DOI:
10.1039/C0SC00158A
(Edge Article)
Chem. Sci., 2010,
1, 271-275
New oligoethylene glycol linkers for the surface modification of an ultra-high frequency acoustic wave biosensor†
Received
1st February 2010
, Accepted 4th May 2010
First published on
15th June 2010
Abstract
This work describes the application of acoustic wave technology for the real-time and label-free detection of biotin–avidin interactions. Biosensing surfaces are constructed onto unelectroded piezoelectric quartz discs as functionalizable mixed self-assembled monolayers (SAM) produced from previously unreported linker and diluent molecules. Biotinthiol can subsequently be immobilized for detection purposes in a straightforward and coupling-free manner. Specific and non-specific adsorptions of avidin are measured at ultra-high frequencies (1.06 and 0.82 GHz) with an electromagnetic piezoelectric acoustic sensor (EMPAS) using micromolar avidin-spiked buffer solutions. These biosensing surfaces, especially the oligoethylene glycol SAM-based variety, display high specificity for avidin, with moderate to excellent reproducibility. This preliminary work constitutes the first application of SAM chemistry and EMPAS technology in the bioanalytical field.
Introduction
Biosensors are analytical devices that are employed for the transduction and detection of biochemical interactions occurring at the sensor–liquid interface.1 Although one-use structures are common, there is significant interest in devices that produce measurable signals in a real-time, ideally label-free manner. This type of detection technology constitutes a particularly attractive analytical tool that has received increasing attention over recent years with respect to environmental,2 food3 and drug analysis,4 detection aspects of biochemical warfare,5 and clinical diagnostics.6 However, before a biosensor can be definitively implemented as a reliable, commercially viable diagnostic device, there are a number of critical requirements that must be addressed. First, the attachment of the biosensing element to the transducer must be performed in a highly controlled fashion in terms of surface distribution and spatial orientation. Moreover, biological activity must be retained upon binding7 in order for the target analyte to interact efficiently with the surface-attached biochemical probe. Secondly, the device, ideally, should display both high specificity and sensitivity towards the target analyte and provide reliable and reproducible results, even in the presence of potentially interfering species. The undesired “non-specific adsorption” of adversary species (as opposed to the “specific adsorption” of the target analyte) has been a common and prevailing concern for some time with respect to the analysis of “real” biological samples such as blood, serum or urine. Accordingly, considerable attention has been paid to the role of adsorption effects and surface chemistry on biosensor response.
Self-assembling monolayer (SAM) chemistry is regularly regarded as a method of choice for the quick and economical preparation of structurally well-defined and customizable thin organic surfaces.8 SAM chemistry relies on the use of linking molecules that are engineered to spontaneously form ordered molecular assemblies on solid inorganic substrates.8 Moreover, functionalizable SAMs can also be designed to immobilize, in a subsequent step, various biomolecules such as proteins,9 antibodies,10 or oligonucleotides.11 Understandably, these attractive properties have endowed SAM chemistry with a unique position for the fabrication of biosensors.12
The conversion of biological events into measurable signals has required the development of new transducing technologies that are capable of being interfaced with appropriate surface chemistry in an intimate overall structure. Amongst the various transducing systems and devices that have been engineered,1 those based on acoustic wave physics that commonly rely on the unique piezoelectric properties of quartz,13,14 constitute an important, yet arguably underexploited14 technology for application in the bioanalytical field.15 The latest addition to the family of bulk acoustic wave devices, the electromagnetic piezoelectric acoustic sensor (EMPAS), which is based on the electromagnetic excitation of higher harmonics in the piezoelectric substrate, has been developed recently in our laboratory.16 In practice, EMPAS offers several major advantages over its predecessors, such as an electrode-free environment and the ability to conduct measurements at tunable, ultra-high frequencies (up to 1 GHz), which allows for detailed information and enhances sensitivity.17
In the present edge article, we describe the preparation of new functionalizable SAMs onto which biomolecules can be immobilized in a subsequent step18 with the ultimate goal of enhancing the development of the EMPAS-based biosensor. We report the construction of several novel, highly performing SAM-modified surfaces which are applied to the real-time and label-free EMPAS detection of biotin–avidin interactions,19 and the evaluation of their biosensing properties, in terms of specific and non-specific avidin adsorption. The nature of the linking molecules is expected to play an important role in the overall performance of the system in terms of response to non-specific adsorption vs. specific interaction.
Experimental
The following includes detailed protocols for quartz disc cleaning, mixed SAM formation, biotinthiol immobilization and EMPAS measurements. Anhydrous toluene for SAM formation and freshly distilled anhydrous DMF (from CaH2, under high vacuum) or spectrograde MeOH for biotinthiol immobilization were systematically used. OTS was distilled and stored in carefully sealed vials prior to use. Et3N was distilled from KOH. Avidin (from egg white, lyophilized powder) and Dulbecco's phosphate buffered saline (PBS) were purchased from Sigma-Aldrich®. Quartz crystals (AT-cut, 13.5 mm or 7 mm in diameter, 20 MHz fundamental frequency) were purchased from Lap-Tech Inc.®. Quartz crystal silanization (SAM formation) and biotinthiol immobilization reactions were prepared in a glovebox maintained under an inert (N2) and anhydrous (P2O5) atmosphere. The crystals were systematically handled with thoroughly pre-cleaned stainless steel tweezers in order to minimize any external contamination. EMPAS measurements were performed at either 1.06 GHz (53rd harmonic) or 0.82 GHz (41st harmonic).
Quartz crystal cleaning procedure
Quartz crystals (13.5 and 7 mm in diameter) were first sonicated in 20 mL of concentrated dishwashing soap for 30 min. The crystals were then thoroughly rinsed with hot water followed by distilled water then gently dried with forced air. Subsequently, the crystals were individually soaked in 6 mL of Piranha solution (3/1 (v/v) mixture of H2SO4 and 30% H2O2) pre-heated to 90 °C using a water bath (CAUTION: Piranha solutions are dangerous. Handle with care). After 30 min, the crystals were rinsed with distilled water (3×) followed by spectrograde methanol (3×). Next, the crystals were sonicated in spectrograde methanol for 2 min then individually transferred into vials, which were subsequently placed in an oven maintained at 150 °C for drying. After 2 h, the vials were immediately transferred into a humidity chamber, maintained at 60% relative humidity using a saturated solution of MgNO3·6H2O, and set aside overnight.
Silanization procedure (SAM formation)
Note:
to prevent any undesired reaction of our trichlorosilyl linkers/diluents with the walls of our test tubes during silanization, glassware was pre-treated with octadecyltrichlorosilane (1/20 (v/v) solution in anhydrous toluene) overnight.
Neat linker (TTTA, OEG-TTTA or OEG-TUBTS, 10 μL) and neat diluent (OTS or 7-OEG, 10 μL) were separately diluted with anhydrous toluene (10 mL). 500 μL of each solution were mixed in individual test tubes into which cleaned quartz crystals were then soaked. The test tubes were sealed with rubber stoppers, removed from the glovebox, and placed on a spinning plate for 2 h. The crystals were then rinsed twice with dry toluene and finally sonicated in toluene for 5 min. After a final rinse with one portion of toluene, the previous procedure was repeated with dry chloroform. Finally, the crystals were rinsed twice with dry chloroform, gently dried under forced air then individually transferred into vials for storage (SAM characterization or EMPAS controls) or immediately engaged in the subsequent biotinthiol immobilization procedure (EMPAS samples).
Immobilization of biotinthiol
Biotinthiol solutions (1.0 mg mL−1) for immobilization onto TTTA/OTS or OEG-TTTA/7-OEG mixed SAMs were prepared by dissolving biotinthiol into freshly distilled DMF. Biotinthiol solutions (1.0 mg mL−1) for immobilization onto OEG-TUBTS/7-OEG mixed SAMs were prepared by dissolving biotinthiol into MeOH or a 1/1 (v/v) MeOH–H2O mixture, to which Et3N (1 μL per mL of solvent) was also added. These solutions were portioned (1 mL) in dry test tubes into which freshly prepared mixed SAM-coated quartz crystals were soaked. The test tubes were sealed with rubber stoppers, removed from the glovebox and placed on a spinning plate overnight. The crystals were then rinsed three times with spectrograde methanol, dried under a gentle N2 stream then finally placed into screw cap vials under atmospheric conditions for EMPAS analysis.
EMPAS measurements
Avidin solutions (1.0 mg mL−1) were prepared by dissolving 1.0 mg of solid avidin into 1 mL of PBS buffer. After the standard set-up of EMPAS,17 biotinylated (or non-biotinylated) mixed SAM-coated quartz crystals were individually inserted into the flow through cell and PBS buffer was flown at a rate of 50 μL min−1. Once the frequency signal stabilized, 50 μL of a freshly prepared 0.1 mg mL−1 avidin-spiked PBS solution (90 μL of PBS buffer + 10 μL of 1.0 mg mL−1 avidin) were injected into the flow through system using a low-pressure chromatography valve. Once the avidin-spiked PBS solution completely passed over our surface, the uninterrupted PBS buffer flow rinsed the surface of any loosely bound material. The frequency signal stabilized again, the experiment was stopped and the frequency shift (for specific or non-specific avidin adsorption) was calculated.
Results and discussion
Our strategy first involved the preparation of robust and durable mixed SAMs onto hydroxylated AT-cut quartz discs using, in conjunction, combinations of unreported trichlorosilane linker and diluent molecules (Scheme 1). Both linkers and diluents possess a highly reactive trichlorosilyl tail function (Cl3Si–), for strong and robust covalent anchorage onto the underlying hydroxylated quartz surfaces,20 and an organic backbone to drive self-assembly and provide stability, rigidity and ordering to the mixed SAMs through intermolecular interactions.8c Linkers also possess reactive functionalizable head functions (trifluoroethyl ester (TFEE) or benzothiosulfonate (BTS)) for the subsequent site-specific covalent immobilization of a probe-biomolecule, biotinthiol (Scheme 2), onto the mixed SAMs. Diluents are shorter molecules where the purpose is to space out linker molecules within otherwise inherently denser and congested “undiluted” assemblies.21 This strategy decreases steric hindrance around neighbouring linker head functional groups. As a result, mixed SAMs were anticipated to offer enhanced binding ability (better reactivity and accessibility) for biomolecule immobilization and to facilitate access with regard to target analyte binding.7a,9,12a–c,22
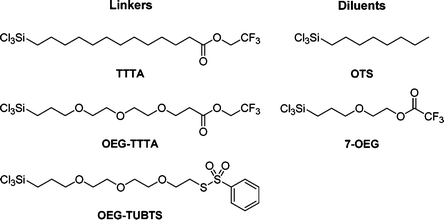 |
| Scheme 1 Linker and diluent chemical structures. | |
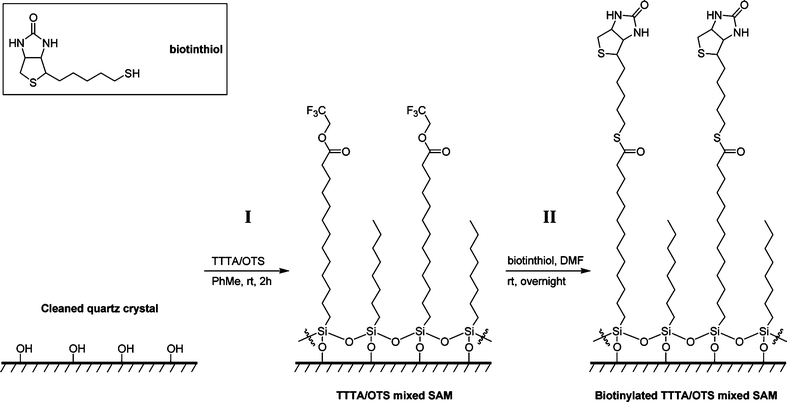 |
| Scheme 2 Illustration of the formation of a mixed SAM on a cleaned quartz crystal (step I) and the subsequent site-specific covalent immobilization of biotinthiol (step II): example with the TTTA/OTS linker/diluent system. OEG-TTTA/7-OEG and OEG-TUBTS/7-OEG systems follow the same scheme. | |
Three types of mixed SAMs (TTTA/OTS, OEG-TTTA/7-OEG, and OEG-TUBTS/7-OEG) were successfully prepared upon immersion of cleaned quartz discs (13.5 or 7 mm in diameter) into the appropriate 1/1/2000 (v/v/v) linker/diluent solutions in anhydrous toluene, for 2 h at room temperature (Scheme 2, step I). Mixed SAM characterization was achieved using contact angle goniometry (Table 1) and angle-resolved X-ray photoelectron spectroscopy (see ESI†). These SAMs were then biotinylated in a single, straightforward, and coupling-free step, upon immersion into 1.0 mg mL−1 solutions of biotinthiol in anhydrous DMF or MeOH, overnight at room temperature (Scheme 2, step II).
Table 1 Static contact angle measurements for TTTA/OTS, OEG-TTTA/7-OEG and OEG-TUBTS/7-OEG mixed SAMs recorded with ultrapure water.
Surface |
Contact angle/° |
TTTA/OTS SAM |
77 |
OEG-TTTA/7-OEG SAM |
69 |
OEG-TUBTS/7-OEG SAM |
75 |
Cleaned quartz disc |
12 |
For each linker/diluent system, EMPAS experiments involved two sets of independent frequency shift measurements (4 to 6 replicates per set), that were conducted at 1.06 GHz (13.5 mm discs) or 0.82 GHz (7 mm discs) using 0.1 mg mL−1 solutions of avidin in phosphate buffered saline (PBS). On the one hand, biotinylated mixed SAMs (samples) were dedicated to record specific adsorption of avidin to biotin. On the other hand, non-biotinylated mixed SAMs constituted controls that were dedicated to quantify non-specific adsorption of avidin. Specific to non-specific adsorption frequency shift ratios (RS/NS) and relative standard deviations (RSD) were then calculated to assess the efficiency of our biosensing surfaces to specifically detect avidin and the reproducibility of our measurements, respectively.
The TTTA/OTS system was first investigated on 13.5 mm discs (Chart 1, exp. 1) which resulted in very encouraging results since the specific adsorption frequency shifts (15 kHz) were significantly larger than the ones recorded for non-specific adsorption (4 kHz). The resulting RS/NS was excellent (3.8/1) and clearly demonstrated the ability of this system to detect avidin with high specificity. Although the reproducibility of our measurements was reasonable for specific adsorption (RSD = 14%), it was rather poor for non-specific adsorption (RSD = 39%).
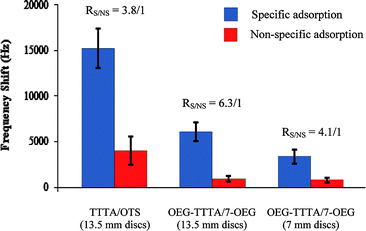 |
| Chart 1 EMPAS specific and non-specific avidin adsorption frequency shifts respectively measured with biotinylated and non-biotinylated TTTA/OTS and OEG-TTTA/7-OEG mixed SAMs, using 0.1 mg mL−1 avidin-spiked PBS solutions. EMPAS measurements were recorded at 1.06 GHz for the 13.5 mm discs and at 0.82 GHz for the 7 mm discs. | |
In order to improve the performance of the system with respect to specific vs. non-specific adsorption, we next prepared OEG-TTTA/7-OEG mixed SAMs, TTTA/OTS mixed SAM analogs that possess oligoethylene glycol (OEG) backbones. This choice was motivated by the fact that OEGylated SAMs have been reported to act as non-fouling surfaces that successfully resist undesired non-specific adsorption,23 a remarkable property that is currently drawing much attention in the bioanalytical field.21,24 This approach was highly successful since OEG-TTTA/7-OEG mixed SAMs did indeed allow us to substantially improve the performance of our device (with a RS/NS now reaching 6.3/1), confirming the general observation that OEGylated SAMs exhibit non-fouling properties (Chart 1, exp. 2). However, the limited reproducibility of the measurements was still a concern for both specific and non-specific adsorptions, with RSD values of respectively 16% and 33%. In view of the current trend towards biosensor miniaturization and in order to make our device even more attractive for future applications, we next prepared OEG-TTTA/7-OEG mixed SAMs on 7 mm discs—i.e. on surface areas four times smaller than those of the 13.5 mm discs (38 mm2vs. 142 mm2). This new experiment (Chart 1, exp. 3) was a success and provided us with an excellent, yet slightly lower, RS/NS of 4.1/1, indicating that our OEG-TTTA/7-OEG biosensing platforms could be considerably miniaturized while still maintaining high specificity for avidin. Reproducibility was still low for both specific (RSD = 23%) and non-specific (RSD = 33%) adsorptions.
As observed for the experimental results discussed above, the reproducibility of our measurements constituted a limitation to our otherwise highly performing TFEE biosensing surfaces. It was hypothesized that low reproducibility values essentially reflect the fact that the biosensing surfaces of a same series, although prepared under identical conditions, likely display different linker/diluent compositions and distributions, surface morphologies, and/or biotinthiol loadings; i.e. discrepancies in binding affinity for avidin. In order to better control biotinthiol loadings, we next sought a new generation of linkers able to reliably immobilize biotinthiol. We eventually synthesized the OEG-TUBTS linker (Scheme 1), an OEGylated molecule that possesses a BTS moiety known to readily and chemoselectively react with thiols to form disulfide bonds.25 BTS functions also tolerate aqueous and alcoholic media,25 which is particularly appreciable when probe-biomolecules are not soluble in aprotic organic solvents. It is also important to note that, to the best of our knowledge and despite these remarkable properties, BTS-based molecules have never been involved within SAM chemistry or for the development of biosensors. We first investigated OEG-TUBTS/7-OEG mixed SAMs (on 7 mm discs, at 0.82 GHz), for which biotinthiol had been immobilized in MeOH as the solvent (Chart 2, exp. 2). These also exhibited the ability to detect avidin but with lower specificity (RS/NS of 2.0/1) compared to the corresponding TFEE SAMs (Chart 2, exp. 1). Gratifyingly, however, and for the first time in our study, reproducibility was excellent, for both specific (RSD = 4%) and non-specific (RSD = 6%) adsorptions.
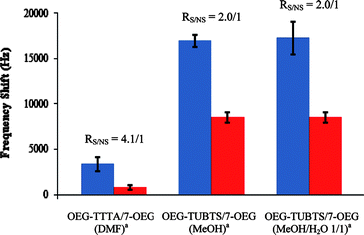 |
| Chart 2 EMPAS specific (blue) and non-specific (red) avidin adsorption frequency shifts respectively measured with biotinylated and non-biotinylated OEG-TUBTS/7-OEG mixed SAMs, using 0.1 mg mL−1 avidin-spiked PBS solutions, and comparison with the OEG-TTTA/7-OEG system. EMPAS measurements were recorded at 0.82 GHz on 7 mm discs. a Solvent(s) used for biotinthiol immobilization. | |
It is also interesting to note that, in comparison with the TFEE OEGylated mixed SAMs, both reproducibility and frequency shift intensities were considerably greater. For non-specific adsorption, this seems to indicate that BTS OEGylated mixed SAMs are more uniform and display comparatively higher affinity for avidin. With respect to specific adsorption, this strongly supports a higher, more reliably controlled and homogeneous site-specific biotinthiol coverage. Finally, it was necessary to determine whether specific adsorption is affected by immobilizing biotinthiol under mild aqueous conditions. For that purpose, we biotinylated OEG-TUBTS/7-OEG mixed SAMs in a 1/1 (v/v) MeOH–H2O mixture.26 It is noteworthy that neither the RS/NS (still 2.0/1) nor the RSD value for specific adsorption (10%) were significantly modified (Chart 2, exp. 3), demonstrating that biotinthiol can also be reproducibly immobilized in an aqueous solvent mixture without altering the performance of the EMPAS biosensor.
Conclusions
In this edge article, we have presented the construction of several novel, highly performing mixed SAM-based piezoelectric biosensors able to detect biotin–avidin interactions in a real-time and label-free manner using the electromagnetic piezoelectric acoustic sensor (EMPAS). This work constitutes the first application of SAM chemistry and EMPAS technology in the bioanalytical field. Biosensing surfaces were built onto piezoelectric AT-cut quartz discs as robust, durable and functionalizable mixed SAMs—using previously unreported trichlorosilane linker and diluent molecules—onto which biotinthiol could subsequently immobilize in a single, straightforward, and coupling-free step through TFEE or unprecedented BTS head functions. The biosensing properties of these assemblies, in terms of specific and non-specific avidin adsorptions, were measured with EMPAS at ultra-high frequencies (1.06 and 0.82 GHz) using micromolar avidin-spiked PBS buffer solutions. With respect to TFEE head function, biotinylated mixed SAMs efficaciously bound avidin, whereas non-biotinylated ones only exhibited limited binding affinities for avidin. Specific to non-specific avidin adsorption ratios were excellent and systematically improved with OEGylated mixed SAMs but the low reproducibility of our measurements was a recurrent problem. In comparison, BTS OEGylated mixed SAMs exhibited excellent reproducibility but lower specificity towards avidin. During this study, we also showed that the overall biosensing platform could be reduced in size while still maintaining high specificity for avidin (TFEE system) and that biotinthiol immobilization could also be performed under mild aqueous conditions without altering the performance of the sensor (BTS system). Research attention is currently focused on developing OEGylated mixed SAMs that would combine the high specificity displayed by TFEE systems and the excellent reproducibility obtained with BTS OEGylated mixed SAMs. It is also planned to progress from using simple buffered target analyte solutions to complex biological fluids such as serum, urine and blood. In these sample matrices, target analytes will be present at low concentration and will have to be distinguished from relatively high concentrations of competing species.
Acknowledgements
The authors are grateful to the Natural Sciences and Engineering Research Council of Canada (NSERC) for partial support of their work on acoustic wave-based biosensors. The authors also thank Drs Peter Brodersen and Rana Sodhi from Surface Interface Ontario for XPS analysis.
Notes and references
-
(a)
B. R. Eggins, Chemical Sensors and Biosensors, John Wiley and Sons Ltd., Chichester, UK, 2002 Search PubMed
;
(b) A. F. Collings and F. Caruso, Rep. Prog. Phys., 1997, 60, 1397 CrossRef CAS
.
- O. A. Sadik, M. M. Ngundi and F. Yan, Biotechnol. Bioprocess Eng., 2000, 5, 407 Search PubMed
.
-
M. Del Carlo, M. Nistor, D. Campagnone, B. Mattiasson and E. Csoregi, Food Biotechnology, 2nd edn, ed. K. Shetty, G. Paliyath, A. Pometto and R. E. Levin, Taylor and Francis Group, Boca Raton, Florida, USA, 2006, p. 1567 Search PubMed
.
- D. Yu, B. Blankert, J.-C. Vire and J.-M. Kauffmann, Anal. Lett., 2005, 38, 1687 CrossRef CAS
.
- J. J. Gooding, Anal. Chim. Acta, 2006, 559, 137 CrossRef CAS
.
-
V. C. Yang and T. T. Ngo, Biosensors and Their Applications, Kluwer Academic/Plenum Publishers, New York, USA, 2000 Search PubMed
.
-
(a) F. Rusmini, Z. Y. Zhong and J. Feijen, Biomacromolecules, 2007, 8, 1775 CrossRef CAS
;
(b) D. C. Kim and D. J. Kang, Sensors, 2008, 8, 6605 CrossRef CAS
and references therein.
-
(a) C. Vericat, M. E. Vela, G. A. Benitez, J. A. Martin Gago, X. Torrelles and R. C. Salvarezza, J. Phys.: Condens. Matter, 2006, 18, R867 CrossRef CAS
;
(b)
R. Shenhar, T. B. Norsten and V. M. Rotello, Introduction to Nanoscale Science and Technology, ed. M. Di Ventra, S. Evoy and J. R. Heflin, Springer, New York, USA, 2004, p. 41 Search PubMed
;
(c) A. Ulman, Chem. Rev., 1996, 96, 1533 CrossRef CAS
.
-
(a) J. A. Camarero, Biophys. Rev. Lett., 2006, 1, 1 CrossRef CAS
;
(b) Y. W. Lee, J. Reed-Mundell, J. E. Zull and C. N. Sukenik, Langmuir, 1993, 9, 3009 CrossRef CAS
.
- A. Azioune, J.-J. Pireaux and L. Houssiau, Appl. Surf. Sci., 2004, 231–232, 402 CrossRef CAS
.
-
(a) L. A. Chrisey, G. U. Lee and C. E. O'Ferrall, Nucleic Acids Res., 1996, 24, 3031 CrossRef CAS
;
(b) M. Saoud, C. Blaszykowski, S. M. Ballantyne and M. Thompson, Analyst, 2009, 134, 835 RSC
.
-
(a) T. Wink, S. J. van Zuilen, A. Bult and W. P. van Bennekom, Analyst, 1997, 122, 43R RSC
;
(b) N. K. Chaki and K. Vijayamohanan, Biosens. Bioelectron., 2002, 17, 1 CrossRef CAS
;
(c) F. Luderer and U. Walschus, Top. Curr. Chem., 2005, 260, 37 CAS
;
(d) S. Ferretti, S. Paynter, D. A. Russell, K. E. Sapsford and D. J. Richardson, Trends Anal. Chem., 2000, 19, 530 CrossRef CAS
;
(e)
B. Liedberg and J. M. Cooper, Immobilized Biomolecules in Analysis: A Practical Approach, ed. T. Cass and F. S. Ligler, Oxford University Press, Oxford, UK, 1998, p. 55 Search PubMed
.
-
(a) G. G. Guilbault, J. M. Jordan and E. Scheide, CRC Crit. Rev. Anal. Chem., 1988, 19, 1 CAS
;
(b) M. R. Deakin and D. A. Buttry, Anal. Chem., 1989, 61, 1147A CAS
.
-
(a) S. Sheikh, C. Blaszykowski and M. Thompson, Anal. Lett., 2008, 41, 2525 CrossRef CAS
;
(b) T. M. A. Gronewold, Anal. Chim. Acta, 2007, 603, 119 CrossRef CAS
.
- Selected reviews:
(a)
J. Rickert, W. Gopel, G. L. Hayward, B. A. Čavić and M. Thompson, Sensors Update (Volume 5), ed. H. Baltes, W. Gopel and J. Hesse, Wiley-VCH, Weinheim, Germany, 1999, p. 105 Search PubMed
;
(b) B. A. Čavić, G. L. Hayward and M. Thompson, Analyst, 1999, 124, 1405 RSC
;
(c) M. A. Cooper and V. T. Singleton, J. Mol. Recognit., 2007, 20, 154 CrossRef CAS
;
(d) K. Länge, B. E. Rapp and M. Rapp, Anal. Bioanal. Chem., 2008, 391, 1509 CrossRef CAS
.
-
(a) M. Thompson, S. M. Ballantyne, L.-E. Cheran, A. C. Stevenson and C. R. Lowe, Analyst, 2003, 128, 1048 RSC
;
(b)
M. Thompson and S. M. Ballantyne, Electromagnetic piezoelectric acoustic sensor, US Patent 7207222, 2007
.
- S. M. Ballantyne and M. Thompson, Analyst, 2004, 129, 219 RSC
.
-
(a) M. E. McGovern and M. Thompson, Anal. Commun., 1998, 35, 391 RSC
;
(b) M. E. McGovern and M. Thompson, Can. J. Chem., 1999, 77, 1678 CrossRef CAS
.
- Avidin is well-known for exhibiting very high affinity towards biotin (Ka ∼ 1015 M−1). This feature made the biotin–avidin system a valuable model for testing the viability of our biosensors. The interactive biotinyl residue was introduced onto the mixed SAMs upon immobilization of our probe-biomolecule, biotinthiol. For a comprehensive handbook on biotin–avidin chemistry and its various applications in the bioanalytical field, see:
M. D. Savage, G. Mattson, S. Desai, G. W. Nielander, S. Morgensen and E. J. Conklin, Avidin-Biotin Chemistry: A Handbook, Pierce Chemical Company, Rockford, Illinois, USA, 1992 Search PubMed
.
- S. R. Wasserman, Y.-T. Tao and G. M. Whitesides, Langmuir, 1989, 5, 1074 CrossRef CAS
.
- G. E. Fryxell, P. C. Rieke, L. L. Wood, M. H. Engelhard, R. E. Williford, G. L. Graff, A. A. Campbell, R. J. Wiacek, L. Lee and A. Halverson, Langmuir, 1996, 12, 5064 CrossRef CAS
.
- J. W. Lee, S. J. Sim, S. M. Cho and J. Lee, Biosens. Bioelectron., 2005, 20, 1422 CrossRef CAS
and references therein.
-
(a)
A. S. Anderson, A. M. Dattelbaum, H. Mukundan, D. N. Price, W. K. Grace and B. I. Swanson, Proceedings of SPIE, Frontiers in Pathogen Detection: From Nanosensors to Systems (Films and Substrates), ed. M. Fauchet, 2009, 7167, 7167Q1 Search PubMed
. See also:
(b) J. Zheng, L. Li, H.-K. Tsao, Y.-J. Sheng, S. Chen and S. Jiang, Biophys. J., 2005, 89, 158 CrossRef
.
-
(a) E. Ostuni, R. G. Chapman, R. E. Holmlin, S. Takayama and G. M. Whitesides, Langmuir, 2001, 17, 5605 CrossRef CAS
;
(b) N. Ngadi, J. Abrahamson, C. Fee and K. Morison, WASET: Engineering and Technology (Proceedings), 2009, 49, 144 Search PubMed
;
(c) G. M. B. F. Snellings, S. O. Vansteenkiste, S. I. Corneillie, M. C. Davies and E. H. Schacht, Adv. Mater., 2000, 12, 1959 CrossRef CAS
;
(d) E. Ostuni, R. G. Chapman, M. N. Liang, G. Meluleni, G. Pier, D. E. Ingber and G. M. Whitesides, Langmuir, 2001, 17, 6336 CrossRef CAS
;
(e) N. Xia, Y. Hu, D. W. Grainger and D. G. Castner, Langmuir, 2002, 18, 3255 CrossRef CAS
;
(f) S. I. Jeon, J. H. Lee, J. D. Andrade and P.-G. De Gennes, J. Colloid Interface
Sci., 1991, 142, 149 CrossRef CAS
.
-
(a) T. F. Parsons, J. D. Buckman, D. E. Pearson and L. Field, J. Org. Chem., 1965, 30, 1923 CrossRef CAS
;
(b) J. L. Kice and T. E. Rogers, J. Am. Chem. Soc., 1974, 96, 8015 CrossRef CAS
.
- Biotinthiol is poorly soluble in water.
|
This journal is © The Royal Society of Chemistry 2010 |