Asymmetric reduction of a key intermediate of eslicarbazepine acetate using whole cell biotransformation in a biphasic medium
Received
21st December 2011
, Accepted 9th February 2012
First published on 10th February 2012
Abstract
A whole-cell mediated asymmetric reduction of oxcarbazepine 1 to S-licarbazepine 2, a key intermediate in the synthesis of Eslicarbazepine acetate, was achieved using the yeast strain Pichia methanolica 103660. The reduction was carried out in a biphasic system consisting of (1
:
1) de-ionized water and hexane. This organism catalyzed the reaction to yield the S-enantiomer 2 with excellent conversion (>98%) and enantiomeric excess (ee > 98%) within 24 h of incubation.
1. Introduction
Eslicarbazepine acetate (ELA) is a third-generation drug belonging to the carbamazepine (CBZ) family and is the active analog of oxcarbazepine (OXC).1 CBZ drugs are used in the management of epilepsy, trigeminal neuralgia and brain disorders. Unlike oxcarbazepine (the oxidized form) which is converted to both (R) and (S)-licarbazepine in vivo, ELA (the reduced form) is a single enantiomer pro-drug,1–3 which are gaining prominence over racemates in the pharmaceutical industry.
The synthesis of S-licarbazepine has been tried via chemical and enzymatic routes. The resolution of racemic-10-hydroxy-10,11-dihydro-dibenzo[b,f]azepine-5-carboxylic acid amide has been described and uses optically pure menthoxy acetic acid4 and tartaric acid anhydride.5 However, these resolution methods lead to the loss of 50% of the racemic material. Asymmetric reduction of commercially available oxcarbazepine is a straight forward method for the synthesis of S-licarbazepine. However, the synthetic efficiency depends on stereoselectivity.
Nevertheless, there are many such approaches precedented in the literature e.g. the asymmetric reduction of a ketone followed by esterification6 or asymmetric hydrogenation of the enol-acetate.7 Both approaches use expensive chiral Ru or Rh catalysts that may not be commercially viable choices for production.
Microbial reductions using bacteria or yeast have been known for decades and yeasts such as Saccharomyces cerevisiae were especially applied in biocatalytic processes.8–12 Both wild type microbes13,14 as well as designer recombinant cells15–17 were successfully employed for ketone reduction on a large scale. In comparison to isolated enzymes, the enzymes in whole-cells are usually more stable, and have the added advantage of an internal co-factor regeneration system. The addition of inexpensive glucose is sufficient to generate the co-factor and to drive the reaction forward.18,19 However, low product concentrations resulting from a limited water solubility of many organic compounds is a major drawback. Reaction medium engineering plays an important role in whole-cell based ketone reductions. The use of water miscible organic solvents,20 non-polar solvents in a biphasic system,21 ketone substrate as an immiscible phase22 and neat substrate as a reaction medium23 have been documented.
Whole-cell bioreduction offers an alternative cost-effective route for producing S-licarbazepine from oxcarbazepine and recently, asymmetric reduction of oxcarbazepine was carried out using Saccharomyces cerevisiae CGMC No. 2266.24
To the best of our knowledge there is no report of the microbial reduction of 1 using Pichia methanolica 103660 as a catalyst. In particular, the reaction was carried out in an aqueous organic biphasic system using glucose as a co-substrate. Reaction parameters such as pH, temperature, substrate concentration and glucose addition were investigated to obtain an optimized bioreduction process.
2. Results & discussion
2.1. Screening
The first step in the development of a whole-cell mediated biocatalytic process is the identification of a strain which has ketone reductase (KRED) activity. Initial screening experiments using different wild-type organisms from our internal collection afforded us three potential strains (including P. methanolica) with <5.0% activity and ∼40% enantiomeric excess towards the (S)-enantiomer 2.
Such a low activity may be due to the extremely low solubility of oxcarbazepine in most of the solvents. The screening reaction conditions utilized 0.2 g l−1 of 1 in the production medium itself. These experiments showed that the volumetric productivity and enantioselectivity has to be improved to achieve an economically viable biocatalytic process. Optimization was initiated utilizing Pichia methanolica as a target microorganism for the bioreduction of 1.
2.2. Co-factor regeneration
To further characterize the bioreduction of 1 to 2 using P. methanolica, the reactions were carried out using resting cells in an aqueous system. However, the reaction did not progress beyond a 15% conversion even after 120 h of incubation. This may be due to the lack of co-factor regeneration in the resting cells reaction. There are numerous reports where glucose is used as a co-substrate to increase the conversion rate in ketone reductions.18,19 The presence of glucose dehydrogenase in cells helps in co-factor regeneration (NADP to NADPH) by converting glucose into gluconic acid (Scheme 1). Table 1 shows the different concentrations of glucose utilized in the bioreduction reaction. The conversion increased from 15 to 72% after just 48 h of incubation using 5% glucose while the enantiomeric excess did not improve.
Table 1 Influence of glucose concentration on the bioreduction of ketone 1 to S-alcohol 2 in aqueous phase
|
Glucose concentration (%) |
1.0 |
3.0 |
5.0 |
7.0 |
Conversion (%) |
35.2 |
40.1 |
71.8 |
69.5 |
Enantiomeric excess (%) |
53.8 |
53.5 |
55.7 |
57.4 |
2.3. Improvement of asymmetric reduction using water immiscible organic co-solvent
Ketone 1 is practically insoluble in water and has poor solubility in most other polar solvents except DMSO wherein it is moderately soluble. However, working with DMSO as a reaction solvent has operational complexities, such as extraction of the product. It is known that enzymes can be active in hydrophobic solvents and there are reports that suggest maintenance of the active site of an enzyme is possible by maintaining several layers of hydration in non-polar solvents.25 It is believed that the toxicity of an organic solvent on a whole cell decreases with an increase in the log P value.26–28 But there are also reports to the contrary wherein the non-polar co-solvent decreases the biocatalytic performance.29 No clear correlation between the log P value and biocatalytic activity could be discerned. This calls for case specific optimization of the reaction medium for every substrate. Keeping in view the properties of the substrate and product, we performed the bioreduction in aqueous biphasic systems using non-polar solvents such as hexane, heptanes, cyclohexane etc. To our surprise, the ketone reduction proceeded extremely well with >85% conversion and >98% enantioselectivity in a hexane–water system (Fig. 1). The reason behind the increase in enantioselectivity is either that hexane might have inhibited other, less selective, KREDs or it could have promoted the activity of the selective enzyme. Based on these results, experiments were conducted to verify the ratio of hexane to water required to achieve maximum bioreduction of 1. Eventually, a 1
:
1 ratio of hexane to water afforded the best conversion.
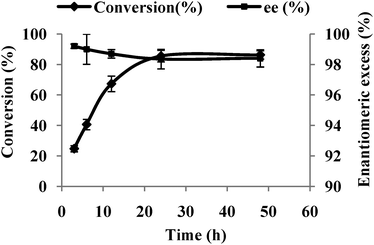 |
| Fig. 1 Bioreduction of ketone 1 by P. methanolica whole-cells in a biphasic system at 30 °C. | |
2.4. Effect of initial substrate concentration
The initial substrate concentration in the reaction medium influences the enzyme activity and may also result in substrate inhibition. Therefore, the influence of the initial substrate concentration on the reduction of ketone 1 to S-alcohol 2 was investigated. The reactions were carried out using different concentrations (0.5–3.0 g l−1) of 1 and keeping the other parameters constant. The conversion is highest when the initial concentration of ketone 1 was 1.5 g l−1 after 24 h of incubation (Fig. 2). However, the decrease in the conversion rate with a further increase in substrate concentration or decreased dilution indicated substrate inhibition. The increase in the substrate concentration did not have any effect on the enantiomeric excess of the product in the presence of hexane as co-solvent, which makes the process feasible for scale-up. Moreover, we were not able to achieve more than an 88% conversion even after 48 h of incubation. However, higher conversions were achieved by using glucose as a co-substrate in the biphasic system. In the presence of 5.0% glucose as a co-substrate, it was possible to increase the reaction conversion to >95% (85% isolated yield) and with >98% ee.
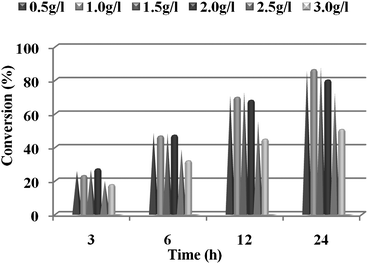 |
| Fig. 2 Effect of substrate concentration on the synthesis of S-alcohol 2 by using whole-cells of P. methanolica (The reactions were carried out for 24 h at 30 °C and 150 rpm using 50% (v/v) hexane). | |
2.6. Effect of physical parameters
The effect of physical parameters such as temperature and agitation were investigated. The reaction was carried out under variable temperatures (20–40 °C) and agitation (100–200 rpm). The optimum temperature and agitation rate for the bioreduction of 1 to 2 using resting cells of P. methanolica were 30 °C and 150 rpm, respectively.
In order to scale-up the bioreduction process, determination of the optimum resting cells concentration in the reaction medium is critical. A higher conversion rate can be achieved by loading more biomass into the reaction mixture. The maximum conversion of >98% and enantioselectivity >98% was achieved by using 150 g l−1 (wet weight) of cells (Fig. 3).
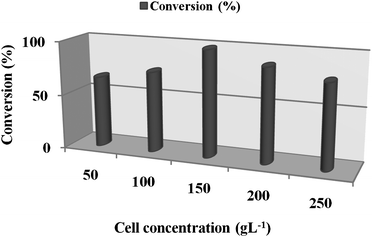 |
| Fig. 3 Influence of cell concentration of P. methanolica on the bioreduction of 1 to 2 after 24 h (The bioreduction consisted of the substrate (1.5 g l−1), glucose (5%), and hexane 50% (v/v)). | |
The reaction was carried out at a preparative scale using 5.0 g of ketone 1. The product was isolated in >85% chemical yield and with >98% optical purity using ethyl acetate as the extracting solvent. This suggests that the KRED present in P. methanolica is a versatile biocatalyst, able to reduce 1 with excellent conversion and enantioselectivity.
3. Conclusion
An aqueous–organic biphasic system was developed and applied to the whole-cell asymmetric ketone reduction. This process affords a key intermediate of the anti-epileptic drug eslicarbazepine acetate. The principal challenge in this study was to increase the conversion and enantioselectivity, which was achieved by developing a whole-cell reduction process in the presence of a water immiscible organic co-solvent. The results are promising for the practical application of whole-cell reduction using P. methanolica for the production of (S)-10-hydroxy-10,11-dihydro-5H-dibenzo[b,f]azepine-5-carboxamide 2.
4. Materials and methods
4.1 Microorganism and chemicals
Pichia methanolica was obtained from Chirotech Technology Centre, Cambridge, UK. Oxcarbazepine was purchased from CTX Life Sciences Private Limited, Gujarat and the S-alcohol was synthesized in-house by a chemical reduction process. The structure of the S-alcohol was confirmed by 1H NMR, FTIR, LC-MS and chiral purity was checked by normal phase chiral HPLC. All other chemicals were of analytical grade and procured from commercial sources. The seed and production medium used was potato dextrose broth (PDB) and was purchased from Himedia Laboratories Pvt. Ltd. Mumbai.
4.2 Medium and cultivation
A solid medium was used for preserving the culture consisting of potato dextrose agar (PDA). The single colony picked from the solid medium was inoculated in 20 ml of the liquid medium (PDB, pH 7.0) in a 100 ml Erlenmeyer flask and incubated in an orbital shaker at 30 °C and 150 rpm for 16 h. Subsequently, 5.0% of the seed culture was transferred into 150 ml of the production medium (PDB, pH 7.0) and incubated. After 48 h of growth, the cells were harvested by centrifugation (5000 rpm, 5 min), and the cell pellet was washed twice with de-ionized water and separated by centrifugation.
4.3. Asymmetric reduction reaction
Accurately weighed 1 (30 mg, 1.5 g l−1) was placed in a 100 ml Erlenmeyer flask containing hexane (10 ml). The cell pellet obtained after centrifugation as above was suspended in a 5.0% glucose solution (10 ml) and added to the reaction mixture. The reaction mixture was incubated in an orbital shaker at 30 °C and 150 rpm for 48 h. 2 ml samples were taken after 3, 6, 12, 24 and 48 h of incubation and analysed for chemical and chiral purity. The sample aliquots were mixed with ethyl acetate (2 ml), vortexed briskly and then centrifuged (10
000 rpm for 2 min) to remove the cells. The organic layer was separated, dried under nitrogen and submitted for HPLC analysis.
4.4. Bioreduction optimization
The effect of various non-polar solvents (hexane, heptane, toluene, octane and cyclohexane) on the bioreduction of ketone 1 to S-alcohol 2 was investigated. Unless otherwise specified, the total volume of the solvent was always 50% of the total volume of the reaction mixture. To evaluate the effect of glucose, various concentrations (1.0–7.0%) were used to suspend the P. methanolica cells. The concentration of glucose was calculated on the basis of the volume of water used in the reaction mixture. In order to investigate the effect of substrate concentration, varying amounts of ketone 1 (0.5–3.0 g l−1) were subjected to bioreduction using P. methanolica cells. Furthermore, to optimize the cell concentration, varying concentrations of cell suspensions (50–250 g l−1) were incubated with 1.5 g l−1 of the substrate at 30 °C (200 rpm) and analyzed for reduction efficiency and enantioselectivity. Finally, the physico-chemical parameters, such as temperature and agitation rate, were optimized. To ascertain the optimum temperature, the reaction was carried out at different temperatures (20–40 °C) in an incubator shaker. The agitation rate was monitored by varying the speed (100–200 rpm) in an orbital shaker.
4.5. Scale-up
In order to scale-up the bioreduction of ketone 1, the reaction was carried out in two 5 L Erlenmeyer flasks and each flask contained resting cells of P. methanolica (150.0 g l−1), H2O–hexane (2 L), glucose (5.0%) and ketone 1 (2.5 g). The reaction was carried out at 30 °C for 48 h in orbital shaker (150 rpm). After complete conversion, the reaction mass was centrifuged (7000 rpm, 5 min) and the hexane was separated. The aqueous layer was passed through a filter and extracted with an equal volume of ethyl acetate. The hexane and ethyl acetate fractions were combined and evaporated under reduced pressure to yield the product.
4.6. Analytical methods
The optical purity of S-alcohol 2 was determined by using a Chiralcel OD-H column (Daicel Chemical Ind., Ltd., Japan). The analysis was performed on an Agilent 1100 Series instrument equipped with a UV-detector. The enantiomers were eluted using hexane (75%), ethanol (25%) and TFA (0.05%) at a flow rate of 0.8 ml min−1. The retention times for the R-isomer, S-isomer and OXC were 9.4, 10.5 and 14.9 min, respectively. The samples were monitored at 215 nm and the area under each peak was integrated to calculate the enantiomeric excess. The chemical purity of the compound was analyzed by Reverse phase HPLC using a Zorbax C-18 column in an Agilent 1100 system. The samples were monitored at 215 nm and every peak having a >0.1 percentage area was considered as an impurity.
Acknowledgements
Author MS is thankful to K Lavanya and Subbaiah Arla for complete AR&D support during the project development.
Notes and references
- L. Almeida and P. Soares-da-Silva, J. Clin. Pharmacol., 2004, 44, 906–918 CrossRef CAS.
- C. Dulsat, N. Mealy, R. Castaner and J. Bolos, Drugs Future, 2009, 34, 189 CrossRef CAS.
- M. Rauchenzauner and G. Luef, Neuropsychiatr. Dis. Treat., 2010, 6, 723–730 CrossRef CAS.
- A. Volosov, B. Yagen and M. Bialer, Epilepsia, 2000, 41, 1107–1111 CrossRef CAS.
-
D. A. Learmonth, WO 02092572, 2002.
-
D. A. Learmonth, G. A. Grasa, A. Zanotti-Gerosa, WO 2007012793 A1, 2007.
-
Y. Bing, L. Wenge and A. L. David, 2010.
- L. C. Bencze, C. Paizs, M. I. ToÅŸa and F. D. Irimie, Tetrahedron: Asymmetry, 2010, 21, 356–364 CrossRef CAS.
- M. Carlquist, C. Olsson, B. Bergdahl, E. W. J. van Niel, M. F. Gorwa-Grauslund and T. r. Frejd, J. Mol. Catal. B: Enzym., 2009, 58, 98–102 CrossRef CAS.
- Q. Hu, Y. Xu and Y. Nie, Bioresour. Technol., 2010, 101, 8502–8508 CrossRef CAS.
- O. W. Gooding, R. Voladri, A. Bautista, T. Hopkins, G. Huisman, S. Jenne, S. Ma, E. C. Mundorff, M. M. Savile, S. J. Truesdell and J. W. Wong, Org. Process Res. Dev., 2009, 14, 119–126 CrossRef.
- J. Liang, J. Lalonde, B. Borup, V. Mitchell, E. Mundorff, N. Trinh, D. A. Kochrekar, R. Nair Cherat and G. G. Pai, Org. Process Res. Dev., 2009, 14, 193–198 CrossRef.
- M. Kataoka, K. Kita, M. Wada, Y. Yasohara, J. Hasegawa and S. Shimizu, Appl. Microbiol. Biotechnol., 2003, 62, 437–445 CrossRef CAS.
- N. Kizaki, Y. Yasohara, J. Hasegawa, M. Wada, M. Kataoka and S. Shimizu, Appl. Microbiol. Biotechnol., 2001, 55, 590–595 CrossRef CAS.
- H. Gröger, F. Chamouleau, N. Orologas, C. Rollmann, K. Drauz, W. Hummel, A. Weckbecker and O. May, Angew. Chem., Int. Ed., 2006, 45, 5677–5681 CrossRef.
-
Y. Ni and J.-H. Xu, Biotechnology Advances, 2011 DOI:10.1016/j.biotechadv.2011.10.007.
- K. Schroer, K. Peter Luef, F. Stefan Hartner, A. Glieder and B. Pscheidt, Metab. Eng., 12, 8–17 CrossRef CAS.
-
K. Faber, Biotransformations in organic chemistry, Springer, Berlin, 2004 Search PubMed.
-
K. Nakamura and T. Matsuda, Reduction of ketones, Wiley-VCH Verlag GmbH, Weinheim, 2002 Search PubMed.
- J. Schumacher, M. Eckstein and U. Kragl, Biotechnol. J., 2006, 1, 574–581 CrossRef CAS.
- M. Miroliaei and M. Nemat-Gorgani, Int. J. Biochem. Cell Biol., 2002, 34, 169–175 CrossRef CAS.
- C. Kohlmann, N. Robertz, S. Leuchs, L. Greiner and S. Na'amnieh, Green Chem., 2011, 13, 3093–3095 RSC.
- A. Jakoblinnert, R. Mladenov, A. Paul, F. Sibilla, U. Schwaneberg, M. B. Ansorge-Schumacher and P. D. de Maria, Chem. Commun., 2011, 47, 12230–12232 RSC.
- Z.-M. Ou, H.-B. Shi, X.-Y. Sun and W.-H. Shen, J. Mol. Catal. B: Enzym., 2011, 72, 294–297 CrossRef CAS.
- A. M. Klibanov, Nature, 2001, 409, 241–246 CrossRef CAS.
- C. Laane, S. Boeren, K. Vos and C. Veeger, Biotechnol. Bioeng., 1987, 30, 81–87 CrossRef CAS.
- P. Nikolova and O. P. Ward, Journal of Industrial Microbiology & Biotechnology, 1992, 10, 169–177 CAS.
- P. Nikolova and O. P. Ward, Journal of Industrial Microbiology & Biotechnology, 1993, 12, 76–86 CAS.
- R. Kratzer, M. Pukl, S. Egger and B. Nidetzky, Microb. Cell Fact., 2008, 7, 37 CrossRef.
|
This journal is © The Royal Society of Chemistry 2012 |