DOI:
10.1039/C0SC00610F
(Edge Article)
Chem. Sci., 2011,
2, 901-906
Self-referenced RGB colour imaging of intracellular oxygen†
Received
5th December 2010
, Accepted 25th January 2011
First published on 18th February 2011
Abstract
Intracellular
oxygen is an important indicator for cell metabolism and respiration. We have designed self-referenced RGB PEBBLEs that enable a simple readout of the intracellular oxygen distribution with conventional wide-field microscopy and a standard RGB digital camera. The RGB PEBBLEs consist of a hydrophobic matrix covered with amino groups on the surface to confer water-dispersibility. Two luminophores are incorporated in a hydrophobic polystyrene matrix that is highly permeable to oxygen. In polystyrene, the dyes are largely protected from quenching or aggregation by cellular components. The dyes have been selected to match the green and the red channel of digital cameras. While the red emission of the oxygen probe is highly sensitive to oxygen with a quenching response of 74%, the green emission of the reference dye is stable under varying oxygen concentrations. Ratiometric images of intracellular oxygen have been acquired that are inherently resistant to fluctuations in absolute signal intensities. As RGB PEBBLEs respond within seconds to changing oxygen concentrations, they are amenable to monitoring fast cellular dynamics.
Introduction
Molecular oxygen is involved in various physiological and intracellular processes such as photosynthesis, cell respiration, signal transduction,1,2cellular metabolism,3organelle functions,4 and cancer progression.5 Thus, monitoring the distribution of oxygen in living cells provides invaluable information for biology and medicine. Several optical sensors for imaging intracellular oxygen have been established, which for the most part consist of luminescent probes quenched by increasing levels of molecular oxygen.6 The probes' response to oxygen can be interrogated by measuring either luminescence intensity or lifetime. While intensity measurements are prone to fluctuations in the intensity of the light source and the sensitivity of the photodetector, luminescence lifetime3,7 measurements are self-referenced and more robust. Capturing lifetime signals, however, often is too slow for real-time imaging of fast and highly dynamic intracellular activities.
A simple class of intracellularoxygen probes consists of dissolved luminophores, such as pyridyl, ruthenium phenanthroline or platinum porphyrins.8–12Probes in solution, however, can sequester in organelles, which ultimately limits the experiment's duration. Furthermore, the hydrophobicity of many oxygen probes can lead to aggregate formation and binding to cellular proteins. This can strongly alter the probe's response to oxygen. These shortcomings of probes in solution were avoided by immobilising oxygen probes on the surface of lipobeads13 or using “probes encapsulated by biologically localised embedding (PEBBLEs)”14 in silicate15,16 or polymer7,12,17nanoparticles. In addition to the luminescent oxygen probe, a second luminophore that is not quenched by oxygen has usually been embedded as a reference dye to obtain ratiometric data, which are inherently resistant to fluctuations in the intensity of the light source and the sensitivity of the detector.
The last missing step for efficient intracellular oxygen imaging is a reliable and fast readout scheme that is amenable to automation. So far, intracellular oxygen has been measured ratiometrically by recording separate images for each emission wavelength using appropriate filter sets. In the present setup, however, only a single image is acquired for the emission of the oxygen probe as well as the reference dye.18 Almost all digital colour cameras are sensitive to red, green and blue (RGB) light and record the data in three separate channels to cover the whole visible spectrum (Fig. S.1 in the supplementary information†). Each channel holds the colour information as brightness values. A colour image appears only if all three channels are mixed. To acquire the emission of the oxygen probe and the reference dye in separate channels, emission wavelengths must be chosen that are in line with the RGB sensitivity of the camera. Furthermore, the oxygen probe and the reference dye should have similar excitation wavelengths. In this case, the excitation light e.g. from a mercury arc lamp, can be easily separated from the emission light by a dichroic mirror. This simple setup is amenable to wide-field as well as confocal fluorescence microscopy equipped with custom CCD- or CMOS-cameras, and accelerates the image acquisition time.
Results and discussion
Selection of polymer matrix, oxygen probe and reference dye
Accurate and fast RGB imaging of intracellular oxygen depends on an optimised sensor design. We devised a new class of so called RGB PEBBLEs for ratiometric oxygen sensing (Fig. 1A). Amino-modified polystyrene beads of 500-nm diameter confer excellent water-dispersibility and at the same time provide a very stable hydrophobic and oxygen-permeable core for embedding hydrophobic dyes. The highly electron-deficient platinum(II) meso-tetrakis-(pentafluorophenyl)porphyrinato (PtTF20PP) complex (Fig. 1B) is a sensitive probe for oxygen.18,19 Upon excitation in the Soret band (390 nm), the complex emits red light at 650 nm with a narrow bandwidth that matches solely the red channel of the CMOS camera used in these experiments. Other oxygen probes are also conceivable20 as long as they can be excited with blue light and the emission matches the sensitivity of the red channel of the RGB camera. Such oxygen probes include platinum or palladium complexes with octaethylporphine or octaethylporphine ketone ligands (PtOEP, PtOEPK, PdOEP or PdOEPK).21 The fluorophore N-(5-carboxypentyl)-4-piperidino-1,8-naphthalimide butyl ester (Fig. 1C), on the other hand, was chosen as a reference dye. Other 4-amino-1,8-naphtalimide dyes may also be used. The reference dye can be excited with blue light, too, but the emission at 490 nm is recorded on the green channel of the camera. As the reference dye displays a broader emission spectrum than the oxygen probe, there is some overlap with the sensitivity of the red channel. This overlap, however, generates no artifacts as the emission does not change at different oxygen concentrations. Oxygen probe and reference dye are much more photostable than the widely used fluorescein and display nearly identical bleaching rates, such that the ratio remains constant over the whole course of an experiment (Fig. 1D). Polystyrene beads were swollen in an organic solvent under ultrasonication to embed the dyes. Due to their hydrophobic character, leaching of oxygen probe and reference dye from the polystyrene matrix was only detectable in organic solvents, but not in aqueous buffers, i.e under physiologically relevant conditions (Fig. S.2A in the supplementary information†). The response of RGB PEBBLEs may be subject to the presence of intracellular proteins as shown by a protein interference test (Fig. S.2B†). This phenomenon can be attributed to dye molecules on the surface of the RGB PEBBLEs where they are not completely protected by the polystyrene matrix.
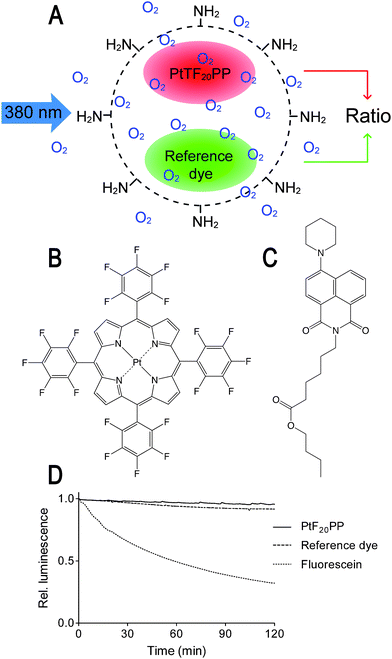 |
| Fig. 1
(A) Schematic drawing of a ratiometric oxygen-sensitive polystyrene RGB PEBBLE. (B) Chemical structure of the oxygen-sensitive platinum(II) meso-tetrakis-(pentafluorophenyl)-porphyrinato (PtTF20PP) complex. (C) Chemical structure of the reference dye N-(5-carboxypentyl)-4-piperidino-1,8-naphthalimide butyl ester. (D) Both dyes are much more photostable than the widely used dye fluorescein and bleaching rates are virtually identical. | |
Characterisation of RGB PEBBLEs
Transmission electron microscopy shows that RGB PEBBLEs are smaller than the original polystyrene beads and have a narrow size distribution (410–430 nm; Fig. 2A). The luminescence spectrum of the RGB PEBBLEs is characterised by two bands with peaks at 490 nm and 650 nm, respectively, corresponding to the emission of the reference and the oxygen probe (Fig. 2B). The emission intensities can be adjusted by the ratio of the oxygen probe and the reference dye embedded in the polystyrene matrix. As the hydrophobic polystyrene prevents access of protons to the dyes, the spectrum is not affected by pH (Fig. 2C), an important aspect especially in an intracellular environment. While the reference dye shows no emission change under different oxygen partial pressures, the emission intensity of the PtTF20PP complex is fivefold lower under oxygen saturation than under nitrogen saturation (Fig. 2D). There is no shift of the emission peak at different oxygen partial pressures. The PtTF20PP complex in the bead responds within seconds to a changing oxygen atmosphere, attesting to the high sensitivity of this oxygen probe as well as the excellent oxygen permeability of the polystyrene bead. In addition, the oxygen response of the PtTF20PP complex is highly reversible and fast (Fig. 2E and Supplementary video†). Changing repeatedly between an oxygen and a nitrogen atmosphere has no influence on the response pattern.
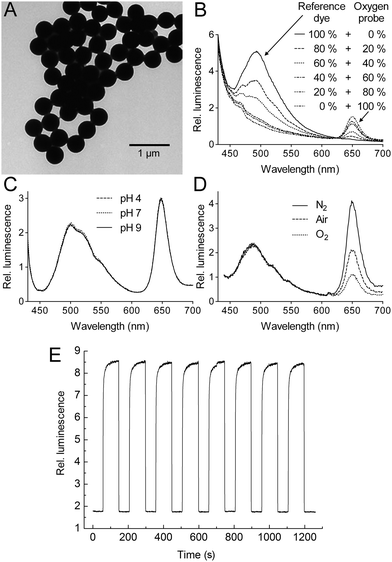 |
| Fig. 2
(A)
Transmission electron microscopy reveals a uniform size of RGB PEBBLEs (410–430nm). (B)Fluorescence spectra of oxygen probe and reference dye incorporated at different ratios into polystyrene exposed to air. (C)Fluorescence spectra are insensitive to pH. (D) Spectral response of the RGB PEBBLEs to nitrogen, air, and oxygen. (E) Changes in fluorescence intensity, reversibility and response time of the oxygen probe in the RGB PEBBLEs under cycling between nitrogen and oxygen. | |
The sensitivity of the RGB PEBBLEs was calculated from the quenching response:16
| 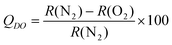 | (Eq. 1) |
where
R(N
2) is the fluorescence intensity ratio (
Iprobe/
Iref) without oxygen and
R(O
2) is the ratio under oxygen saturation. The quenching response was 74%. The response of the RGB PEBBLEs to molecular oxygen was standardised in atmospheres containing various parts of oxygen and
nitrogen (
Fig. 3A-D). It is important to note that oxygen probe and reference
dye embedded in the polystyrene beads are not subject to dissolved molecular oxygen in aqueous solution. Before molecular oxygen can interact with the oxygen probe it must leave the aqueous phase and enter the hydrophobic matrix. The oxygen partial pressure (
pO
2) in the gas phase and the oxygen concentration in
water ([O
2] in ppm) are in equilibrium (Henry's law). An atmospheric oxygen partial pressure of 21% corresponds to 8 ppm of dissolved oxygen at room temperature. Hence, oxygen in the gas phase was applied directly to the RGB PEBBLEs.
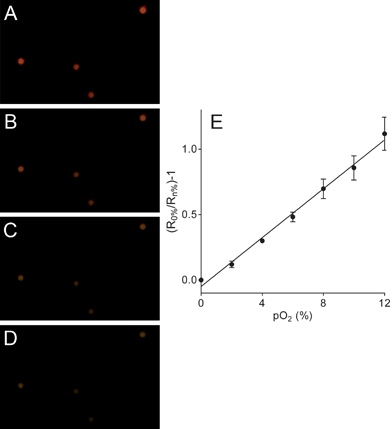 |
| Fig. 3
(A-D)
RGB images of RGB PEBBLEs with diameters of 500 nm at 0%, 4%, 8% or 12% oxygen partial pressure. (E) Linear Stern–Volmer plot of the ratio between the red and the green channel of individual RGB PEBBLEs at different oxygen partial pressures. The error bars represent standard deviations between individual RGB PEBBLEs. | |
Changes in the emission intensities of the PtTF20PP complex compared to the stable emission of the reference dye are clearly visible by eye in an RGB image. At low oxygen pressures the RGB PEBBLEs appear red because the red portion of the spectrum dominates. At increasing oxygen concentrations, the colour changes from red to green as the red intensity decreases. The Stern–Volmer equation relates the intensity ratios to the oxygen partial pressures or the concentration of dissolved oxygen, respectively:
| 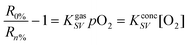 | (Eq. 2) |
where
KgasSV and
KconcSV are the respective Stern–Volmer constants in the gas phase and in solution.
R0% and
Rn% denote the ratio between the brightness values of the red channel (oxygen probe) to the green channel (reference
dye) at different oxygen partial pressures/concentrations.
Fig. 3E shows a Stern–Volmer plot for individual RGB PEBBLEs. At low oxygen partial pressures the Stern–Volmer plot is linear, indicating that the RGB PEBBLEs are especially useful for the sensitive measurement of low intracellular oxygen concentrations. At partial pressures higher than 12%, the standardisation is less accurate as the oxygen probe displays an exponential response to oxygen partial pressures (
Fig. 2B) with a higher sensitivity for changes in low than in high oxygen partial pressures.
RGB PEBBLEs with diameters of 100 nm, which are useful for high resolution imaging, were also prepared but cannot be analysed at the single bead level due to the diffraction limit of light microscopy (Fig. S.3 in the supplementary information†).
In vivo measurements of oxygen distributions
Intracellular
oxygen was measured in epithelial normal rat kidney (NRK) cells. The RGB PEBBLEs of 500 nm in diameter were easily incorporated into NRK cells by endocytosis.22 Real-time images of the intracellular oxygen distribution were acquired using an inverted epifluorescence microscope and a CMOS or CCD colour camera. While in previous work PEBBLEs were injected into the cytoplasm and in part into the nucleus by gene gun delivery,15 we chose the endocytotic pathway to observe the oxygen content of endosomes. The positively charged amino groups on the surface of the beads support cellular uptake by endocytosis.23Fig. 4A shows NRK cells shortly after adding RGB PEBBLEs. Most RGB PEBBLEs appear green in the oxygen saturated cell culture medium, from where they can be taken up by cellular endocytosis. The presence of a few RGB PEBBLEs displaying red luminescence indicates that the oxygen supply in endosomal compartments is low. The progress of endocytosis in Fig. 4B is clearly visible as more RGB PEBBLEs change their colour from green to red after 24 h. The magnified inset shows that the RGB PEBBLEs are mainly located on both sides of the cell membrane. Another picture of RGB PEBBLEs located inside and outside of cells is shown in Fig. S.4 (supplementary information†). After about 48 h (Fig. 4C), the endosomal compartments of NRK cells are highly loaded with RGB PEBBLEs. The endosomal compartments are confined to the cytoplasm such that no RGB PEBBLEs are located in the cell nucleus (counterstained with the DNA binding dye DAPI). When the cellular membranes were destroyed with the detergent Triton X-100,24 the RGB PEBBLEs were released from the cells and their colour turned from red to green in the oxygen-saturated culture medium (Fig. 4D).
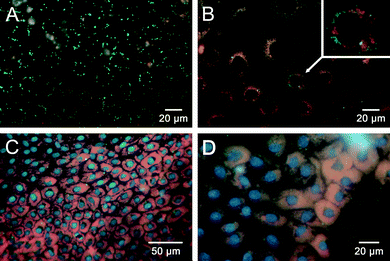 |
| Fig. 4 Imaging intracellular oxygen in NRK cells. (A) In the oxygen saturated cell culture medium, RGB PEBBLEs display green fluorescence. (B) After endocytosis (24 h), the colour of RGB PEBBLEs turns from green to red due to the limited oxygen supply in intracellular compartments. The inset shows an enlarged section of a single cell. (C) After 48 h, large amounts of the RGB PEBBLEs have been internalised by the cells. (D) Partial membrane rupture by 2% Triton X-100 releases some RGB PEBBLEs into the cell culture medium where their colour turns from red to green. | |
Visualising the oxygen distribution directly with RGB images (Fig. 5A) is difficult, as differences in colour intensities can result from an oxygen response as well as the number of RGB PEBBLEs in a cell. These errors of RGB images are corrected for if the ratio of the brightness values between the red (oxygen probe) and the green (reference dye) channel is calculated for each pixel in the image and plotted in pseudo-colours (Fig. 5B).18 The colour distribution in the ratiometric image indicates that the oxygen supply in intracellular compartments is not homogeneous.
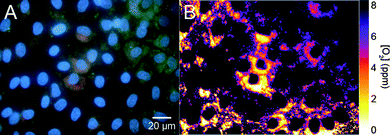 |
| Fig. 5
RGB image (A) and ratiometric image (B) of the intracellular oxygen distribution. The ratio of the brightness values between the red (oxygen probe) and the green (reference dye) channel is calculated for each pixel in the image and plotted in pseudo-colours. | |
Experimental
Synthesis of N-(5-carboxypentyl)-4-piperidino-1,8-naphthalimide butyl ester (reference dye)
All chemicals and solvents were of analytical grade and used as supplied by the manufacturers. 2.5 g (10 mmol) 4-chloro-1,8-naphtalic anhydride, 1.7 g (13 mmol) of 6-aminocaproic acid and a catalytic quantity of zinc acetate were dissolved in 70 mL DMF and stirred for 18 h at room temperature (21 ± 1 °C). The reaction mixture was heated to 80 °C for 1 h and then added to 350 mL water. After 2 h, a yellow precipitate had formed that was filtered and washed with cold water. The crude product was dissolved in 100 mL of boiling ethanol and filtered hot. The filtrate was left to cool over night to room temperature. The yellow crystals were filtered, washed three times with cold ethanol and dried in a desiccator over KOH. Then, 1.5 g (4.35 mmol) of the yellow crystals, 646 μL (6.52 mmol) of piperidine and 901 mg (6.52 mmol) of K2CO3 were dissolved in 30 mL DMF and stirred for 18 h at 90 °C. Cooled to room temperature, the solution was poured into 150 mL of cold water and after 2 h adjusted to pH 3 with HCl. The suspension was centrifuged, dried in a desiccator over CaCl2 and the product was purified by recrystallisation from ethanol. Yellow crystals were filtered off, washed with a small quantity of cold ethanol and dried at 50 °C. Finally, 400 mg (1.01 mmol) of these yellow crystals were suspended in 50 ml n-butanol, and 1 mL of concentrated sulfuric acid was added dropwise. The suspension was stirred at 90 °C for 24 h, cooled to room temperature and the volume was reduced to 15 mL. Ethyl acetate (50 ml) was added and the organic layer was washed two times with water, two times with a saturated solution of Na(HCO3)2 and one time with brine (100 mL each), dried over Na2SO4 and reduced to dryness. A yield of 322 mg of red oil containing some residual n-butanol was obtained. The chemical structure of the reference dye was confirmed by mass spectrometry (Finnigan MAT 95, Bremen, Germany): C27H34N2O4, HR-EI-MS: [M+., radical cation] 450.2519 (calculated), 450.2519 (found) and NMR spectrometry recorded on an Avance 300 MHz spectrometer (Bruker BioSpin, Rheinstetten, Germany). Chemical shifts (δ) are given in parts per million (ppm) using solvent signals as the reference. Coupling constants (J) are reported in Hertz (Hz). Splitting patterns are designated as s (singlet), d (doublet), t (triplet), dd (doublet doublet), m (multiplet): 1H-NMR (300 MHz, CDCl3) δ (ppm) 0.92 (t, 4H, J = 7.68 Hz), 1.36 (m, 3H), 1.45 (m, 2H), 1.59 (m, 3H), 1.72 (m, 6H), 1.88 (m, 4H), 2.31 (t, 2H, J = 7.41 Hz), 3.2 (t, 3H, J = 4.94 Hz), 4.05 (t, 2H, J = 6.59 Hz), 4.15 (t, 2H, J = 7.41 Hz), 7.18 (d, 1H, J = 7.96 Hz), 7.66 (t, 1H, J = 7.41 Hz), 8.38 (d, 1H, J = 8.5 Hz), 8.48 (d, 1H, 8.23 Hz), 8.56 (d, 1H, 7.13). 13C-NMR (300 MHz, CDCl3) δ (ppm) 13.73, 19.15, 24.36, 24.79, 26.24, 26.70, 27.83, 30.68, 34.30, 40.00, 54.56, 64.15, 114.72, 115.97, 123.15, 125.36, 126.28, 129.94, 130.60, 131.01, 132.67, 157.30, 164.12, 164.60, 173.82. The fluorophore N-isopropyl-4-piperidino-1,8-naphthalimide is a most viable reference dye, too. Both reference dyes are commercially available from Chromeon (Regensburg, Germany, www.chromeon.com).
Preparation and characterisation of RGB PEBBLEs
All experiments were performed at room temperature (21 ± 1 °C). Transmission electron microscopy was performed on a FEI Tecnai F30 instrument operated at 300 kV. Aqueous suspensions (2.5%) of amino-modified polystyrene beads with diameters of 500 nm or 100 nm were obtained from Polysciences Europe, Eppelheim, Germany). The bead suspension (500 μL) was mixed with 3.0 mL of distilled water and 1.5 mL of tetrahydrofurane (THF) to swell the polystyrene beads under ultrasonication for 10 min. Then, 300 μL of 2.0 mg mL−1 reference dye was mixed with 200 μL of 2.0 mg mL−1platinum(II) meso-tetrakis-(pentafluorophenyl)porphyrinato (PtTF20PP) complex (from Porphyrin Systems, Norderstedt, Germany), and added dropwise to the polystyrene bead suspension under continued ultrasonication. The mixture was exposed to ultrasonication for another 20 min to incorporate both dyes into the polystyrene beads. THF in the mixture was removed under reduced pressure. The RGB PEBBLEs were separated by centrifugation (8000 rpm for 10 min), washed with double distilled water containing 2%–5% (v/v) ethanol, and at least two times with double distilled water. Finally, a stock dispersion of 0.6% (w/v) RGB PEBBLEs in 2 mL double distilled water was prepared and stored at 4 °C. Fluorescence spectra of RGB PEBBLEs (typically 0.03% (w/v)) were acquired on an Aminco-Bowman AB2 luminescence spectrometer (Thermo Spectronic, Rochester, NY, USA) using an excitation wavelength of 405 nm. To test for dye leaching, the RGB PEBBLE stock dispersion was separated by centrifugation (8000 rpm for 10 min) and dispersed in either 67 mM phosphate buffered saline, pH 7.0 (PBS), PBS with 1% (w/v) NaCl, PBS with 2% (w/v) NaCl, ethanol or THF. After incubation for 12 h at room temperature, the dispersion was centrifuged as before, and the RGB PEBBLEs were dispersed in double distilled water and spectra were acquired. To test for protein interference, the RGB PEBBLE stock dispersion was separated by centrifugation (8000 rpm for 10 min), dispersed in PBS and the spectrum recorded. Then, human serum albumin (HSA) was added to yield a concentration of 1% (w/v) in the dispersion. After 15 min, the second spectrum was recorded.
Cellular imaging
For routine cell culture, normal rat kidney cells (NRK-52 E) from the German Collection of Microorganisms and Cell Cultures (DSMZ, Braunschweig, Germany) were kept in a humidified cell culture incubator at 37 °C in a 5% CO2 atmosphere. Cells were cultured in Dulbecco's modified Eagle's medium with 4.5 g L−1D-glucose containing 5% (v/v) of fetal calf serum, 100 μg mL−1penicillin/streptomycin, and 2 mM L-glutamine. The microscopic studies were performed with cells cultured on chambered coverglasses. Confluent cell monolayers were exposed to 1.14 × 109RGB PEBBLEs (500 nm diameter) per mL of cell culture medium for 6, 24, 48 or 72 h, respectively. Nuclei of living cells were stained with a 10 nM solution of 4′,6-diamidine-2-phenylindol (DAPI) for 15 min. RGB colour images were recorded at room temperature (21 ± 1 °C) on an inverted epifluorescence microscope (Nikon, Japan) equipped with a mercury arc lamp, an excitation bandwidth filter (330 to 380 nm), a 400-nm dichroic mirror, a longpass filter for emission wavelengths of >420 nm and either an EOS 50D CMOS camera (Canon, Japan) or a Coolpix 990 CCD camera (Nikon, Japan). Pseudo colour images were obtained using ImageJ software (U. S. National Institutes of Health, Bethesda, Maryland, USA).
Conclusions
Self-referenced RGB PEBBLEs are an excellent tool for imaging intracellular oxygen using conventional fluorescence microscopy. The hydrophobic polystyrene matrix retains two hydrophobic dyes, one for oxygen sensing and the other as an internal reference. These dyes have emission wavelengths that match either the sensitivity of the red or the green channel of RGB cameras and allow for ratiometric measurements based on single RGB images. The two dyes are for the most part protected from direct contact with cellular components such that quenching by intracellular components is largely prevented. Due to the amino-modified polystyrene surface, stable and mono-dispersed suspensions of RGB PEBBLEs in aqueous buffer solutions and cell culture media can be generated, which is a prerequisite for efficient cellular uptake without intracellular aggregation. The polystyrene matrix is highly permeable to oxygen (and other gases), such that the local concentration of cellular oxygen can be detected fast and reliably. As RGB PEBBLEs respond within seconds to changing oxygen concentrations, they will enable monitoring fast cellular reactions such as the dynamics of metabolism and cell respiration.
Acknowledgements
We thank Mr Heiko I. Siegmund of the Universitätsklinikum Regensburg for acquiring the TEM images and Dr Martin Link for useful discussions. Xu-dong Wang thanks the Deutscher Akademischer Austauschdienst (DAAD; Bonn) for a research fellowship.
Notes and references
- R. K. Bruick and S. L. McKnight, Science, 2002, 295, 807–808 CrossRef CAS.
- M. C. Hung, G. B. Mills and D. H. Yu, Nat. Med., 2009, 15, 246–247 CrossRef CAS.
- T. C. O'Riordan, A. V. Zhdanov, G. V. Ponomarev and D. B. Papkovsky, Anal. Chem., 2007, 79, 9414–9419 CrossRef CAS.
- Y. Will, J. Hynes, V. I. Ogurtsov and D. B. Papkovsky, Nat. Protoc., 2006, 1, 2563–2572 CAS.
- H. R. Christofk, M. G. Vander Heiden, M. H. Harris, A. Ramanathan, R. E. Gerszten, R. Wei, M. D. Fleming, S. L. Schreiber and L. C. Cantley, Nature, 2008, 452, 230–234 CrossRef CAS.
- X. D. Wang, H. X. Chen, Y. Zhao, X. Chen and X. R. Wang, TrAC, Trends Anal. Chem., 2010, 29, 319–338 CrossRef CAS.
- M. P. Coogan, J. B. Court, V. L. Gray, A. J. Hayes, S. H. Lloyd, C. O. Millet, S. J. A. Pope and D. Lloyd, Photochem. Photobiol. Sci., 2010, 9, 103–109 RSC.
- H. Malak, J. W. Dobrucki, M. M. Malak and H. M. Swartz, Biophys. J., 1998, 74, A189–A189.
- J. Ji, N. Rosenzweig, I. Jones and Z. Rosenzweig, J. Biomed. Opt., 2002, 7, 404–409 CrossRef CAS.
- T. Saito, N. Asakura, T. Kamachi and I. Okura, J. Porphyrins Phthalocyanines, 2007, 11, 160–164 CrossRef CAS.
- A. Fercher, G. V. Ponomarev, D. Yashunski and D. Papkovsky, Anal. Bioanal. Chem., 2010, 396, 1793–1803 CrossRef CAS.
- C. F. Wu, B. Bull, K. Christensen and J. McNeill, Angew. Chem., Int. Ed., 2009, 48, 2741–2745 CrossRef CAS.
- J. Ji, N. Rosenzweig, I. Jones and Z. Rosenzweig, Anal. Chem., 2001, 73, 3521–3527 CrossRef CAS.
- Y. E. K. Lee, R. Smith and R. Kopelman, Annu. Rev. Anal. Chem., 2009, 2, 57–76 Search PubMed.
- H. Xu, J. W. Aylott, R. Kopelman, T. J. Miller and M. A. Philbert, Anal. Chem., 2001, 73, 4124–4133 CrossRef CAS.
- Y. E. L. Koo, Y. F. Cao, R. Kopelman, S. M. Koo, M. Brasuel and M. A. Philbert, Anal. Chem., 2004, 76, 2498–2505 CrossRef CAS.
- Y. F. Cao, Y. E. L. Koo and R. Kopelman, Analyst, 2004, 129, 745–750 RSC.
- X. D. Wang, R. J. Meier, M. Link and O. S. Wolfbeis, Angew. Chem., Int. Ed., 2010, 49, 4907–4909 CrossRef CAS.
- S. W. Lai, Y. J. Hou, C. M. Che, H. L. Pang, K. Y. Wong, C. K. Chang and N. Y. Zhu, Inorg. Chem., 2004, 43, 3724–3732 CrossRef CAS.
- Y. Amao, Microchim. Acta, 2003, 143, 1–12 CrossRef CAS.
- D. B. Papkovsky and T. C. O'Riordan, J. Fluoresc., 2005, 15, 569–584 CrossRef CAS.
- S. Mukherjee, R. N. Ghosh and F. R. Maxfield, Physiol. Rev., 1997, 77, 759–803 CAS.
- M. Lundberg, S. Wikstrom and M. Johansson, Mol. Ther., 2003, 8, 143–150 CrossRef CAS.
- C. A. Schnaitman, J. Bacteriol., 1971, 108, 545–552 CAS.
Footnotes |
† Electronic supplementary information (ESI) available: Spectral response of CMOS camera, test for leaching and protein interference, images of 100-nm beads, microscope image of RGB PEBBLEs in cells, and video for Fig. 2E. See DOI: 10.1039/c0sc00610f |
‡ Current Address, Xiamen University, Department of Chemistry, Xiamen 361005, China |
|
This journal is © The Royal Society of Chemistry 2011 |
Click here to see how this site uses Cookies. View our privacy policy here.