DOI:
10.1039/B811063H
(Critical Review)
J. Environ. Monit., 2009,
11, 27-40
JEM Spotlight: Applications of advanced nanomaterials for environmental monitoring
Received
2nd July 2008
, Accepted 9th October 2008
First published on 6th November 2008
Abstract
Rapid progress of the nanotechnology and advanced nanomaterials production offers significant opportunities for a wide range of applications for detection and remediation of a broad range of environmental contaminants. The convergence of analytical techniques and nanotechnology provides attractive possibilities for development of miniaturized, rapid, ultrasensitive and inexpensive methods for in situ and field-based environmental monitoring devices. This review provides an overview of the various nanoparticles and nanostructures used for this purpose, their integration into functional analytical devices, applications as electrode materials and gas sensing nanoprobes, in biosensors and as capture probes in immunomagnetic separations. Relevant, specific examples of nanomaterials-based chemical and biological sensors with applications in environmental monitoring are discussed.
1. Introduction
Nanoparticles (NPs) and nanostructures are those materials whose structural elements have been engineered at the nanometre scale (dimensions of the order of or below 100 nm),1 and possessing completely new properties as compared to the bulk material. Examples of advanced nanomaterials include metallic, semiconductor and ceramic nanoparticles, nanowires, nanotubes and nanorods as well as composites of these materials. The uniqueness of these materials is due to their mechanical, electrical, optical, catalytic, magnetic and photonic properties, and an extremely high surface area. In the field of environmental monitoring, these properties offer opportunities for a wide range of applications for detection and remediation of different contaminants and toxins.2–6 Other environmental applications are in wastewater treatment and catalysis, for example, as catalysts for noxious and toxic gases.
The convergence of analytical techniques and nanotechnology provides opportunities for development of miniaturized, rapid, ultrasensitive and inexpensive methods for in situ and field-based environmental monitoring. Numerous papers reported the use of nanoscale materials for the construction of gas sensors,2–4enzyme sensors, immunosensors and genosensors, for direct wiring of enzymes at electrode surfaces and for signal amplification as labels in electrochemical, optical or photoelectrochemical phenomena.7,8 For example, metal oxide NPs have excellent catalytic properties, making them suitable for the construction of enzymeless electrochemical sensors.9–13 Magnetic iron oxide nanostructures have recently gained a great deal of attention due to their potential for providing control of electrochemical processes,14,15 creating magneto-switchable devices16,17 and in immunomagnetic concentration/separation. Biological recognition elements can be attached to nanomaterial surfaces to develop various catalytic and affinity biosensors. Several metal oxide nanostructures were used as gas sensing nanoprobes.18,19
Extensive research papers and reviews covering the synthesis and characterization of various nanostructured materials were published in the literature in recent years. In this review, we focus on the most important and widely used advanced nanomaterials (i.e.carbon nanotubes, nanofibers and nanowires, metal/metal oxide nanoparticles, nanocomposite, quantum dots, nanoporous adsorbents and dendrimers), their integration in functional analytical devices and discuss the success and limitations of these materials in environmental monitoring, focusing on chemical and biological sensing. Their use as electrode materials, immobilization matrices, selective gas sensing probes, magnetic capture probes and specific examples in the environment are reviewed.
2. Types of nanomaterials in environmental monitoring
Table 1 summarizes the most relevant examples of advanced nanomaterials and nanostructures, their characteristics and potential applications in environmental monitoring reported in literature. Specific examples are reviewed in this section.
Table 1 Examples of advanced nanomaterials for environmental monitoring
Nanomaterials |
Types |
Properties |
Potential applications |
Ref. |
Carbon nanotubes
|
Single and multi walled with various diameters and length |
Electrocatalytic activity; High adsorption capacity |
Sorbents |
23–25,35,179
|
Various types of chemical and biological sensors |
Metal nanoparticles |
Au, Ag of various shapes and dimensions |
Electrocatalytic activity; Ag has antibacterial activity |
Sorbents |
48–55
|
Various types of chemical and biological sensors |
Magnetic iron oxide |
Fe2O3, Fe3O4, Fe3S4, MeO-Fe2O3 where M = Ni, Co, Zn,… etc.) |
Superparamagnetic; |
Immunomagnetic separation and concentration of target analytes |
48–55
|
Catalytic sites for H2O2 |
Semiconductor metal oxides |
TiO2, ZnO, ZrO2, CeO2, |
Photocatalytic, antibacterial and electrocatalytic activity |
Photocatalytic damage |
18,63,79,180–183
|
Gas sensing probes and biosensing |
Quantum Dots
|
Inorganic fluorophores with intensity varying with the size and composition |
Photoluminexcence; Can be biofuntionalized with enzymes, DNA and Ab |
Detection based on the changes in changes in the photoluminescence intensity |
93–97
|
Dendrimers
|
Hyperbranched nanostructures with different length and nanometre size branches |
High surface area with functionalized end groups; High sorption capacity |
DNA microarrays and DNA sensors; |
98–100
|
Composition of filters in waste water treatment |
Since their discovery in 1991,20carbon nanotubes (CNTs) have been extensively studied as nanoscale building blocks for analytical devices.21 Typically, CNTs are divided into two categories: single walled carbon nanotubes (SWNTs) having a diameter of ∼1.5 nm and multiwalled carbon nanotubes (MWNTs), consisting of 2–30 concentric layers of graphite with diameters ranging from 3–50 nm. The length of CNTs varies from nanometres to several micrometres.22 Their nano dimensions, surface chemistry, extremely high surface area, and the electronic properties make CNTs an ideal candidate for chemical and biochemical sensing.23 Other important characteristics include their ability to enhance the binding of biomolecules,24 favorize direct electronic communication between redox proteins and electrode surfaces and increase the electrocatalytic activity. This allowed detection of several analytes (e.g.pesticides) at a low-applied potential without the use of electronic mediators, thus reducing the effect of interferences.25,26 Enzymes such as acetylcholinesterase (AChE)25,26 and organophosphate hydrolyse (OPH)27 were immobilized within CNTs/hybrid composites (mainly polymeric based) and used to construct electrochemical biosensors.28–34 Different strategies for the functionalization of CNTs for sensing and biosensing applications have been described in other works.23,35 These sensors showed superior performance in terms of sensitivity, applied potential and surface fouling as compared to those based on macroscale materials. Examples of environmental applications include monitoring of organophosphorus pesticides,25–27 phenolic compounds30–34 and herbicides.29
Metal NPs (Au, Pt or Cu) can be incorporated in CNTs/polymeric composites to further enhance their characteristics. Such nanoassemblies were used in electrochemical sensors for the detection of nitroaromatic explosives, with a detection limit of 1 ppb TNT in soil, a wide linear range of up to 2000 ppb and a reproducibility of ∼ 15%.36 Similarly, magnetic NPs can also be attached to the CNTs surface to create a unique ‘magneto-nanotubes’ structure in which electrocatalytic and magnetic properties can be combined, making it possible to control the entire assembly in a magnetic field and creating opportunities developing magneto-switchable devices.37
Metal NPs, such as gold, silver and iron, constitute one of the most researched branches of nanotechnology due to their electronic, optical, catalytic and thermal properties.38,39 Among those, Ag NPs are known for their antimicrobial activity and have been used in water treatment.40–43 Their bactericidal activity is shape and size dependent, with particles of sizes less than 100 nm showing optimal antibacterial activity. Another application is as oligonucleotide labeling tags in electrochemical DNA sensors.44 For example, Au NPs have been intensively used for signal amplification, as a component of bio-immobilization matrixes in biosensors and in a variety of colorimetric and fluorescence assays. A miniaturized battery operated nanomaterial surface energy transfer (NSET) probe designed for detection and screening of mercury in soil, water and fish, with detection limits in the ppt range is an example of this approach.45 In the NSET system, AuNPs (acting as an acceptor) were combined with an organic dye (Rhodamine) as a donor. The method is based on the quenching properties of AuNPs through energy- and electron-transfer, providing enhanced sensitivity for the detection of metal ions. In the presence of Hg, the self-adsorbed dye molecules are released from the NPs surface, generating distinct changes in the fluorescence signal, proportional with the concentration of Hg(II). Fig. 1A illustrates the TEM of Au NPs in the presence and absence of mercury and Fig. 1B shows the fluorescence changes of Rhodamine B adsorbed onto Au–NPs in the presence of different concentration of Hg(II).45 Other NPs such as zero valent iron and bimetallic iron NPs coupled to metal catalysts such as Pd, Pt or Ni were used in environmental remediation of chlorinated organics, polychlorinated biphenyls (PCBs),46 inorganic ions and heavy metal ions such as As(III), Pb(II), Cu(II), Ni(II) and Cr(VI).47
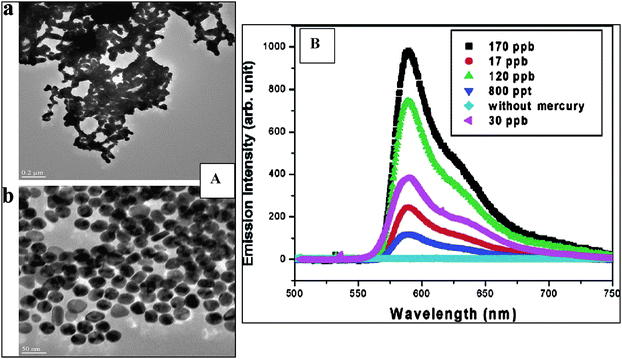 |
| Fig. 1
A: TEM of Au NPs in the presence and absence of 130 ppm Hg(II). B: Changes in the fluorescence spectra of Rhodamine B adsorbed onto Au NPs with different concentrations of Hg(II) (from reference 45 with permission from the American Chemical Society). | |
Magnetic iron oxide NPs provide attractive possibilities in many environmental applications, such as remediation, biosensing, cell labeling and separations.48–55 These applications require NPs to be well-dispersed in liquid media, chemically stable, and uniform in size. Significant progress was made in the control of size, size distribution, shape, and chemical composition of magnetic NPs in recent years.56,57 These are readily available in different forms (Fe2O3, Fe3O4, Fe3S4, MeO–Fe2O3 where M = Ni, Co, Zn, … etc.) and can be purchased from several companies or, they can be prepared using established synthetic procedures.55 An interesting application of these nanomaterials is as capture-probes in immunomagnetic separations, in which immuno-functionalized magnetic NPs first bind and concentrate the target analyte from the sample, and then separate it using a magnetic field. This approach could be used to simplify the sample preparation process and facilitate detection of target analytes with very low detection limits. Moreover, environmental contaminants can be adsorbed onto the particles surface and then be easily separated from the medium using a magnet.58 For example, it was found that arsenic can be effectively and economically removed under low magnetic fields (∼1T) when adsorbed onto iron oxide nanoparticles.59 Similarly, biomolecules attached on magnetic NPs carriers can be separated from the medium and directed to a particular site.60 In the biosensors field, the electrocatalytic properties of various ferric oxides (hematite, maghemite, amorphous Fe2O3, β- Fe2O3 and ferrihydrite) at a glassy carbon electrode (GCE) showed performance characteristics comparable with those of Prussian Blue modified electrodes.10
Since the discovery of the photocatalytic properties of titanium dioxide (TiO2) various semiconductor photocatalysts were investigated and utilized for environmental applications. These include ZnO, SnO2, WO3,61 MnO211,12 and recently CeO2.62 Nano-TiO2 of different forms and shapes such as spheres, wires and hollow spheres as well as high surface area nanostructured TiO2 films were produced.63,64 Several literature reports discussed synthesis of uniform TiO2 NPs with desired surface functionalities.65–67 Most applications of TiO2 are in catalysis and photocatalysis, photovoltaic cells, as dielectric gate materials, and in photo-assisted degradation of organic molecules. For example, commercially available TiO2 photocatalysts (e.g. Degussa P-25) were widely used in photodegradation of aqueous organic contaminants such as organic dyes, pesticides and phenols47,68 and as materials in gas sensors for CO, NO and CH4.69,70 Doped TiO2 nanomaterials (e.g.Al, Pt, S) were used in water and soil remediation, as biocidal agents that kill bacteria, fungi, algae and viruses.47,71 Efficiencies of 99% for EscherichiaColi47 and 95% for Micrococus tylae71 were obtained after exposure times of 30 min and 1 hr. In addition to TiO2, another semiconductor, CeO2 was used in heterogeneous catalysis, gas sensing and in environmental remediation for the catalytic oxidation of different hydrocarbons.72,73 Its sensing properties were attributed to its good structural stability, high oxygen mobility and predominant ionic defects which act as oxygen traps. TiO2 doped CeO2catalysts were used in catalytic wet oxidation of phenol.74 In another application, ZnO was reported to simultaneously sense and degrade organic contaminants such as 4-chlorocatechol in water.75 Coupling of photocatalytic degradation and sensing offers interesting opportunities for further developments in the field of environmental monitoring.
Several biosensing applications of metal oxide NPs, mainly in electrochemical sensors, were also reported.76–78 For example, MnO2 and CeO2 have catalytic active sites for hydrogen peroxide sensing,11,12,79 which is the product of many enzymatic reactions80,81 and a key component in various environmental processes. Recently, we studied the electrocatalytic activity of CeO2 towards H2O2 and developed a ceria based enzymeless hydrogen peroxide sensor.79
2.5. Nanocomposite materials and nanoporous sorbents
Nanocomposite materials are prepared by combining nanometre size materials (e.g. CNTs, metal NPs) with sol-gels, polymers or other linkers and could contain catalysts, mediators and stabilizers. This allows fabrication of advanced materials with enhanced characteristics tailored towards a particular application or a specific environmental target.82 These materials have been proved to be very effective in several environmental applications, as sorbents for aromatic compounds,83 filters for water purification84 and in a variety of biosensing applications.28–34,84,85
Nanoporous sorbents, such as zeolites, consisting of a highly ordered structure of aluminium (Al), silicon (Si) and oxygen (O2), have attracted considerable interest in the environmental field. In general, these are crystalline porous materials,86 whose surface properties can be manipulated by varying the Si/Al ratio during synthesis. This allowed fabrication of nanostructures with tunable ion-exchange capacity and hydrophobic or organophilic adsorptive properties.87,88 In addition to their use as sorbents for environmental pollutants (e.g. NO2, toluene, hydrocarbons) these materials also found applications in sensing.89,90
Quantum dots (QDs) are colloidal inorganic nanocrystals fluorophores with unique photophysical characteristics. Their optical and spectroscopic properties depend on size, composition and surface states and thus, any physical or chemical perturbation results in fluorescence suppression. In the environmental field, QDs were used as sensing probes for small metal ions,91,92pesticides,93,94 phenols95 and nitroaromatic explosives.96 These are based on analyte-induced deactivation or activation of photo-luminescence, all of which are easily influenced by the surface coating. Core-shell structures obtained by coating the QDs with higher band gap inorganic semiconductor materials, such as (CdSe)ZnS, showed an increase in the photoluminescence quantum yield and in their chemical and photo-stability. Examples of QDs used for metal analysis include CdS for Cu(II), Ag(II), I−; CdSe for Cu(II), Ag(I), CN−, triethylamine, benzylamine; CdS–ZnS and CdTe for Cu(II).92 QDs can also be coupled with enzymes to obtain QDs-enzyme conjugates that are responsive to enzyme substrates and inhibitors93,94 and with antibodies.96 Such an approach was used to fabricate AChE-labeled CdS97 and OPH-(CdSe)ZnS93,94 for the detection of pesticides. The quantification signal was based on changes in photoluminescence intensity of the QDs bioconjugate in the presence of the analyte (e.g.paraoxon).93,94 QDs coupled to antibody allowed detection of trinitrotoluene (TNT) in an aqueous environment using fluorescence energy transfer (FRET).96
2.7.
Dendrimers
Dendrimers or dentritic polymers, consisting of well defined hyperbranched nanostructures with different length and a number of branches with nanometre sizes and with functionalized end groups,98 are actively studied for environmental and industrial applications.99 For example, they were used as high capacity nanochelating agents for the removal and recovery of heavy metals in water purification systems100 and in the composition of filters impregnated in ceramic membranes for the removal of organic compounds.84 Other applications are in bioassays and biosensing as support for DNA probes for improving hybridization efficiency or, as labels to improve detection capabilities.98
3. Environmental applications of advanced nanomaterials: assembly in functional analytical devices
Environmental applications of advanced nanomaterials can be divided into two main directions: (i) remediation and treatment in which nanomaterials act as adsorption and/or degradation sites of contaminants and (ii) integrated into functional detection/sensing devices that respond to a particular (or class) of environmental targets. In the later situation, they are used to enhance the sensitivity, selectivity, response time and stability of these devices. Nanomaterials used for environmental remediation purposes include: (1) magnetic iron oxides for removal of contaminants such as As(III), As(V), Cu(II), dichlorophenol and synthetic organic compounds,101 (2) carbon nanotubes for adsorption of toxic aromatic compoundsvia the π-electron interactions between the graphene surface and the benzene moiety,102,103 and more recently, of toxins like dioxin and microcystins,104 (3) TiO2 nanocomposites105–109 for photodegradation of numerous organic molecules, cells and viruses, under UV irradiation, through a photodegradation mechanism involving free radicals and (4) Ag NPs for inactivation of bacteria and viruses.40
In addition to their remediation capabilities, the coupling of advanced nanostructured materials with physical transducers and biomolecular recognition elements brought new directions in the environmental monitoring arena. Various chemical and biological sensors have been developed, allowing selective and highly sensitive detection of a broad range of environmental analytes. Most of these devices are electrochemical sensors due to their simplicity, reduced size, low cost and easy assembly in field-deployable systems.110–115 Optical assays (e.g. based on optical phenomena like QDs photoluminescence quenching) were also reported. These devices are developed as a reliable alternatives to classical methods currently used in analytical chemistry laboratories (chromatographic and coupled chromatographic-spectrometric procedures: GC-MS, HPLC-MS) which are very powerful tools for monitoring toxic analytes but, are expensive and time-consuming.116 Three aspects are typically associated with analytical sensing devices for environmental monitoring:116 (i) analyte specificity for determination of a specific compound, (ii) global toxicity associated with a class of pollutants as in the case of alarm systems and, (iii) group specificity, important for screening purposes. The following sections describe the use of advanced nanomaterials for integration into routine functional analytical devices for environmental monitoring, mainly chemical and biological sensors, divided according to their molecular recognition system. Table 2 summarizes nanomaterials that were used for this purpose and the corresponding analytical performance of these devices.
Table 2 Examples of selected nanomaterials applied for environmental monitoringa
Nanomaterials |
Analyte
|
Environmental matrix |
Sensitivity/Detection limit/Linear Range |
Quantification method/signal |
Ref. |
QDs – quantum dots, TiO2 Titanium dioxide, CNTs – Carbon nanotubes, TNT – trinitrotoluene, OPH – Organophosphate hydrolase, OPs – Organophosphorous pesticides. SWNTs – single walled carbon nanotubes, MWNTs - multiwalled carbon nanotubes, H2O2 – hydrogen peroxide, pM – picomolar, DNA – Deoxynucleic acid, BHI – Brain heart infusion, ELIME – Enzyme linked immunomagnetic electrochemical, NADH – Nicotinamide adenine dinucleotide (reduced), DL – detection limit, LR – Linear range.
|
Dendrimers
|
Copper(II)
|
Aqueous solutions |
— |
Binding energy |
100
|
Nanocomposite clay: allophane and smectite |
Copper(II)
|
Aqueous solutions |
— |
Mass difference |
184
|
17β-estradiol napthalene |
Pt, Au and Cu NPs and CNTs |
Explosive (TNT) |
Water, Soil |
1 ppb |
Cyclic voltammetry
|
|
Au NPs |
Paraoxon
|
Buffer
|
53.7 nA µM−1/12 µg L−1/24 to 1920 µg L−1 |
Current |
156
|
Ag NPs |
DNA
|
Aqueous |
--/0.5 pM/1 to 600 pM |
Stripping voltammetry
|
|
Quantum dots CdSe-ZnS QDs |
Paraoxon
|
Water
|
10 nM |
Fluorescence quenching |
92
|
CNTs/OPH |
Paraoxon Methylparathion
|
Water
|
250 nA µM−1/150 nM/up to 4 µM |
Cyclic voltammetry
|
27
|
6 nA µM−1/800 nM/up to 2 µM |
MWNTs |
H2O2 |
Phosphate buffer
|
2.3 nA µM−1/650 nM/5 to 500 µM |
Capillary electrophoresis/Amperometry |
29
|
Phenol
|
27 nA µM−1/210 nM/2.5 to 20 µM |
CNTs |
NO2 |
Air |
50 ppb/-/- |
Voltage |
185
|
CNTs |
H2O2 |
Aqueous |
0.56 nA µM−1/50 µM |
Cyclic voltammetry
|
32
|
β-NADH
|
2.03 nA µM−1/2000 nM |
Immunomagnetic beads |
E. Coli
|
BHI
|
-/-/101 to 106 cfu/ml |
Impedance spectroscopy
|
186
|
Superparamagnetic beads |
Salmonella typhimurium
|
buffer
|
8 × 103cells/ml |
ELIME
|
187
|
3.1.
Enzyme-based biosensors
Enzyme sensors are one of the most popular types of biosensors used for environmental pollutants monitoring. Nanostructured materials possess many of the required characteristics for the efficient immobilization of enzymes onto transducer surfaces, offering a good environment for retention of biocatalytic activity, and providing enhanced stability and efficiency. In addition, the reduced mass transfer limitation of substrates and inhibitors leads to improved sensitivities when compared to conventional macro-scale matrices.114,115 Examples include sensors based on cholinestereases (ChEs), acetylcholinesterase (AChE) and butyrylcholinesterase (BuChE) for pesticides, synthetic pyrethroids and heavy metals,117 tyrosinase for the detection of phenolic compounds,118 and OPH for the detection of pesticides.27
The most commonly used are the ChEs biosensors based on enzyme inhibition.119–121 This class of sensors is of particular interest in the area of global toxicity monitoring,116,122 with their signal reflecting the sum of all inhibiting species present in the sample. ChE enzymes are inhibited by several toxic chemicals such as organophosphate and carbamate pesticides, heavy metals, nicotine, nerve gases and anatoxin-a(s). In principle, all these compounds could be detected with these sensors. Depending on the extraction source, ChEs can have different substrate specificity and susceptibility to inhibitors. The most important research developments in electrochemical ChE biosensors have been discussed in detail in another work.119Table 3 summarizes various enzyme biosensors reported in literature for pesticides monitoring, fabricated using advanced nanomaterials. As an example, AChE sensors, with the enzyme immobilized in a CNT matrix25,26 exhibited enhanced electrocatalytic activity with more than one order of magnitude toward thiocholine, the product of the enzyme catalyzed reaction,119 and facilitated operation at low applied potential without the use of electronic mediators. With few exceptions, all electrochemical ChE sensors reported in literature were able to detect pesticides in the ppb range. The use of nanomaterials could lower the detection limit down to ppt levels. Willner's group reported photoelectrochemical sensing of AChE inhibitors using covalently linked AChE onto CdS NPs onto a Au electrode surface.123,124 The assembly of the CdS NPs/AChE onto the electrode surface and the detection principle is illustrated in Fig. 2A. Upon photo-irradiation, in the presence of the enzymatic substrate acetylcholine, a photocurrent is generated originating from the excitation of the semiconductor NPs. The photocurrent is proportional with the concentration of the enzyme reaction product, acetylthiocholine (Fig. 2B) and decreases in the presence of enzyme inhibitors. The same group developed a colorimetric test for AChE inhibitors based on the catalytic growth of AuNPs in the presence of acetylthiocholine.125 Du et al.126 reported a similar design for pesticide determination (i.e.malathion) with an electrochemical detection system. In another work, AChE labeled Au NPs were used to fabricate a localized surface plasmon resonance sensor which allowed detection of 0.234 ppb paraoxon.127
Table 3 Summary of enzyme biosensors based on advanced nanomaterialsa
Biosensor configuration |
Pollutant detected |
Linear inhibition response |
Detection limit |
Sensitivity |
Inhibition time/Response time (sec) |
Ref |
AChE–AuNPs-SiSG/GCE = AChE-gold nanoparticles-silica sol-gel/glassy carbon electrode; AChE-CHIT/MnTPP NPs/GCE = AChE-chitosan – manganese (111) meso-tetraphenylporphyrin nanoparticles/glassy carbon electrode; BuChE or OPH-IMERs = BuChE or OPH immobilized enzyme reactor – prepared using silica-encapsulation in situ through histidine-tag attachment of the silica/enzyme nanocomposites to a packed column; PPO/nanoCaCO3/GCE = polyphenol oxidase/calcium carbonate NPs/glassy carbon electrode; Au-PPy/Tyr/GCE – gold polypyrrole nanocomposite/Tyrosinase/glassy carbon electrode; Tyr–Aucoll–graphite–Teflon = Tyrosinase–gold NPs – composite graphite-teflon.
|
Biosensors based on AChE inhibition |
AChE-AuNPs-SiSG/GCE |
Monocrotophos |
0.001 to 1 µg/ml |
0.6 ng/ml at 10% inhibition |
— |
— |
188,189
|
2 to 15 µg/ml |
|
|
|
|
AChE-CHIT/MnTPP NPs/GCE |
Trichlorfon
|
1.0 nM–1.0 mM |
0.5 nM |
— |
— |
190
|
AChE-CHIT-AuNPs/GCE |
Malathion
|
0.001–0.1 µg mL−1 |
0.001 µg/ml |
— |
12 |
191
|
2–20 µg mL−1 |
|
|
|
|
Monocrotophos |
0.01–1 µg mL−1 |
|
|
|
|
1–5 µg mL−1 |
|
|
|
|
PDDA/AChE/PDDA/CNT/GCE |
Paraoxon
|
1 × 10−12–1 × 10−8 M |
4 × 10−13 M |
— |
6 |
29b
|
AuNPs/AChE |
Carbofuran
|
|
33 nM |
— |
— |
192
|
AChE-ZrO2/CHIT-GCE |
Phoxim
|
6.6 × 10−6 –4.4 × 10−4 M |
1.3 × 10−6 M |
— |
— |
193
|
Malathion Dimethoate
|
1.0 × 10−8 –5.9 × 10−7 M |
|
|
|
|
|
8.6 × 10−6 – 5.2 × 10−4 M |
|
|
|
|
|
Biosensors based on BuChE inhibition |
BuChE-IMERs |
Paraoxon
|
0–25 ppb |
5 ppb |
— |
— |
121
|
Demeton-S |
— |
— |
— |
— |
|
Malathion
|
— |
— |
— |
— |
|
|
OPH based biosensors |
OPH-IMPERs |
Paraoxon
|
0–2.5 ppm |
— |
— |
— |
121
|
Demeton-S |
0.01–0.2 µM |
— |
— |
— |
|
Malathion
|
0.01–0.2 µM |
— |
— |
— |
|
OPH-SiNPs SPR |
Paraoxon
|
20–100 µM |
20 µM |
— |
— |
194
|
|
Tyrosinase based biosensors |
PPO/nanoCaCO3/GCE |
Phenol
|
6 × 10−9–2 × 10−5 M |
0.44 nM |
474 mA/M |
<12 |
195
|
Au-PPy/Tyr/GCE |
Phenol
|
2.5 × 10−7 to 7 × 10−5 M |
3 × 10−8 M |
497.1 mA/M |
9 |
85
|
Tyr–Aucoll–graphite–Teflon |
Catechol
|
0.010–8.0 µM |
0.003 µM |
746 mA/M |
— |
196
|
Phenol
|
0.025–4.0 µM |
0.02 µM |
540 mA/M |
— |
|
3,4-dimethylphenol
|
0.05–2.0 µM |
0.011 µM |
563 mA/M |
— |
|
4-chloro-3-methylphenol
|
0.03–10.0 µM |
0.012 µM |
550 mA/M |
— |
|
4-chlorophenol
|
0.08–4 µM |
0.019 µM |
650 mA/M |
— |
|
4-chloro-2-methylphenol
|
10–275 µM |
3.3 µM |
9 mA/M |
— |
|
4-methylphenol
|
0.025–13 µM |
0.014 µM |
165 mA/M |
— |
|
3-methylphenol
|
0.025–12 µM |
0.012 µM |
184 mA/M |
— |
|
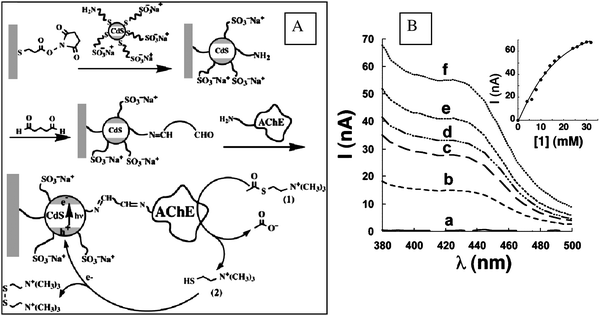 |
| Fig. 2
A: Schematic showing photoelectrochemical sensing principle of AChE substrate and inhibitors using a CdS NPs/AChE hybrid system. B: Photocurrent changes in the presence of different acetylthiocholine concentrations (from reference 90 with permission from the American Chemical Society). | |
In the case of OPH biosensors, the detection of pesticides is based on substrate hydrolysis in the presence of enzyme, followed by the direct measurement of the hydrolysis product using spectrophotometric or electrochemical procedures. Therefore, as opposed to ChEs sensors, the signal is directly proportional with the concentration of pesticide, which is the substrate of the enzymatic reaction.122 Detection limits of 0.15 µM and 0.8 µM for paraoxon and methyl parathion with sensitivities of 25 and 6 nA/µM were obtained using a OPH-CNTs-nafion composite deposited onto the surface of a glassy carbon electrode.27 An innovative method for direct detection of OPs neurotoxins using OPH was developed by Simonian et al.128 The method is based on the change in fluorescence of a competitive inhibitor of the OPH when this is displaced by the OPs substrate.128 In another system, paraoxon was detected using core-shell QDs with covalently linked OPH, via photoluminescence quenching.129 Arribas et al.29b developed an OPH–electrochemical sensor based on stimulated formation of cupric ferrocyanide NPs as a result of the accumulation of nitrophenol, the product of the enzymatic reaction, onto a carbon electrode transducer. However, OPH is not commercially available130 and thus the number of environmental applications is limited and insignificant as compared with biosensors based on ChE enzymes.
Tyrosinase biosensors represent another class of enzyme sensors relevant to environmental monitoring. These have been applied for the detection of pesticides such as 2,4-D, atrazine, and ziramviaenzyme inhibition131 and of phenolic compounds via direct enzyme oxidation.29,85 Sensors based on MWNTs dispersed in polyethylenimine (PEI) for electrochemical detection of phenolic compounds and herbicides were reported.29 A tyrosinase biosensor with the enzyme immobilized in Au polypyrrole nanocomposites allowed detection of as low as 8 × 10−8 M phenol, with a sensitivity of 460.1 A/M and a response time of less than 10 seconds.85 QDs-tyrosinase hybrids allowed determination of 100 nM phenolic compounds, via photoluminescence quenching QDs.95 In another work, core-shell (MgFe2O4–SiO2) magnetic NPs with covalently immobilized tyrosinase were attached to a carbon paste electrode surface via a magnet. The electrode was used to detect phenol in water samples.132 Such biosensors can have potential applications in monitoring of bisphenol A.133 Further developments in this direction could be obtained by incorporating enzyme-immobilized magnetic NPs in a flow injection analysis (FIA) system and retaining them near the detector using permanent magnets, as described by Kauffmann et al.134 for glucose oxidase.
In the future, development of more reliable immobilization protocols, and engineering of more sensitive enzymes135,117 could allow enzyme biosensors to be competitive for field environmental analysis and facilitate development of small, portable instruments for rapid toxicity testing and multianalyte screening.118
3.2. Immunosensors
Immunoassay techniques based on the ability of antibodies (Ab) to recognize and bind to a specific antigen (Ag)136,137 have gained considerable interest for the detection of environmental contaminants such as dioxins, triazine herbicides, explosives, pesticides, polychlorinated biphenyls and surfactants.138,139 These can use either a direct or indirect detection method, via an enzyme or a fluorescence label and the transduction mode can be electrochemical, quartz crystal piezoelectric and optical. As a result of the highly specific Ag-Ab recognition, immunosensors are characterized by high selectivity and sensitivity and can be adapted to work remotely, on-line or on-site.139 However, the limited number of compounds that can be determined and the cross-reactivity problems restrict their practical applicability in complex environmental samples.138,139 Comprehensive reviews of different types of immunosensors used for pesticides determination in natural waters have been published by Mallat and Barceló,140 Jiang et al.,141 Sadik and Van Emon142 and Kurosawa et al.143 Surprisingly, there are relatively few reports on immunosensors based on nanomaterials for environmental monitoring.7,144 Typically, in an immunosensor design these can be used as supporting materials for Ab immobilization and as labels for signal enhancement.7
Magnetic Fe2O3 NPs functionalized with specific antibodies (Ab) were used for the design of immunomagnetic electrochemical sensors through immobilization of the Ab-NPs assembly on the surface of an electrochemical transducer.145,48 Typically, the functionalized immunomagnetic NPs are attached to the surface of the electrode through a permanent magnet. The utilization of Ab-coated magnetic particles is efficient in overcoming the need of regeneration of the sensing surface and makes possible integration into automatic systems, which is hard to achieve otherwise due to the difficulties in renewing the sensing surface. The immunocomplex is usually quantified through the use of enzyme labels, with the electrochemical detection of the enzyme reaction product after the complex is exposed to the enzymatic substrate, or through a fluorescent label followed by fluorescence detection. Different environmental contaminants were detected in this way, such as: polychlorinated biphenyls (PCBs),146 2,4 D herbicide, atrazine147–151pesticides and bacterial pathogens.152,153 For example, such an approach allowed detection of Arochlor 1248 PCB with a detection limit of 0.4 ng/ml using screen-printed three electrode strips. Zacco et al.147 developed a sensitive electrochemical magnetoimmunosensor for atrazine using anti-atrazine-specific antibody covalently coupled to activated magnetic beads and reported a detection limit of 0.027 nmol L−1. Other immunosensors with renewable surface were proposed for the detection of pathogens such as Salmonella Typhimurium.152,153
Another interesting application of immunomagnetic iron oxide NPs was for selectively concentrating traces of pathogenic bacteria (Staphyloccocus saprophyticus and Staphyloccocus aureus) via IgGs attached onto the particles surface and then probing them using matrix assisted laser desorption/ionization mass spectrometry.154 Detection limits of as low as 3 × 105 cfu (colony-forming units)/ml were achieved in aqueous solutions. It was found that these IgG-NPs conjugates can bind selectively to the cell membrane. Fig. 3A shows TEM images of S. saprophyticus in the presence of bare and IgG modified Au NPs showing the binding of IgG–Au–NPs to the entire cell surface.154 Surprisingly, the bare AuNPs do not bind to the cell surface in the absence of the Ab, demonstrating that binding occurs exclusively through affinity interactions. Fig. 3B illustrates an example of a synthetic procedure used to attach IgG onto Fe3O4 magnetic NPs.154 General principles and other specific examples of monodispersed bio-functional magnetic NPs for protein separation and pathogen detection were discussed recently in an article by Guet al.155
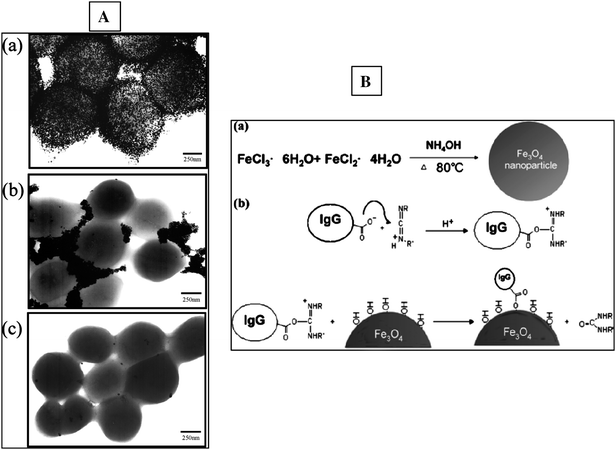 |
| Fig. 3 A: TEM images of S. Saprophyticus in the presence of IgG modified (a), bare (b) and BSA (c) Au–NPs. B: synthetic procedure used to immobilize IgG onto Fe3O4 magnetic NPs (from reference 154 with permission from the American Chemical Society). | |
Other nanomaterials such as AuNPs, QD and polymeric NPs were used in various immunoassay designs. An electrochemical immunosensor fabricated by casting Ab labeled Au NPs on a glassy carbon electrode allowed detection of as low as 12 ppb paraoxon with a linear range of 24–1920 ppb.156 Park et al.157 improved the sensitivity of a piezoelectric immunosensor for bisphenol A by approximately eight times by using polymeric NPs of 2-methacryloyloxyethyl phosphorylcholine and polysterene coated with anti-Bisphenol Ab. Fluorescently (TNB-Alexa Fluor 555) labeled Luminex microbeads allowed rapid and sensitive detection of 2,4,6-trinitrotoluene (TNT) using a competitive immunoassay.156 When TNT is present in solution, Alexa Fluor-TNB is displaced and the microspheres become less fluorescent, generating a decrease in the fluorescence signal. Similar competitive procedures could be used to immobilize different types of Ab onto a variety of nanoassemblies as illustrated by the general schematic in Fig. 4. An optical indium tin oxide (ITO) waveguide with fluorescent europium(III) chelate NPs labels (Seradyn) used as a platform in a competitive atrazine immunoassay provided detection limits comparable with the traditional ELISA.158 QDs coupled to antibody fragments were used to develop solution phase, nanoscale sensing assemblies for detecting explosives (e.g.TNT) in aqueous environments using a fluorescence energy transfer (FRET) technique.96
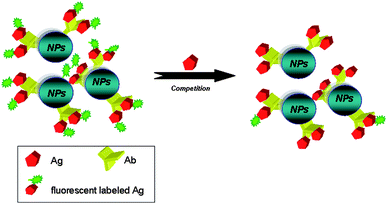 |
| Fig. 4 Example of a competitive fluorescence immunoassay based on a fluorescently labeled Ab-functionalized NP platform. | |
3.3.
DNA–based assays
Several nanoscale materials were used to fabricate DNA assays and quantify hybridization events in both solution phase and attached to transducer surfaces. DNA sensors with single-stranded (ss)oligodeoxynucleotides immobilized on electrode surfaces via magnetic NPs were fabricated and described in several papers.159–165 A hybrid probe-target duplex is formed when the sequence of target DNA matches that of the oligodeoxynucleotide probe. Pathogens such as Salmonella spp were detected using a genomagnetic assay with electrochemical detection, enzymatic labelling and DNA amplification with magnetic primers.165 The electrode consisted of a graphite epoxy composite and streptavidin modified magnetic NPs with immobilized DNA.
DNA functionalized core-shell magnetic/luminescent NPs probes were used for quantifying bacteria biodegrading methyl tertiary-butyl ether using fluorescence spectroscopy. The general strategy is presented in Fig. 5. A hybridized DNA-NPs complex was formed in the presence of a DNA labelled with a fluorescent dye (fluorescein isothiocyanate). Then, the hybridization complex was separated from the non-hybridized dye with a magnet and finally, the hybridization was quantified by measuring the fluorescence of the fluorescein dye. In another work, a three layer magnetic NPs structure with a gold surface, silica core and a magnetic inner layer was functionalized with DNA.164 Upon hybridization with complementary oligonucleotides, the DNA-aggregates act in the same way as Au NPs.
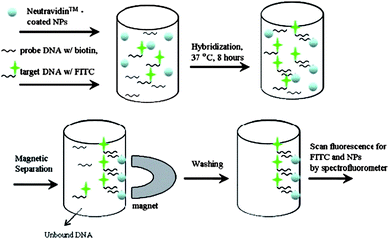 |
| Fig. 5 Strategy for quantifying DNA hybridization reactions using core-shells Eu:Gd2O3/Fe3O4 NPs with fluorescence detection (from reference 197 with permission from Elsevier). | |
As an alternative to enzymatic or fluorescent labels, Au–NPs functionalized with oligonucleotides were used as probes for nucleic acid detection and screening of DNA-binding molecules, providing opportunities for obtaining enhanced sensitivity and selectivity in many bioanalytical assays. Mirkin's group166–169 developed sequence-selective DNA detection that uses two AuNPs probes with one or two covalently bound oligonucleotides, complementary to the target of interest. Mixing of the two DNA-NPs, in the presence of DNA binding molecules, resulted in the formation of a NPs network. The process is accompanied by a color change, from red to blue, due to the formation of NPs aggregates. Depending on the binding affinity strength, the process is reversible with the temperature (Fig. 6).169 A colorimetric assay for drug screening was developed based on this principle. Such strategies could be applied to the detection of various environmental targets. In other works, AuNPs were used as bio-tags for the electrochemical transduction of hybridization events. The signal can be amplified by using a so-called silver enhancement process, via a chemical or electrochemical Ag deposition. The process consists in the reduction of Ag ions to atoms on AuNPs surfaces, in the presence of a reducing agent. For example, Lee et al.170 utilized an Ag enhancement procedure to develop an electrochemical method to quantify DNA hybridization on ITO electrodes. The schematic of the principle and the fabrication steps of the hybridization assay are illustrated in Fig. 7.170
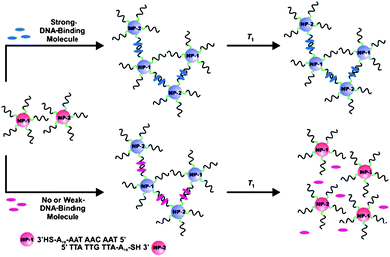 |
| Fig. 6 DNA-AuNPs assemblies for colorimetric screening DNA-binding molecules (from reference 169 with permission from Wiley-VHH Verlag GmbH&Co KGaA, Weinheim). | |
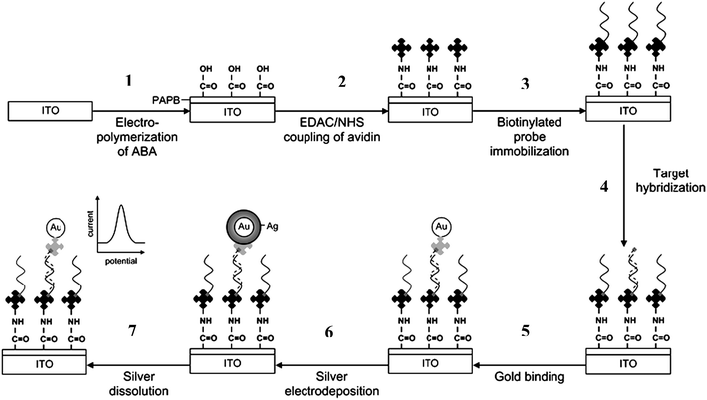 |
| Fig. 7 Schematic of the DNA hybridization assay protocol with the silver electrodeposition of the 10 nm gold–DNA conjugate tag. The protocol involves seven major steps: (1) electropolymerization of 2-aminobenzoic acid (ABA); (2) covalent attachment of avidin to the carboxyl group of the electropolymerized ABA viaEDAC/NHS coupling chemistry; (3) immobilization of biotinylated capture probevia avidin–biotin interaction; (4) hybridization between the capture probe and biotinylated target; (5) specific attachment of the 5 nm gold–streptavidin label; (6) silver enhancement of the hybrid-bound gold nanoparticle with the electrodeposition method; and (7) measurement of the deposited silver with linear sweep voltammetry. from reference 170. With permission from the Royal Society of Chemistry. | |
Furthermore, as an alternative to the more established immunological procedures, single stranded DNA or RNA ligands (aptamers) were recently used as biomolecular recognition elements. These can be selected to bind different environmental targets with high recognition ability. As an example, a DNAzyme (catalytic nucleic acid) possessing a high specificity for Pb2+ (the 17E DNAzyme) was used to fabricate a label free sensor for metal ion detection using AuNPs probes. The method is based on the fact that unfolded ssDNA could be absorbed on citrate protected AuNPs while the dsDNA do not (Fig. 8A).171 In the absence of Pb2+, AuNPs form visible aggregates after addition of NaCl. However, in the presence of Pb2+, the particles do not aggregate. This observation was confirmed with TEM and UV-VIS spectroscopy (Fig. 8B). The 17E DNAzyme based sensor allowed detection of Pb2+ within 20 minutes, with a detection limit of 500 nM; the calibration curve is presented in Fig. 8C. Aptamers based assays and their practical applications were summarized in several review papers.172–175 Though very promising, their environmental applications are still very little explored.
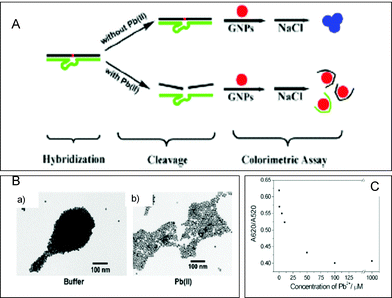 |
| Fig. 8
A: Strategy for Pb2+ detection using GNP colorimetry. 17E DNAzyme was first hybridized with its substrate to form the duplex. In the presence of Pb2+, the substrate strand was cleaved, resulting in the release of fragments which could be adsorbed on the GNP probes and thus could stabilize the GNP probes in the presence of a given high concentration of salt (the red solution appears). In the absence of Pb2+, however, the remaining duplex could not be adsorbed on the GNP probes and thus could not stabilize the GNP probes in the presence of a given high concentration of salt (the blue solution appears due to the aggregation of GNP probes induced by the salt). B: TEM images of the 13 nm GNPs mixed with 17E–17DS duplex solutions in the absence (A) and presence of 50 µM Pb2+ (B) after addition of 39 mM NaCl. C: calibration curve. (From reference 171 with permission from the IOPP Publishing.) | |
3.4. Nano-based gas sensing
Sensors for the detection of gases such as CO2, CO, SO2, O2, O3, H2, Ar, N2, NH3, are important in many different fields, such as, industrial emission control, household security, vehicle emission control, and environmental monitoring. Organic vapors such as methanol, ethanol, and phenol have also been considered.5 Nanostructured materials contribute a great deal to the function and optimization of these devices. The main types of materials used to fabricate gas sensors are nanosized metal oxides. Their semiconducting property allows for the electrical conductivity of the material to change when the composition of the atmosphere changes. Devices based on these materials are very sensitive due to their high area/volume ratio and function based on the same principles as standard metal oxide sensors. In the device, nanostructures are dispersed on the surface of a substrate to allow for increased adsorption of gases. The nanostructured surface changes its electronic properties when in contact with the analyte.6
Twenty three metal oxide semiconductors nanoparticles (e.g. Fe2O3, Cr2O3, Mn2O3, NiO, CuO, CdO, SnO2, ZnO, etc.) were used to fabricate a sensor for monitoring nitrous oxide (N2O). Upon contact with N2O all metal oxides indicated the formation of electron accepting adsorbates onto their surface. Among them, SnO2 modified with basic oxide promoters (e.g.SrO, CaO) showed superior sensing properties for N2O.176 The mechanism involved decomposition of N2O into N2 and O2, followed by deposition of the intermediates (adsorbates, ad) onto the porous oxide surface. All sensors showed increased electrical resistance upon exposure.176 The following reactions were proposed to illustrate this mechanism:
In another work, ZnO nanostructureed films obtained by vapor phase deposition allowed selective detection of CO and NH3 when integrated into a conductometric gas sensor.177 A review on this topic was published by Gouma et al.19Zirconia (ZrO2) NPs were used as selective sorbents for the design of an electrochemical sensor for organophosphate pesticides detection.178 The sensor is based on the affinity of ZrO2 for the phosphoric group of nitroaromatic organophosphorus (OPs) compounds. To fabricate the sensor, ZrO2 was electrodeposited onto gold electrodes by cyclic voltammetry and then exposed to OPs to allow binding. The amount of bound OPs was quantified by square-wave voltammetry. A detection limit of 3 ng/ml and a linear range of 5–100 ng/ml for methyl parathion were achieved after two min adsorption. In another work, MgFe2O4 NPs were used as materials in sensors for ethanol, methane (CH4), liquid petroleum gas (LPG) and hydrogen sulfide (H2S).145
5. Conclusions
Advanced nanomaterials (nanoparticles, nanofibers, nanoporous sorbents, etc.) have made a significant impact in the environmental field. Their increased surface area-to-volume ratio, coupled with the ability to tune their surface properties through molecular modification make them ideal for many environmental monitoring and remediation applications. This review provided an overview of the various nanoparticles and nanostructures used for this purpose, their integration into functional analytical devices, applications as electrode materials and gas sensing nanoprobes, in biosensors and as capture probes in immunomagnetic separations. Examples of nanomaterials-based chemical and biological sensors with applications in environmental monitoring were reviewed.
In the future, progress in nanotechnology and microelectronics could facilitate development of powerful environmental monitoring systems for rapid toxicity screening, multianalyte testing and possibly as detectors in chromatographic systems. Such devices are likely to become more compact, robust, smaller and adaptable for in field and continuous monitoring of a large diversity of environmental pollutants such as pesticides, environmental estrogens and bacterial pathogens. However, while several nanomaterial based systems have been reported in literature, their implementation into routine functional devices still remains a challenge. On the other side, environmental impact and health effects of synthetic nanomaterials are largely unknown and their use has recently become of particular concern.198
Acknowledgements
This work was supported by grants NSF-0727861, NSF-0804506, USDA-PUF 8000019748-01 and USAR W911WF-05-1-0339.
References
- X. Luo, A. Morrin, A. J. Killard and M. R. Smyth, Electroanal, 2006, 18(4), 319–326 CrossRef CAS.
- J. Pillay and K. I. Ozoemena, Electrochem. Comm., 2007, 9, 1816–1823 CrossRef CAS.
- A. Vaseashta, M. Vaclavikova, S. Vaseashta, G. Gallios, P. Roy and O. Pummakarnchana, Sci Tech. Adv. Mat., 2007, 8, 47–59 Search PubMed.
- G. Jimenez-Cadena, J. Riu and F. X. Rius, Analyst, 2007, 132, 1083–1099 RSC.
- R. Khan and M. Dhayal, Electrochem. Comm., 2008, 10(3), 492–495 CrossRef CAS.
- Y.-F. Li, Z.-M. Liu, Y.-Y. Liu, Y.-H. Yang, G.-L. Shen and R. Q. Yu, Anal. Biochem., 2006, 349, 33–40 CrossRef CAS.
- G. Liu and Y. Lin, Talanta, 2007, 74, 308–317 CrossRef CAS.
- M. Pumera, S. Sánchez, I. Ichinose and J. Tang, Sens. Actuat B, 2007, 123, 1195–1205 CrossRef.
- A. Salimi, R. Hallaj, S. Soltanian and H. Mamkhezri, Anal. Chim. Acta, 2007, 594, 24–31 CrossRef CAS.
- J. Hrbac, V. Halouzka, R. Zboril, K. Papadopoulos and T. Triantis, Electroanal., 2007, 19(17), 1850–1854 CrossRef CAS.
- K. Schachl, H. Alemu, K. Kalcher, J. Jezkova, I. Svancara and K. Vytras, Analyst, 1997, 122, 985–989 RSC.
- S. Yao, J. Xu, Y. Wang, X. Chen, Y. Xu and S. Hu, Anal. Chim. Acta, 2006, 557, 78–84 CrossRef CAS.
- M. Hermanek, R. Zboril, I. Medrik, J. Pechousek and C. Gregor, J. Am. Chem. Soc., 2007, 129, 10929–10936 CrossRef CAS.
- J. Wang, M. Scampicchio, R. Laocharoensuk, F. Valentini, O. Gonzalez-Garcia and J. Burdick, J. Am. Chem. Soc., 2006, 128(4), 4562–4563 CrossRef CAS.
- J. Wang, M. Musameh and R. Laocharoensuk, Electrochem. Commun., 2005, 7, 652–656 CrossRef CAS.
- E. Katz and I. Willner, J. Am. Chem. Soc, 2004, 124, 10290–10291.
- E. Katz, I. Willner and J. Wang, Electroanal., 2004, 16, 19–44 CrossRef CAS.
- E. V. Milson, J. Novak, M. Oyama and F. Marken, Electrochem. Commun., 2007, 9, 436–442 CrossRef.
- P. I. Gouma, A. K. Prasad and K. K. Iyer, Nanotechnol., 2006, 17, S48–S53 CrossRef CAS.
- S. Lijima, Nature, 1991, 354, 56–58 CrossRef CAS.
- P. M. Ajayan, Chem. Rev., 1999, 99, 1787–1799 CrossRef CAS.
- R. H. Baughman, A. A. Zakhidov and W. A. de Heer, Science, 2002, 297, 787–792 CrossRef CAS.
-
D. Andreescu, S. Andreescu, O. A. Sadik, New Materials for Biosensors, Biochips and Molecular Bioelectronics in “Biosensors and Modern Biospecific Analytical Techniques”, (Ed. L. Gorton) Vol. XLIV, “Comprehensive Analytical Chemistry” (Ser. Ed. D. Barcelo), Elsevier, Amsterdam, (ISBN 0-444-50715-9), March 2005 Search PubMed.
- S. Sotiropoulou, V. Gavalas, V. Vamvakaki and N. A. Chaniotakis, Biosens. Bioelectron., 2003, 18, 211–215 CrossRef.
- K. A. Joshi, J. Tang, R. Haddon, J. Wang, W. Chen and A. Mulchaldani, Electroanal., 2005, 17, 54–58 CrossRef CAS.
- Y. H. Lin, F. Lu and J. Wang, Electroanal., 2004, 16, 145–149 CrossRef CAS.
- R. P Deo, J. Wang, I. Block, A. Mulchanhani, K. A Joshi, M. Trojanoweiz, F. Scholz, W. Chen and Y. Lin, Anal. Chim Acta., 2005, 530, 185–189 CrossRef CAS.
- G. A. Rivas, M. D. Rubianes, M. D. Rodriguez, N. F. Ferreyra, G. L. Lique, M. L. Pendano, M. A. Miscoria and C. Parrado, Talanta, 2007, 74, 291–307 CrossRef CAS.
-
(a) A. S Arribas, E. Bemermejo, M. Chicharo, A. Zapardiel, G. L. luque, N. F. Ferreyra and G. A Rivas, Anal Chim. Acta., 2007, 596, 183–194 CrossRef;
(b) A. S. Arribas, T. Vázquez, J. Wang, A. Mulchandani and W. Chen, Electrochem. Commun., 2005, 7, 1371–1374 CrossRef CAS.
- P. J. Britto, K. S. V. Santhanam and P. M. Ajayan, Bioelectrochem. Bioenergetics, 1996, 41, 121–125 CrossRef CAS.
- R. Antiochia, I. Lavagnini, F. Magno, F. Valentini and G. Palleschi, Electroanal., 2004, 16, 1451–1458 CrossRef CAS.
- F. Valentini, A. Amine, S. Orlanducci, M. L. Terranova and G. Palleschi, Anal. Chem., 2003, 75, 5413–5421 CrossRef CAS.
- H. Luo, Z. Shi, Z. Gu and Q. Z. Zhuang, Anal. Chem., 2001, 73, 915–920 CrossRef CAS.
- Y. Sha, L. Qian, Y. Ma, H. Bai and X. Yang, Talanta, 2006, 70(3), 556–560 CrossRef CAS.
-
S. Andreescu, J. Njagi, C. Ispas, Nanostructured Materials for Enzyme Immobilization and Biosensors, in “The New Frontiers of organic and composite nanotechnology” Ed. V. Erokhin, M. K. Ram, O. Yavuz, Elsevier (ISBN 978-0-0804-5052-0), 2008, 355–394 Search PubMed.
- S. Hrapovic, E. Majid, K. Male and J. H. T. Luong, Anal Chem., 2006, 78, 5504–5512 CrossRef CAS.
- M. Musameh and J. Wang, Langmuir, 2005, 21, 8565–8568 CrossRef CAS.
- S. Guo and E. Wang, Anal. Chim. Acta., 2007, 598, 181–192 CrossRef CAS.
- N. N. Kariuki, J. Luo, S. A. Hassan, I.-Im. S. Lim, L. Wang and C. J. Zhong, Chem. Mater., 2006, 18, 123–132 CrossRef CAS.
- S. Shrivastava, T. Bera, A. Roy, G. Sighn, P. Ramachandrarao and D. Dash, Nanotechnol., 2007, 18, 1–9.
- C. Baker, A. Pradhan, L. Pakstis, D. J. Pochan and S. I. Shah, J. nanosci. Techno., 2005, 5, 244–249 Search PubMed.
- I. Sondi and B. Salopek-Sondi, J. Colloid Interface Sci., 2004, 275, 1770–1782.
- J. R. Morones, J. L. Elechiguerra, A. Camacho, K. Holt, J. b. Kouri, J. P. Ramirei and M. Y. Yacaman, Nanotechnol., 2005, 16, 2346–2353 CrossRef CAS.
- H. Cai, Y. Xu, N. Zhu, P. He and Y. Fang, Analyst, 2002, 127, 803–808 RSC.
- G. K. Darbha, Ray A. Anandhi and P. C. Ray, ACS Nano, 2007, 1(3), 208–214 CrossRef CAS.
- P. He and D. Y. Zhang, Environ. Sci. Technol., 2005, 39, 3314–3320 CrossRef CAS.
- J. Theron, J. A. Walker and T. E. Cloete, Critical Reviews in Microbiology, 2008, 34(1), 43–69 CrossRef CAS.
- M. Corti, A. Lascialfari, E. Micotti, A. Castellano, M. Donativi, A. Quarta, P. D. Cozzoli, L. Manna, T. Pellegrino and C. Sangregorio, J. Magn. Magn. Mater., 2008, 320(14), E320–E323 CrossRef CAS.
- Y. W. Jun, Y. M. Huh, J. S. Choi, H. T. Song, S. Kim, S. Yoon, K. S. Kim, J. S. Shin, J. S. Suh and J. Cheon, J. Am. Chem. Soc, 2005, 127(16), 5732–5733 CrossRef CAS.
- H. T. Song, J. S. Choi, Y. M. Huh, S. Kim, Y. W. Jun, J. S. Suh and J. Cheon, J. Am. Chem. Soc, 2005, 127(28), 9992–9993 CrossRef CAS.
- S. Hanessian, J. A. Grzyb, F. Cengelli and L. Juillerat-Jeanneret, Bioorg. Med. Chem., 2008, 16(6), 2921–2931 CrossRef CAS.
- T. Y. Liu, S. H. Hu, K. H. Liu, D. M. Liu and S. Y. Chen, J. Control. Release, 2008, 126(3), 228–236 CrossRef CAS.
- F. H. Chen, Q. Gao and J. Z. Ni, Nanotechnology, 2008, 19, 165103–165112 CrossRef.
- S. H. Wu, Y. S. Lin, Y. Hung, Y. H. Chou, Y. H. Hsu, C. Chang and C. Y. Mou, Chembiochem, 2008, 9, 53–57 CrossRef CAS.
- I. Safarik and M. Safarikova, Monatshefte fur Chemie, 2002, 133, 737–759 CrossRef CAS.
-
(a) Y. Yin and A. P. Alivisatos, Nature, 2005, 437, 664–670 CrossRef CAS;
(b) C. Burda, X. B. Chen, R. Narayanan, M. A and El-Sayed, Chem. Rev., 2005, 105(4), 1025–1102 CrossRef CAS.
- H. Y. Park, M. J. Schadt, L. Wang, I. I. S. Lim, P. N. Njoaki, S. H. Kim, M. J. Jang, J. Luo and C. J. Zhong, Langmuir, 2007, 23(17), 9050–9056 CrossRef CAS.
- I.-M. Hsing, Y. Xu and W. Zhao, Electroanal., 2007, 19(7–8), 755–768 CrossRef CAS.
- C. T. Yavuz, J. T. Mayo, W. W. Yu, A. Prakash, J. C. Falkner, S. Yean, L. Cong, H. J. Shipley, A. Kan, M. Tomson, D. Natelson and V. L. Colvin, Science, 2006, 314, 964–967 CrossRef.
- S. J. Kuhn, D. E. Hallahan and T. D. Giorgio, Annals of Biomedical Engineering, 2006, 34, 51–58 Search PubMed.
- J. Peral, X. Domenech and D. F. Ollis, J. Chem. Technol. Biotechnol., 1997, 70, 117–140 CrossRef CAS.
- C. Zengxiong, Z. Wanpeng, Y. Shaoxia and W. Jianbing, Chinese J. Catal., 2006, 27, 1073–1079 Search PubMed.
- D. S. Koktysh, X. Liang, B.-G. Yun, I. Pastoriza-Santos, R. L. Matts, M. Giersig, C. Serra-Rodrigues, L. M. Liz-Marzan and N. A. Kotov, Adv. Funct. Mater., 2002, 12, 255–265 CrossRef CAS.
- U. Diebold, Surf. Sci. Rep, 2003, 48, 53–229 CrossRef CAS.
- M. Niederberger, G. Garnweitner, F. Krumeich, R. Nesper, H. Colfen and M. Antonietti, Chem. Mater., 2004, 16, 1202–1208 CrossRef CAS.
- Y. Xie, L. Zhou and H. Huang, Biosens. Bioelectron., 2007, 22, 2812–2818 CrossRef CAS.
- E. Santos, N. K. Kuromoto and G. A. Soares, Mater. Chem. Phys., 2007, 102, 92–97 CrossRef CAS.
- Z. Zhang, C.-C. Wang, R. Zakaria and J. Y. Ying, J. Phys. Chem. B, 1998, 102, 10871–10878 CrossRef CAS.
- L. Francioso, D. S. Presicce, M. Epifani, P. Siciliano and A. Ficarella, Sens. Actuat. B, 2005, 107, 563–571 CrossRef.
- Y. J. Choi, Z. Seeley, A. Bandyopadhyay, S. Bose and S. A. Akbar, Sens. Actuat. B, 2007, 124, 111–117 CrossRef.
- J. C. Yu, W. Ho, J. Yu, H. Yip, P. K. Wong and J. Zhao, Environmental Sci. Technol, 2005, 39, 1175–1179 CrossRef CAS.
- S. V. Manorama, N. Izu, W. Shin, I. Matsubara and N. Murayama, Sens. Actuat. B, 2003, 89, 299–304 CrossRef.
- S. S. Lin, C. L. Chen, D. J. Chang and C. C. Chen, Water Research, 2002, 36, 3009–3014 CrossRef CAS.
- S. Hamoudi, F. Larachi, G. Cerrella and M. Cassanello, Ind. Eng. Chem. Res., 1998, 37, 3561–3566 CrossRef CAS.
- P. Kamat, R. Huehn and R. Nicoleascu, J. phys. Chem. B, 2002, 106, 788–794 CrossRef CAS.
- C. Terashima, T. N. Rao, B. V. Sarada, N. Spataru and A. Fujishima, J. Electroanal. Chem., 2003, 544, 65–74 CrossRef.
- S. B. Hall, E. A. Khudaish and A. L. Hart, Electrochim. Acta, 1999, 44(25), 4573–4582 CrossRef CAS.
- M. Chikae, K. Idegami, K. Kerman, N. Nagatani, M. Ishikawa, Y. Takamura and E. Tamiya, Electrochem. Commun, 2006, 8(8), 1375–1380 CrossRef CAS.
- C. Ispas, J. Njagi, M. Cates and S. Andreescu, J. Electrochem, Soc., 2008, 155(8), F169–F176 CrossRef CAS.
- B. Sljukic, C. E. Banks and R. G. Compton, Nano Lett., 2006, 6(7), 1556–1558 CrossRef CAS.
- B. Šljukić and R. G. Compton, Electroanalysis, 2007, 19(12), 1275–1280 CrossRef CAS.
- M. Albareda-Sirvent, A. Merkoci and S. Alegret, Sens. Actuators. B, 2000, 69(1–2), 153–163 CrossRef.
- J. Jin, R. Li, H. Wang, H. Chen, K. Liang and J. Ma, Chem. Commun., 2007, 4, 386–388 Search PubMed.
- R. Allabashi, M. Arkas, G. Hormann and D. Tsiourvas, Water Research, 2007, 41, 476–486 CrossRef CAS.
- J. Njagi and S. Andreescu, Biosens. Bioelectron., 2007, 23(2), 168–175 CrossRef CAS.
- M. A. Camblor, A. Corna and S. Valencia, J. Mater. Chem., 1998, 8, 2137–2145 RSC.
- A. Tavoralo, P. Tavolaro and E. Drioli, Colloid Surf. B Biointerfaces, 2007, 55, 67–76 CrossRef.
- W. Song, V. H. Grassian and S. C. Larsen, Environ. Scie. Technol., 2005, 39, 1214–1220 Search PubMed.
- H. Kotte, B. Grundig, K. D. Vorlop, B. Stehlitz and U. Stottmeister, Anal. Chem., 1995, 67, 65–70 CrossRef CAS.
- J. Wang and A. Walcarius, J. Electroanal. Chem., 1996, 407, 183–187 CrossRef CAS.
- R. C. Somers, M. G. Bawendi and D. G. Nocera, Chem. Soc. Rev., 2007, 36, 579–591 RSC.
- J. M. Costa-Fernandez, R. Pereiro and A. Sanz-Medel, Trends in Analytical Chemistry, 2006, 25, 207–218 CrossRef.
- C. A Constantine, K. M. Gattas-Asfura, S. V. Mello, G. Grespo, V. Rastogi, T. C. Cheng, J. J. DeFrank and R. M. Leblanc, J. Phys, Chem. B, 2003, 107, 13762–13764 CrossRef.
- X. Ji, J. Zheng, J. Xu, V. Rastogi, T. C. Cheng, J. J. DeFrank and R. M. Leblanc, J. Phys, Chem. B, 2005, 109, 3793–3799 CrossRef.
- J. Yuan, W. Guo and W. Wang, Anal. Chem, 2008, 80, 1141–1145 CrossRef CAS.
- E. R. Goldman, I. L. Meditnz, J. L. Whitley, A. Hayhurst, A. R Clapp, H. T. Uyenda, J. R. Deschamps, M. E. Lessman and H. Mattoussi, J. Am. Chem. Soc., 2005, 127, 6744–6751 CrossRef CAS.
- J. M. Abad, S. F. L. Mertens, M. Pita, V. F. Fernandez and D. J. Schiffrin, J. Am. Chem. Soc., 2005, 127, 5689–5694 CrossRef CAS.
- A.-M. Caminade, C. Padie, R. Laurent, A. Maraval and J.-P. Majoral, Sensors, 2006, 6, 901–914 CrossRef CAS.
- A. W. Bosman, H. M. Janssen and E. W. Meijer, Chem Rev., 1999, 99, 1665–1668 CrossRef CAS.
- M. S. Diallo, S. Christie, P. Swaminathan, J. H. Johnson Jr. and W. A. Goddard, Environ. Scie. Technol., 2005, 39, 1366–1377 Search PubMed.
- L. H. Cumbal and A. k. SenGupta, Ind. Eng. Chem. Res., 2005, 44, 600–605 CrossRef CAS.
- W. Chen, L. Duan and D. Zhou, Environ. Scie. Technol., 2007, 41, 8295–8300 Search PubMed.
- T. Masciangioli and W.-X. Zhang, Environ. Scie. Technol., 2003, 37, 102A–108A Search PubMed.
- Q. R. Long and R. T. Yang, J. Am. Chem. Soc., 2001, 123, 2058–2059 CrossRef CAS.
- S. Horikoshi, A. Tokunaga, N. Watanabe, H. Hidaka and N. Serpone, Journal of Photochemistry and Photobiology A: Chemistry, 2006, 177, 129–143 Search PubMed.
- D. Chatterjee and S. Dasgupta, Journal of Photochemistry and Photobiology C: Photochemistry Reviews, 2005, 6, 186–205 Search PubMed.
- S. Horikoshi, A. Tokunaga, N. Watanabe, H. Hidaka and N. Serpone, Journal of Photochemistry and Photobiology A: Chemistry, 2004, 162, 33–40 Search PubMed.
- Z. Liu, X. Zhang, S. Nishimoto, M. Jin, D. A. Tryk, T. Murakami and A. Fujishima, J. Phys. Chem. C, 2008, 112, 253–259 CrossRef CAS.
- V. Belgiorno, L. Rizzo, D. Fatta, C. D. Rocca, G. Lofrano, A. Nikolaou, V. Naddeo and S. Meric, Desalination, 2007, 215, 166–176 CrossRef CAS.
- G. Hanrahan, D. G. Patil and J. Wang, J. Environ. Monit., 2004, 6, 657–664 RSC.
- K. R. Rogers, Anal. Chim. Acta, 2006, 568, 222–231 CrossRef CAS.
- O. Domínguez Renedo, M. A. Alonso-Lomillo and M. J. Arcos Martínez, Talanta, 2007, 73, 202–219 CrossRef.
- E. Z. Ron, Curr. Opin. Biotechnol., 2007, 18, 252–256 CrossRef CAS.
- J. Kim, J. W. Grate and P. Wang, Chem. Eng. Sci., 2006, 61, 1017–1026 CrossRef CAS.
- J. Wang, Analyst, 2005, 130, 421–426 RSC.
- U. Wollenberger, B. Neumann, K. Riedel and F. K. Scheller, Fresenius J. Anal. Chem., 1994, 348, 563–566 CAS.
- G. Istamboulie, S. Andreescu, J.-L. Marty and T. Noguer, Biosens. Bioelectron., 2007, 23(4), 506–512 CrossRef.
- Z. Liu, Y. Liu, H. Yang, Y. Yang, G. Shen and R. Yu, Anal. Chim. Acta, 2005, 533, 3–9 CrossRef CAS.
- S. Andreescu and J.-L. Marty, Biomol. Eng., 2006, 23, 1–15 CrossRef CAS.
- S. Andreescu, L. Barthelmebs and J. L. Marty, Anal. Chim. Acta., 2002, 464(2), 171–180 CrossRef CAS.
- C. Bonnet, S. Andreescu and J.-L. Marty, Anal. Chim. Acta., 2003, 481, 209–211 CrossRef CAS.
- H. R. Luckarift, R. Greenwald, M. H. Bergin, J. C. Spain and G. R. Johnson, Biosens. Bioelectron., 2007, 23, 400–406 CrossRef CAS.
- V. Pardo-Yissar, E. Katz, J. Wasserman and I. Willner, J. Am. Chem. Soc., 2003, 125, 622–623 CrossRef CAS.
- M. Zayats, A. B. Kharitonov, S. P. Pogorelova, O. Lioubashevski, E. Katz and I. Willner, J. Am. Chem. Soc., 2003, 125, 16006–16014 CrossRef CAS.
- V. Pavlov, Y. Xiao and I. Willner, Nano Lett., 2005, 5(4), 649–653 CrossRef CAS.
- D. Du, J. Ding, J. Cai and A. Zhang, Sens. Actuators, B, 2007, 127, 317–322 CrossRef.
- T.-J. Lin, K.-T. Huang and C.-Y. Liu, Biosens. Bioelectron., 2006, 22, 513–518 CrossRef CAS.
- A. L. Simonian, T. A. Good, S.-S. Wang and J. R. Wild, Anal. Chim Acta, 2005, 534, 69–77 CrossRef CAS.
- X. S. Ji, J. Y. Zheng, J. M. Xu, V. K. Rastogi, T. C. Cheng, J. J. Defrank and R. M. Leblanc, J. PhysChem. B, 2005, 109(9), 3793–3799 CrossRef.
- G. Liu and Y. Lin, Anal. Chem., 2006, 78, 835–843 CrossRef CAS.
- G. Y. Kim, J. Shim, M. S. Kang and S. H. Moon, J. Environ. Monit., 2008, 10(5), 632–637 RSC.
- Z. Liu, Y. Liu, H. Yang, Y. Yang, G. Shen and R. Yu, Anal. Chim. Acta, 2005, 533, 3–9 CrossRef CAS.
- S. Andreescu and O. A. Sadik, Anal. Chem., 2004, 76, 552–260 CrossRef CAS.
- A. Nomura, S. S. O. Mehdi and J. M. Kauffmann, Anal. Chem., 2004, 76, 5498–5502 CrossRef CAS.
- P. R. B. O. Marques, G. S. Nunes, S. Andreescu and J.-L. Marty, Biosens. Bioelectron., 2004, 20, 824–831 CrossRef.
- O. A. Sadik, A. K. Wanekaya and S. Andreescu, J. Environ. Monit., 2004, 6, 413–522 RSC.
- S. Andreescu and O. A. Sadik, Pure Appl. Chem. (A review), 2004, 76(4), 861–878 Search PubMed.
- D. R. Shankaran, K. V. Gobi and N. Miura, Sens. Actuators B, 2007, 121, 158–177 CrossRef.
- M. Farré, L. Kantiani and D. Barceló, Trends Anal. Chem., 2007, 26(11), 1100–1112 CrossRef CAS.
- E. Mallat and D. Barceló, Trends Anal. Chem., 2001, 20(3), 124–132 CrossRef CAS.
- X. Jiang, D. Li, X. Xu, Y. Ying, Y. Li, Z. Ye and J. Wang, Biosens. Bioelectron., 2008, 23, 1577–1587 CrossRef CAS.
- O. A. Sadik and J. M. Van Emon, Biosens. Bioelectron., 1996, 11(8), 1–10 CrossRef CAS.
- S. Kurosawa, J.-W. Park, H. Aizawa, S.-I. Wakida, H. Tao and K. Ishihara, Biosens. Bioelectron., 2006, 22, 473–481 CrossRef CAS.
- S.-Q. Hua, J.-W. Xie, Q.-H. Xu, K.-T. Rong, G.-L. Shen and R.-Q. Yu, Talanta, 2003, 61, 769–777 CrossRef CAS.
- J. P. Li and H. D. Gao, Elecroanalysis, 2008, 20(8), 881–887 CrossRef CAS.
- S. Centi, S. Laschi, M. Franek and M. Mascini, Anal. Chim. Acta, 2005, 538(1–2), 205–212 CrossRef CAS.
- E. Zacco, M. I. Pividori and S. Alegret, Anal. Chem, 2006, 78, 1780–1788 CrossRef CAS.
- A. G. Ghering, C. G. Crawford, R. S. Mazenko, L. J. VanHouten and J. D. Brewster, J. Immunol. Methods, 1996, 195(1–2), 15–25 CrossRef.
- M. Dequaire, C. Degrand and B. Limoges, Anal. Chem., 1999, 71(13), 2571–2577 CrossRef CAS.
- F. G. Perez, M. Mascini, I. E. Tothil and A. P. F. Turner, Anal. Chem., 1998, 70(11), 2380–2386 CrossRef CAS.
- S. Helali, C. Martelet, A. Abdelghani, M. A. Maaref and N. Jafrezic-Renault, Electrochim. Acta, 2006, 51, 5182–5186 CrossRef CAS.
- M. Varshney and Y. B. Li, Biosens. Bioelectron., 2007, 22(11), 2408–2414 CrossRef CAS.
- X. L. Su and Y. B. Li, Biosens. Bioelectron., 2005, 21(6), 840–848 CrossRef CAS.
- K. C. Ho, P. J. Tsai, Y. S. Lin and Y. C. Chen, Anal. Chem., 2004, 76, 7162–7168 CrossRef CAS.
- H. Gu, K. Xu, C. Xu and B. Xu, Chem. Commun., 2006,(9), 941–949 RSC.
- S.-H. Hu, J.-W. Xei, Q.-H. Xu, K.-T. Rong, G.-L. Shen and R.-Q. Yu, Talanta, 2003, 61, 769–777 CrossRef CAS.
- J. W. Park, S. Kurosawa, H. Aizawa, Y. Goda, M. Takai and K. Ishihara, Analyst, 2006, 131, 155–162 RSC.
- C. M. Cummins, M. E. Koivunen, A. Stephanian, S. J. Gee, B. D. Hammock and I. M. Kennedy, Biosens. Bioelectron., 2006, 21, 1077–1085 CrossRef CAS.
- R. H. Liu, J. N. Yang, R. Lenigk, J. Bonanno and P. Grodzinski, Anal. Chem., 2004, 76(7), 1824–1831 CrossRef CAS.
- E. Palecek and M. Fojita, Talanta, 2007, 74(3), 276–290 CrossRef CAS.
- A. Erdem, F. Sayar, H. Karadeniz, G. Guven, M. Oszos and E. Piskin, Electroanalysis, 2007, 19(7–8), 798–804 CrossRef CAS.
- S. G. Grancharov, H. Zeng, S. H. Sun, S. X. Wang, S. O'Brien, C. B. Murray, J. R. Kirtley and G. A. Held, J. Physical Chem. B, 2005, 109(26), 13030–13035 Search PubMed.
- D. B. Robinson, H. H. J. Persson, H. Zeng, G. X. Li, N. Pourmand, S. H. Sun and S. X. Wang, Langmuir, 2005, 21(7), 3096–3103 CrossRef CAS.
- S. I. Stoeva, F. Huo, J. S. Lee and C. A. Mirkin, J. Am. Chem. Soc., 2005, 127, 15362–15363 CrossRef CAS.
- A. Lermo, S. Campoy, J. Barbe, S. Hernandez, S. Alegret and M. I. Pividori, Biosens. Bioelectron., 2007, 22, 2010–2017 CrossRef CAS.
- C. A. Mirkin, R. L. Letsinger, R. C. Mucic and J. J. Storhoff, Nature, 1996, 382, 607–609 CrossRef CAS.
- R. Elghanian, J. J. Storhoff, R. C. Mucic, R. L. Letsinger, C. and A. Mirkin, Science, 1997, 277, 1078–1081 CrossRef CAS.
- J. J. Storhoff, R. Elghanian, R. C. Mucic, C. A. Mirkin and R. L. Letsinger, J. Am. Chem. Soc., 1998, 120, 1959–1964 CrossRef CAS.
- M. S. Han, A. K. R. Lytton-Jean, B. K. Oh, J. Heo and C. A. Mirkin, Angew. Chemie, Int. Ed., 2006, 45, 1807–1810 CrossRef CAS.
- T. Ming-Hung Lee, H. Cai and I. H. Hsing, Analyst, 2005, 130, 364–369 RSC.
- H. M. Wei, B. Li, S. Dong and E. Wang, Nanotechnology, 2008, 19, 1–5.
- T. Endo, K. Kerman, N. Nagatani, Y. Takamura and E. Tamiya, Anal. Chem., 2005, 77(21), 6976–6984 CrossRef CAS.
- S. Tombelli, M. Minunni and M. Mascini, Biomol. Eng., 2007, 24, 191–200 CrossRef CAS.
- S. Song, L. Wang, J. Li, J. Zhao and C. Fan, Trends Analytical Chemistry, 2008, 27(2), 108–171 Search PubMed.
- N. Santos-Alvarez, M. J. Lobo-Castanon, A. J. Miranda-Ordieres and P. Tunon-Blanco, Trends Analytical Chemistry, 2008, 27(2), 437–446 Search PubMed.
- E. Kanazawa, G. Sakai, K. Shimanoe, Y. Kanmura, Y. Teraoka, N. Miura and N. Yamazoe, Sens. Actuat. B, 2001, 77, 72–77 CrossRef.
- C. Baratto, E. Comini, G. Faglia, G. Sberveglieri, M. Zha and A. Zappettini, Sens. Actuat. B., 2005, 109, 2–6 CrossRef.
- G. Liu and Y. Lin, Anal. Chem., 2005, 77(18), 5894–5901 CrossRef CAS.
- V. Vamvakaki, K. Tsagaraki and N. Chaniotakis, Anal. Chem., 2006, 78, 5538–5542 CrossRef CAS.
- D. B. Haddow, J. M. Kelly, P. F. James, R. D. Short, A. M. Scutt, R. Rawsterne and L. Kothari, J. Mater. Chem., 2000, 10, 2795–2801 RSC.
- S. E. Kim, J. H. Lim, S. C. Lee, S.-G. Nam and J. Choi, Electrochim. Acta, 2008, 53, 4846–4851 CrossRef CAS.
- A. S. Karakoti, N. A. Monteiro-Riviere, R. Aggarwal, J. P. Davis and R. J. Narayan, JOM, 2008, 60(3), 33–37 CrossRef CAS.
- E. Topoglidis, A. E. G. Cass, B. O'Regan and J. R. Durrant, J. Electroanal. Chem., 2001, 517, 20–27 CrossRef CAS.
- G. Yuan, Journal of environmental science and health, Part A Toxic/Hazardous substances and environmental engineering, 2005, 39(10), 2661–2670 Search PubMed.
- J.-H. Lee, J. Kim, H. W. Seo, J.-W. Song, E.-S. Lee, M. Won and C.-S. Han, Sens. and Actuat. B, 2008, 129, 628–631 Search PubMed.
- L. Yang and Y. Li, Journal of Microbiological Methods, 2006, 64, 9–16 CrossRef.
- A. G. Gehring, C. G. Crawford, R. S. Mazenko, L. J. Van Houten and J. D. Brewster, Journal of Immunological Methods, 1996, 195, 15–25 Search PubMed.
- D. Du, S. Chen, J. Cai and A. Zhang, Talanta, 2008, 74, 766–772 CrossRef CAS.
- D. Du, S. Chen, J. Cai and A. Zhang, Biosens. Bioelectron., 2007, 23, 130–134 CrossRef CAS.
- X. H. Li, Z. H. Xie, H. Min, Y. Z. Xian and L. T. Jin, Electroanal., 2007, 19(24), 2551–2557 CrossRef CAS.
- D. Du, J. W. Ding, J. Cai and A. D. Zhang, J. Electroanal. Chem., 2007, 605(1), 53–60 CrossRef CAS.
- O. Shulga and J. R. Kirchhoff, Electrochem. Commun., 2007, 9, 935–940 CrossRef CAS.
- Y. Yang, M. Guo, M. Yang, Z. Wang, G. Shen and R. Yu, Int. J. Environ. Anal. Chem., 2005, 85, 163–175 CrossRef CAS.
- H. R. Luckarift, S. Balasubramanian, S. Paliwal, G. R. Johnson and A. L. Simonian, Colloids Surf., B, 2007, 58(1), 23–33.
- D. Shan, M. Zhu, E. Han, H. Xue and S. Cosnier, Biosens. Bioelectron., 2007, 23, 648–654 CrossRef CAS.
- V. Carralero, M. L. Mena, A. Gonzalez-Cortés, P. Yáñez-Sedeño and J. M. Pingarrón, Biosens. Bioelectron., 2006, 22, 730–736 CrossRef CAS.
- A. Son, D. Dosev, M. Nichkova, I. M. Kennedy, K. M. Scow and K. R. Hristova, Anal. Biochem., 2007, 370, 186–194 CrossRef CAS.
- B. C. Englert, J. Environ. Monit, 2007, 11, 1154–1161 Search PubMed.
|
This journal is © The Royal Society of Chemistry 2009 |