19-carbon cognate (conjugated nonadecadienoic acid) enhanced energy expenditure in part by improving voluntary physical activity in normal mice.5 Additionally, we have previously reported that dietary CLA significantly reduced body fat in older female nescient basic helix-loop-helix 2 (Nhlh2) knock-out (N2KO) mice.6 This is a unique animal model, resulting in age-associated reduced voluntary physical activity preceding the onset of obesity.7 Thus, this N2KO mouse model is a very useful model to investigate the physiological metabolism of obesity development, which is similar to that of humans. This study was designed to investigate CLA's efficacy on voluntary physical activity in young N2KO mice, which may lead to prevention of weight gain. The present study provides an important scientific foundation for future clinical trials of targeted application of CLA for obesity prevention.
Experimental
Materials
CLA was provided from Natural Lipids Ltd. AS (Hovdebygda, Norway). The purity of CLA was 80.7% CLA (37.8% cis-9,trans-11, 37.6% trans-10,cis-12 and 5.3% other isomers), 13.7% oleic acid, 3.2% stearic acid, 0.4% palmitic acid and 0.2% linoleic acid. Semi-purified powdered diets (TD10017 and TD07518) were purchased from Harlan Laboratory (Madison, WI). Serum triacylglyceride (TG), glucose and total cholesterol assay kits were purchased from Genzyme Diagnostics (Charlottetown, PE, Canada). Other solvents used were purchased from either Sigma Chemical Co. (St. Louis, MO) or Fisher Scientific (Pittsburg, PA).
Animals and diet
All animal work was completed in compliance with the Institutional Animal Care and Use Committee at the University of Massachusetts. Animals were housed in a windowless room on a 12-hour light–dark cycle. As described in a previous report,7 heterozygous breeders (129Sv/J) between 2 months and 1 year of age were used for maintenance of the Nhlh2 mouse line. At 3 weeks of age, all animals were weaned and tail biopsy was taken and immediately placed on dry ice for genotype analysis. Since all necessary male wild-type and N2KO mice used in this study were not available at the same time, block design was used; each block consisted of two 3-week-old male wild type and two male N2KO animals. When a block was completed from breeding colonies the mice were moved and housed in individual wire-bottomed cages. During a 1-week adaptation period, all mice were fed a semi-purified powdered diet (TD10017, 5 w/w% fat) and baseline measurement of their voluntary physical activity was obtained. The animals were then randomly divided into two groups and provided either control (high-fat diet) or 0.5% CLA-containing high-fat diet for 8 weeks. Treatment diet consisted of 20 w/w% fat (high-fat diet) to reflect the average American diet with 40% of calories from fat,8 the composition is shown in Table 1. Diet and water were provided ad libitum throughout the experiment and fresh diet was provided twice a week. Body weight and food intake were recorded weekly. At the end of the study, mice were fasted for 4 h and sacrificed by CO2 asphyxiation. Blood was collected by cardiac puncture, and serum was used for further analyses. Internal organs (liver, heart, kidney, spleen and white adipose tissues (WAT)) were weighed.
Table 1 Composition of experimental diets
Ingredient |
g kg−1 |
Casein, vitamin-free tested |
169.1 |
L-cystine |
2.2 |
Sucrose |
100 |
Cornstarch |
288.5 |
Maltodextrin |
132 |
Cellulose |
50 |
Soybean oil |
195 |
CLA or soybean oil |
5 |
Mineral mix, AIN-93M-MX (TD94049) |
42.8 |
Vitamin mix, AIN-93-VX (TD94047) |
12.4 |
Choline bitartrate |
3 |
tert-Butylhydroquinone |
0.04 |
Total |
1000 |
Voluntary physical activity (non-exercise movement)
Voluntary movement was monitored using LoliTrack Quatro Video Tracking Software Version 1.0 (Loligo Systems, Tjele, Denmark) with an infrared camera. The mice were placed into special clear cages (30 × 46 × 40 cm) individually during the dark phase (6:00 pm–5:00 am) once biweekly with free access to diet and water (provided as HydroGel®, Clear H2O, Portland, ME). This cage was larger than a typical mouse cage and was used to prevent a restriction in the numbers and positioning of activity monitors that could be caused by using a small size cage.9 The mice to be tracked were placed against a contrasting background and the software assigned an X, Y coordinate pair to the center of the contrasting objects. The program found all pixels within the range of 640 × 480 pixels and calculated the center X and Y coordinates of these pixels. The time-stamped X, Y-coordinates were written into a data file to 5 times per second, and then the data was imported into Excel for analysis. Movement data for 9 h (8:00 pm–5:00 am) except 2 h (6:00 pm–8:00 pm) of the early phase for adapting to surroundings at dark cycle was analyzed. Voluntary movements were expressed as total travel distance (m) during the dark cycle.
Serum parameters
Serum was separated by centrifugation at 3000g for 20 min at 4°C. Serum samples were used for determination of TG, glucose, and total cholesterol using commercial kits as specified by the manufacturer.
mRNA expression
The white adipose tissue and the liver were homogenized in 1 mL of TRIzol reagent and then total RNA was isolated according to the TRIzol protocol. Total RNA was reverse transcribed to cDNA using a High Capacity cDNA Reverse Transcription kit (Applied Biosystems, Foster, CA), as described in the manufacture's protocol. cDNA was used as a template for the relative quantitation for the selected target genes [CCAAT/enhancer binding protein α (C/EBPα, Mm00514283_s1), leptin (Mm00434759_m1), hormone sensitive lipase (HSL, Mm00495359_m1), uncoupling protein 2 (UCP2, Mm00627597_m1), peroxisome proliferator-activated receptor α (PPARα, Mm00440903_m1), fatty acid synthase (FAS, Mm00662291_g1), fatty acid binding protein 4 (FABP4, Mm00445878_m1)] with predesigned TaqMan gene expression assay kits. Each 20 μL reaction contained 100 ng cDNA, 2X TaqMan Gene Expression Mastermix, forward and reverse primers and TaqMan probe. All reactions were carried out in triplicate with the StepOnePlus Real-Time PCR System (Applied Biosystems) using the following conditions: 50°C for 2 min and 95°C for 10 min followed by forty cycles of 95°C for 15 s and 60°C for 1 min. Results were expressed as a relative value after normalization to GAPDH mRNA.
Statistical analyses
Data were analyzed by two-way ANOVA using PROC GLM of the SAS software for Windows release 9.2 (SAS Institute Inc., Cary, NC, USA) on the W32_VSHOME platform. For the body weight, food intake and voluntary physical activity data, repeated measures were performed for the data analysis. To test for differences in adipose depot and organ weights among the experimental groups, analysis of covariance (ANCOVA) with final body mass as a covariate was used. Homogeneity of regression assumptions of the ANCOVA model was tested and met. The Least Squares Means option using a Tukey–Kramer adjustment was used for the multiple comparisons among the experimental groups. Data are shown as the mean ± S.E. P values <0.05 are reported as statistically significant.
Results
Body weights and food intake
When mice were fed control diet, N2KO mice had significantly greater body weights from week 7 (11-weeks-old) compared to wild type, but there was no difference in body weights between CLA-fed wild type and CLA-fed N2KO mice (Fig. 1A). CLA supplementation significantly reduced body weights in both genotypes, and the effect was greater in N2KO mice in the late stage of the experiment.
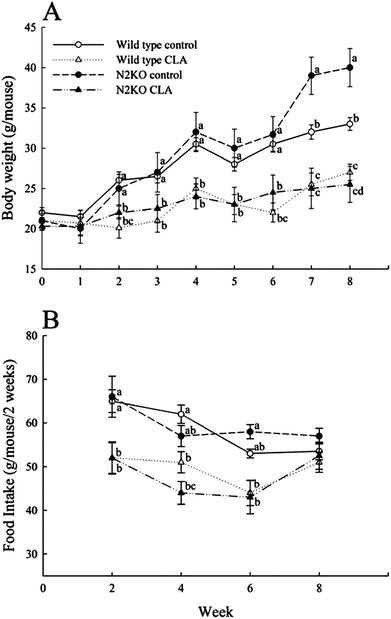 |
| Fig. 1 The effect of CLA on body weight (A) and food intake (B) in wild type and Nhlh2 knock-out mice. Values represent means ± S.E. (n = 3). (a–d) Means with different letters at the same time point are significantly different (P < 0.05). | |
In both dietary treatments, there were no differences in food intake between wild type and N2KO mice during the experimental period (Fig. 1B). CLA fed wild and N2KO animals consumed less food compared to the respective controls, but the food intake did not show differences at the end of the experiment among all treatment groups.
Organ weights
Total adipose tissue weight, including epididymal, mesenteric, and retroperitoneal adipose tissue, liver, heart, kidney, and spleen weights, is shown in Table 2. As expected, N2KO mice had greater adipose depots than wild type (P = 0.0168 for overall genotype effect). Supplementation of CLA reduced adipose depots in both genotypes, although significance was observed only in N2KO mice (P = 0.0420 for overall diet effect). There were no significant differences in the weights of liver, heart, kidney and spleen among all treatment groups.
|
Dietary group |
Effects (P value) |
Wild control |
Wild CLA |
N2KO control |
N2KO CLA |
Diet (D) |
Genotype (G) |
D × G |
Values represent means ± S.E. (n = 3). Means are adjusted for final body mass as a covariate using the ANCOVA analysis. Means with different superscripts within the same row are significantly different (P < 0.05). Adipose depot includes epididymal, mesenteric and retroperitoneal fat.
|
Organ, g
|
Adipose depot |
2.68 ± 0.64b |
1.90 ± 0.81b |
3.91 ± 0.94a |
2.82 ± 0.72b |
0.0420 |
0.0168 |
0.8189 |
Liver |
1.07 ± 0.08 |
1.06 ± 0.09 |
1.07 ± 0.11 |
1.37 ± 0.08 |
0.2999 |
0.0892 |
0.0746 |
Heart |
0.15 ± 0.01 |
0.16 ± 0.02 |
0.11 ± 0.02 |
0.15 ± 0.02 |
0.3096 |
0.2152 |
0.3202 |
Kidney |
0.47 ± 0.07 |
0.48 ± 0.09 |
0.30 ± 0.11 |
0.44 ± 0.08 |
0.5552 |
0.2454 |
0.3887 |
Spleen |
0.07 ± 0.01 |
0.09 ± 0.01 |
0.08 ± 0.01 |
0.10 ± 0.01 |
0.2313 |
0.6284 |
0.8357 |
Voluntary physical activity (non-exercise movement)
To evaluate the effects of CLA on voluntary physical activity, travel distance during the dark cycle (9 h) was recorded every other week (Fig. 2A–E). N2KO animals showed significantly reduced physical activity compared to wild-type controls starting at week 4, although no significant difference between the two control genotypes was observed at the end of the experiment (P = 0.1385 and P = 0.0102 for overall genotype effects at week 4 and week 6, respectively). When CLA was supplemented, no difference in activity was observed in wild-type animals, but CLA feeding significantly prevented the reduction of activity in N2KO animals (P = 0.0205 and P = 0.1472 for overall diet effects at week 6 and week 8, respectively).
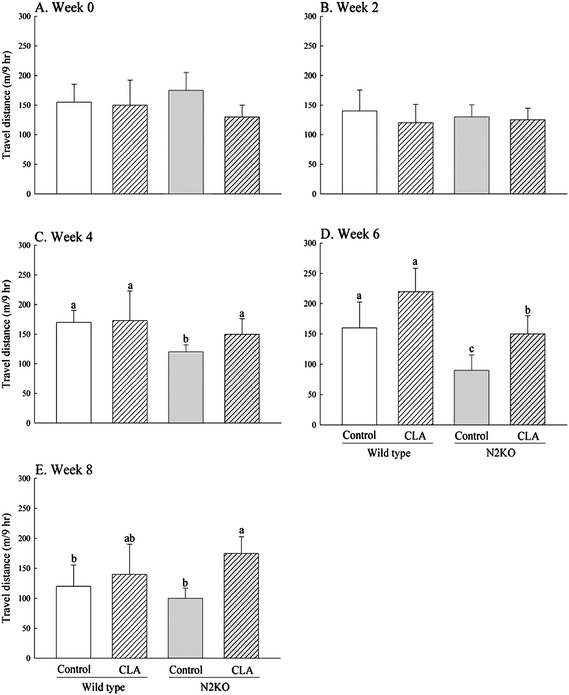 |
| Fig. 2 Effect of CLA on voluntary movement in wild type and Nhlh2 knock-out mice. Voluntary movement was monitored between 8 pm and 5 am once biweekly for 8 weeks during CLA or control feeding (A)–(E). Values represent means ± S.E. (n = 3). (a–c) Means with different letters are significantly different (P < 0.05). | |
Serum parameters
The serum levels of TG, glucose, and total cholesterol in mice were analyzed after CLA treatment for 8 weeks (Table 3). There were no significant differences in serum parameters between the two different genotypes. Supplementing CLA significantly reduced serum TG concentrations in both genotypes (P = 0.0077 for overall diet effect).
Table 3 Serum parametersa
mg dL−1 |
Dietary group |
Effects (P value) |
Wild control |
Wild CLA |
N2KO control |
N2KO CLA |
Diet (D) |
Genotype (G) |
D × G |
Values represent means ± S.E. (n = 3). Means with different superscripts within the same row are significantly different (P < 0.05).
|
Triglyceride |
125.4 ± 38.1a |
60.7 ± 14.9b |
127.4 ± 28.9a |
58.3 ± 12.5b |
0.0077 |
0.9810 |
0.9041 |
Glucose |
285.3 ± 49.2 |
264.2 ± 96.1 |
243.1 ± 74.5 |
315.6 ± 85.1 |
0.7168 |
0.9429 |
0.5096 |
Total cholesterol |
306.1 ± 97.6 |
304.6 ± 35.2 |
327.5 ± 25.4 |
250.2 ± 62.7 |
0.4022 |
0.7225 |
0.4210 |
Effects on lipid metabolism
To determine the gene expression levels of key enzymes involved in lipid and energy expenditure metabolism, the selected mRNA levels were measured in WAT and liver (Fig. 3). No significant differences were observed between wild type and N2KO controls for markers involved in lipid metabolism from WAT, except UCP2 (Fig. 3A). CLA treatment significantly decreased the WAT gene expressions of C/EBPα and leptin in both genotypes (P = 0.0002 and P = 0.0004 for overall diet effects on C/EBPα and leptin, respectively), while CLA had no effect on HSL expression level. The expression of UCP2, the key marker for energy expenditure, was decreased in WAT of CLA fed N2KO mice compared to N2KO control. There were no effects of CLA on mRNA levels of genes involved in lipid metabolism from the liver (Fig. 3B).
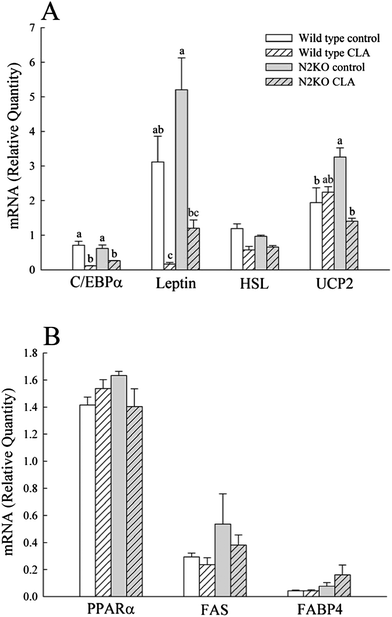 |
| Fig. 3 The effect of CLA on mRNA expressions of selected genes from WAT (A) and liver (B). C/EBPα, CCAAT/enhancer binding protein α; HSL, hormone sensitive lipase; UCP2, uncoupling protein 2; PPARα, peroxisome proliferator-activated receptor α; FAS, fatty acid synthase; FABP4, fatty acid binding protein 4. Values represent means ± S.E. (n = 3). (a–c) Means with different letters are significantly different (P < 0.05). | |
Discussion
In our previous report, dietary trans-10,cis-12 CLA significantly reduced body fat and the serum levels of leptin and TG in older female N2KO mice.6 The work described herein was performed to evaluate the effects of CLA on prevention of inactivity-induced obesity in N2KO animals during growth period. The results from this report indicate that CLA exposure during growth period increased voluntary physical activity, thus effectively preventing inactivity-induced obesity in N2KO animals.
As expected, compared to wild type throughout the experiment period, N2KO mice showed a significant increase in body weight with reduced physical activity, which appeared prior to obesity onset.7 Consistent with the previous publication, CLA supplementation significantly reduced body weights in N2KO mice from the early stage of the experiment (week 2).6 In addition, CLA prevented physical inactivity in N2KO mice starting at week 4. Physical activity is the most variable component of energy expenditure and has been the target of behavioral interventions to control body weight in humans.10 Recently, the systematic review with a number of studies suggested that decreasing any type of sedentary time is associated with lower health risk from obesity and cardiovascular disease in youth aged 5–17 years.11 Therefore, the food-based approach for improving daily physical activity during the growth period could be one strategy for preventing childhood obesity and related metabolic diseases. Previously, CLA has been shown to enhance exercise performance in animals and humans, which is presumed to be due to the increased fat utilization and muscle mass.2,12–15 In addition, the previous study showed that CLA supplementation increased non-exercise physical activity in ICR mice.5 Although it is not currently clear how CLA influences voluntary activity levels, the present findings along with the previous studies suggest that CLA may modulate physical activity in both strenuous exercise and voluntary movement in rodents.
It is possible that CLA influences voluntary physical activity by behavioral regulation via neuro-hormonal system in N2KO mice. Indeed, the reduced physical activity in N2KO animals is due, at least in part, to behavioral or motivational changes,7 and the hypothalamic neurotransmitters are important determinants for the control of motivation, emotion, and reward behaviors.16 Interestingly, CLA has been shown to increase serum concentrations of adrenalin and noradrenalin in mice.17 In addition, leptin, an adipocyte hormone whose expression and secretion is dramatically regulated by CLA, has been reported to influence voluntary activity and energy expenditure in ob/ob and C57BL/6J mice.18,19 Therefore, further investigations are needed to determine how CLA controls voluntary physical activity, in particular with regard to regulation of hormones.
Among two major CLA isomers of current interest, cis-9,trans-11 and trans-10,cis-12, the trans-10,cis-12 CLA has been shown to inhibit lipid accumulation by modulating peroxisome proliferator-activated receptor γ (PPARγ) signaling in adipocytes.20–22 In preadipocytes, the cross-regulation between PPARγ and C/EBPα is a key component of the transcriptional control of adipogenesis and insulin sensitivity.23 Indeed, Rosen et al.24 showed that C/EBPα has no ability to promote adipogenesis in the absence of PPARγ, suggesting a strong correlation between PPARγ and C/EBPα on adipogenic action. In the present study, the mRNA level of C/EBPα in WAT of both genotype mice was significantly decreased by CLA, and this suggests that CLA inhibits fat accumulation by modulating C/EBPα-mediated PPARγ signaling in WAT of growing mice, as shown with reduced adipose depots, leptin expressions, and serum TG levels in CLA fed mice.
It has been suggested that CLA increases energy expenditure, as shown by increased oxygen consumption and expressions of uncoupling proteins by CLA.1,5 In the present study, however, it is not clear whether CLA contributes to an increase of energy expenditure by enhancing physical activity, resulting in body fat reduction. Our previous study showed that N2KO animals exhibit reduced physical activity with no changes in energy expenditure compared to wild type animals, although measured energy expenditure in this study was close to the basal metabolic rate.25 Thus an increased physical activity by CLA would be a significant contributing factor for weight balance in N2KO animals, and warrants further evaluation under simultaneous measurement of energy expenditure from basal metabolic rate and from physical activity to ensure CLA's efficacy.
There are currently seven human clinical studies reporting on CLA and exercise performance.26–32 Four reported that CLA supplementation resulted in positive effects on at least one exercise outcome compared to control.26–29 These studies primarily evaluated effects of CLA on strength or endurance, which are intensive exercises rather than normal movement. This implies that CLA could improve voluntary activity in both exercise and non-exercise forms, although the non-exercise activity has not been evaluated with CLA in humans. However, others reported no changes in exercise outcomes with CLA in humans.30–32
Fatty liver associated with CLA is mainly found in animal studies, and is suggested to be linked to significant fat mobilization from adipocytes and enhanced lipogenesis in the liver.1,33–35 However, O'Hagan and Menzel36 suggested that fatty liver caused by CLA supplementation may be a temporary and reversible response of biological systems to CLA. In fact, patho-physiological evaluation of the liver after 18 months supplementation found no evidence that CLA caused fatty liver.37 Our results here did not suggest any significant differences in liver weights by 8 weeks-supplementation of 0.5% CLA as a mixture of two major isomers in both wild type and N2KO mice. In addition, CLA supplementation did not affect hepatic gene expressions involved in lipogenesis in either genotypes (Fig. 3B).
Conclusions
This is the first report showing that CLA improves voluntary physical activity in an inactivity-induced animal model of obesity. Dietary CLA effectively prevented the impairment of voluntary physical activity in N2KO mice and reduced body fat, serum TG, and the expressions of C/EBPα and leptin from WAT in both wild type and N2KO mice during growth period. These findings suggest that dietary CLA could effectively prevent the development of inactivity-induced obesity during the growth period.
Acknowledgements
Authors thank Ms Jayne M. Storkson for help preparing this manuscript. This material is based up on work supported by the National Institute of Food and Agriculture, U.S. Department of Agriculture, the Massachusetts Agricultural Experimental Station and the Department of Food Science under Project no. MAS00998. Dr Yeonhwa Park is one of the inventors of CLA use patents that are assigned to the Wisconsin Alumni Research Foundation.
Notes and references
- Y. Park and M. W. Pariza, Food Res. Int., 2007, 40, 311–323 CrossRef CAS.
- A. Dilzer and Y. Park, Crit. Rev. Food Sci. Nutr., 2012, 52(6), 488–513 CrossRef CAS.
- A. Kennedy, K. Martinez, S. Schmidt, S. Mandrup, K. LaPoint and M. McIntosh, J. Nutr. Biochem., 2010, 21(3), 171–179 CrossRef CAS.
- M. W. Pariza, Y. Park and M. E. Cook, Prog. Lipid Res., 2001, 40, 283–298 CrossRef CAS.
- Y. Park and Y. Park, Food Chem., 2012, 133, 400–409 CrossRef CAS.
- S. Hur, F. Whitcomb, S. Rhee, Y. Park, D. J. Good and Y. Park, J. Med. Food, 2009, 12(1), 56–63 CrossRef CAS.
- C. A. Coyle, E. Jing, T. Hosmer, J. B. Powers, G. Wade and D. J. Good, Physiol. Behav., 2002, 77, 387–402 CrossRef CAS.
- Y. Park and Y. Park, J. Nutr. Biochem., 2010, 21, 764–773 CrossRef CAS.
- G. A. Wittert, H. Turnbull and P. Hope, Physiol. Behav., 2005, 85, 613–620 CrossRef CAS.
- J. M. Jakicic and A. D. Otto, Am. J. Clin. Nutr., 2005, 82(1), 226S–229S CAS.
- M. S. Tremblay, A. G. LeBlanc, M. E. Kho, T. J. Saunders, R. Larouche, R. C. Colley, G. Goldfield and S. C. Gorber, Int. J. Behav. Nutr. Phys. Activ., 2011, 8, 98 CrossRef.
- W. Mizunoya, S. Haramizu, T. Shibakusa, Y. Okabe and T. Fushiki, Lipids, 2005, 40, 265–271 CrossRef CAS.
- J. H. Kim, H. G. Park, J. H. Pan, S. H. Kim, H. G. Yoon, G. S. Bae, H. Lee, S. H. Eom and Y. G. Kim, J. Med. Food, 2010, 13(5), 1057–1060 CrossRef CAS.
- H. Blankson, J. A. Stakkestad, H. Fagertun, E. Thom, J. Wadstein and O. Gudmundsen, J. Nutr., 2000, 130, 2943–2948 CAS.
- C. Pinkoski, P. D. Chilibeck, D. G. Candow, D. Esliger, J. B. Ewaschuk, M. Facci, J. P. Farthing and G. A. Zello, Med. Sci. Sports Exercise, 2006, 38(2), 339–348 CrossRef CAS.
- U. D. Wankhade, K. R. Vella, D. L. Fox and D. J. Good, PLoS One, 2010, 5(8), e12324 Search PubMed.
- K. Ohnuki, S. Haramizu, K. Oki, K. Ishihara and T. Fushiki, Lipids, 2001, 36, 583–587 CrossRef CAS.
- G. J. Morton, K. J. Kaiyala, J. D. Fisher, K. Ogimoto, M. W. Schwartz and B. E. Wisse, Am. J. Physiol.: Endocrinol. Metab., 2011, 300, E392–E401 CrossRef CAS.
- A. D. Laposky, J. Shelton, J. Bass, C. Dugovic, N. Perrino and F. W. Turek, Am. J. Physiol., 2006, 290, R894–R903 CAS.
- L. Granlund, L. K. Juvet, J. I. Pedersen and H. I. Nebb, J. Lipid Res., 2003, 44(8), 1441–1452 CrossRef CAS.
- L. Granlund, J. I. Pedersen and H. I. Nebb, Biochim. Biophys. Acta, Mol. Cell Biol. Lipids, 2005, 1687(1–3), 11–22 CrossRef CAS.
- J. M. Brown, M. S. Boysen, S. S. Jensen, R. F. Morrison, J. Storkson, R. Lea-Currie, M. W. Pariza, S. Mandrup and M. K. McIntosh, J. Lipid Res., 2003, 44, 1287–1300 CrossRef CAS.
- Z. Wu, E. D. Rosen, R. Brun, S. Hauser, G. Adelmant, A. E. Troy, C. McKeon, G. J. Darlington and B. M. Spiegelman, Mol. Cell, 1999, 3, 151–158 CrossRef CAS.
- E. D. Rosen, C. H. Hsu, X. Wang, S. Sakai, M. W. Freeman, F. J. Gonzalez and B. M. Spiegelman, Genes Dev., 2002, 16, 22–26 CrossRef CAS.
-
J. H. Kim, Y. Park, D. Kim, D. J. Good and Y. Park, J. Nutr. Biochem. DOI:10.1016/j.jnutbio.2012.02.005.
- H. Blankson, J. A. Stakkestad, H. Fagertun, E. Thom, J. Wadstein and O. Gudmundsen, J. Nutr., 2000, 130, 2943–2948 CAS.
- C. Pinkoski, P. D. Chilibeck, D. G. Candow, D. Esliger, J. B. Ewaschuk, M. Facci, J. P. Farthing and G. A. Zello, Med. Sci. Sports Exercise, 2006, 38(2), 339–348 CrossRef CAS.
- M. Tarnopolsky, A. Zimmer, J. Paikin, A. Safdar, A. Aboud, E. Pearce, B. Roy and D. Doherty, PLoS One, 2007, 10, e991 Search PubMed.
- S. M. Cornish, D. G. Candow, N. T. Jantz, P. D. Chilibeck, J. P. Little, S. Forbes, S. Abeysekara and G. A. Zello, Int. J. Sport Nutr. Exercise Metab., 2009, 19(1), 79–96 CAS.
- R. B. Kreider, M. P. Ferreira, M. Greenwood, M. Wilson and A. L. Almada, J. Strength Condit. Res., 2002, 16, 325–334 Search PubMed.
- S. Colakoglu, M. Colakoglu, F. Taneli, F. Cetinoz and M. Turkmen, J. Sports Med. Phys. Fitness, 2006, 46, 570–577 CAS.
- M. L. Diaz, B. A. Watkins, Y. Li, R. A. Anderson and W. W. Campbell, J. Nutr. Biochem., 2008, 19, 61–68 CrossRef CAS.
- M. W. Pariza, Am. J. Clin. Nutr., 2004, 79, 1132S–1136S CAS.
- L. Clement, H. Poirier, I. Niot, V. Bocher, M. Guerre-Millo, S. Krief, B. Staels and P. Besnard, J. Lipid Res., 2002, 43, 1400–1409 CrossRef CAS.
- H. Poirier, I. Niot, L. Clement, M. Guerre-Millo and P. Besnard, Biochimie, 2005, 87, 73–79 CrossRef CAS.
- S. O'Hagan and A. Menzel, Food Chem. Toxicol., 2003, 41, 1749–1760 CrossRef CAS.
- Y. Park, K. J. Albright and M. W. Pariza, Food Chem. Toxicol., 2005, 43(8), 1273–1279 CrossRef CAS.
|
This journal is © The Royal Society of Chemistry 2012 |