Silica grafted imidazolium-based ionic liquids: efficient heterogeneous catalysts for chemical fixation of CO2 to a cyclic carbonate
Received
1st June 2009
, Accepted 13th August 2009
First published on 11th September 2009
Abstract
Imidazolium-based ionic liquids with varied alkyl chain lengths bearing different anions (Cl−, Br− and I−) were synthesized and immobilized onto the commercial silica surface (IMIS). These heterogeneous catalysts exhibited high catalytic activities and selectivities in the cycloaddition of carbon dioxide to allyl glycidyl ether (AGE). The immobilized ionic liquids with longer alkyl chain length and more nucleophilic anion showed higher activity. In particular, compared with pristine IMIS, zinc halide-combined IMIS (IMIS-Zn) with Cl− exhibited an increased activity, while the IMIS-Zn with Br− and I− showed lower activity than IMIS itself. In addition, the textures of silica supports (surface area and average pore size) have a great impact on the catalytic activity owing to the substance-confined diffusion effects. The recycling experiments showed that the resulting heterogeneous catalysts exhibited good reusability.
Broader context
CO2 is the most abundant waste gas produced by human activities and one of the greenhouse gases. On the other hand, CO2 provides a nontoxic, cheap, and highly functional carbon source. How to convert this carbon resource into useful products is a significant issue in the environmental field. To realize that, chemical fixation of CO2 with epoxides to form cyclic carbonates under mild conditions by using ionic liquid-based heterogeneous catalysts offers a promising alternative. In our current study, we have demonstrated an economic and efficient synthetic strategy to fabricate a series of heterogeneous catalysts by means of grafting ionic liquids onto the commercial silica surfaces. These heterogeneous catalysts exhibited high catalytic activities and selectivities in the coupling reaction of allyl glycidyl ether and CO2. The factors such as molecular structure of grafted ionic liquids (alkyl chain length and anions), reaction conditions (temperature and pressure) as well as the texture properties (pore size and surface area) of commercial silica supports on the efficiency of coupling reaction have been investigated in detail.
|
Introduction
Carbon dioxide (CO2) is the most abundant waste gas produced by human activities and one of the greenhouse gases. On the other hand, CO2 is nontoxic, cheap, and highly functional carbon source and so it is extensively explored as a substitute for phosgene, isocyanates or carbon monoxide.1–3 More interestingly, by the coupling reaction with epoxide, CO2 can be utilized to synthesize cyclic carbonates. Five- and six-membered cyclic carbonates are excellent aprotic polar solvents, electrolytes in secondary batteries and used extensively as intermediates in the production of fine chemicals (dialkyl carbonates, glycols, carbonates, etc.), engineering plastics and pharmaceuticals.4 To synthesize cyclic carbonate, a wide range of catalysts such as alkali metal salts,5,6 metal oxides,7–9 zeolites,10 organic bases,11,12 titanosilicates,13 transition metal complexes and ionic liquids14–16 have been employed.
Owing to its low viscosity and negligible vapor pressure, ionic liquids (ILs) have been widely used as environmentally benign solvents in various organic reactions and regarded as a new important precursor material for catalyst. The recent development in catalysis showed that ILs could be used to catalyze many reactions, and showed high performance and selectivity.17–19 In particular, the imidazolium-based ILs showed effective catalytic performance towards the chemical fixation of CO2 with epoxides. For instance, [C4mim]Cl, [C4mim]Br and [C4mim]PF6 have all proved to be suitable reaction media for CO2cycloaddition to epoxide with good selectivity and conversion.20,21
Recently, the IL-based heterogeneous catalysts have been developed to synthesize cyclic carbonate and they allowed the combination of the advantages of both homogeneous ILs and heterogeneous catalysts. Compared to pure Lewis acidic ILs, such heterogeneous catalysts show additional advantages such as the reduction of the amount of ionic liquids employed and easy separation, as well as recovery of catalyst from the reaction mixture which is desirable from economic, environmental and industrial view. To synthesize the immobilized IL heterogeneous catalysts, it is crucial to choose suitable supports and an immobilization approach which directly affect the supported amount and catalytic behavior of the supported catalysts. To date, a variety of supports such as amorphous silica,22 mesoporous silica23,24 and polymer25 have been chosen to support ionic liquid as a heterogeneous catalyst for fixing CO2 to cyclic carbonate. For instance, Xie et al.25 introduced imidazolium-based IL into a crosslinked polymer matrix by means of the co-polymerization method. The resulting heterogeneous catalyst exhibited good catalytic activity in the reaction of various epoxides with CO2. Udayakumar et al.23,24 prepared IL-immobilized MCM41 catalystsvia the co-condensation approach for the synthesis of cyclic carbonate. Although these pioneering works have demonstrated an effective protocol for fabricating heterogeneous catalysts for the fixation of CO2 to a cyclic carbonate, the corresponding synthetic procedures are tedious and high-cost, which is not convenient for the production in plant-scale.
Aiming to reduce the cost of catalyst preparation, simplify the preparation procedures, minimize the consumption of ionic liquids, facilitate the separation of catalyst from reaction system and improve the reusability of the catalyst, we synthesized a series of imidazolium-based heterogeneous catalysts by grafting ionic liquids onto commercial silica. The activity of the resulting catalysts towards the coupling reaction of CO2 with allyl glycidyl ether (AGE) has been examined in detail. Several factors which affect the catalytic activity (such as molecular structures of ILs, reaction temperature, CO2 pressure as well as types of commercial silica) have been investigated in this study.
Experimental
Materials
Imidazole (Aldrich), 1-bromobutane (Aldrich), 1-chlorobutane (Aldrich), 1-bromopropane (Aldrich), bromoethane (Aldrich), 1-iodobutane (Aldrich), (3-chloropropyl) triethoxysiliane (Sigma), toluene (Aldrich), acetonitrile (Dae Jung), zinc chloride (Sigma), dichloromethane (Dae Jung) and allyl glycidyl ether (Aldrich) were used as received. Three types of commercial silica gels [70–100 m2 g, 260–340 Å (S1); 500 m2 g, 60 Å (S2); 800 m2 g, 22 Å (S3)] were purified using hot piranha solution (H2O2 and H2SO4) before use.
Synthesis of ILs (IL1–6)
1-Methyl-3-(3-triethoxysilypropyl) imidazolium chloride (IL1) was synthesized according to the process shown in reaction (1) of Scheme 1. 1-Methylimidazole (10 mL) and (3-chloropropyl) triethoxysiliane (80 mL) were mixed in a dry 250 mL flask under nitrogen flow. The mixture was stirred at 70 °C for 48 h. The reaction mixture was cooled to room temperature. After this, the unreacted reactants were removed by extraction with diethyl ether followed by evaporating the residual ether under reduced pressure. Finally, IL1 was obtained in 95% yield. 1H-NMR (300 MHz, CDCl3): δ 0.58 (m, 2H), 1.18 (t, 9H), 1.98 (m, 2H), 3.79 (q, 6H), 4.09 (s, 3H), 4.30 (t, 2H), 7.32 (s, 1H), 7.62 (s, 1H), 10.58 ppm (s, 1H); 13C-NMR (75 MHz, CDCl3): δ 6.8, 18.0, 23.9, 36.1, 51.3, 58.0, 121.9, 124.0, 138.1 ppm.
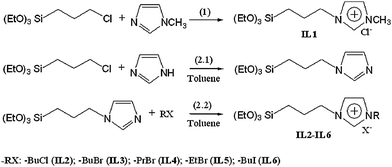 |
| Scheme 1 Synthesis of IL1 in reaction (1) and IL2–6 in reactions (2.1) and (2.2). | |
IL2–IL6 were synthesized according to procedures described in reactions (2.1) and (2.2) (Scheme 2). In a typical procedure, imidazole (3.4 g) was added in the flask containing 50 mL dry toluene, (3-chloropropyl)triethoxysilane (12 mL) was poured into the solution and the mixture was refluxed for 3 h under argon atmosphere. When the reaction was completed, 10 mL of 1-bromobutane was subsequently injected into the flask. The mixture was stirred continuously for another 24 h; IL2 can be obtained after removal of solvent. Following similar procedures, IL3–IL6 were synthesized by using 1-chlorobutane, 1-bromopropane, bromoethane and 1-iodobutane to replace 1-bromobutane, respectively. The NMR data of IL2–IL6 are as follows:
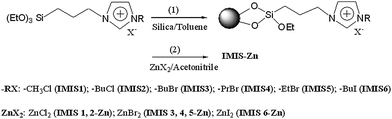 |
| Scheme 2 Synthesis of IMIS1–6 (1) and IMIS-Zn (2). | |
1-Butyl-3-(3-triethoxysilylpropyl) imidazolium chloride (IL2).
1H-NMR (300 MHz, CDCl3): δ 0.61 (m, 2H), 0.95 (t, 3H), 1.21 (t, 9H), 1.39 (m, 2H), 1.92 (m, 4H), 3.82 (q, 6H), 4.36 (m, 4H), 7.38 (s, 1H), 7.58 (s, 1H), 10.78 ppm (s, 1H); 13C-NMR (75 MHz, CDCl3): δ 6.8, 13.2, 18.1, 19.3, 24.1, 31.8, 49.4, 51.4, 58.6, 121.3, 122.4, 136.8 ppm.
1-Butyl-3-(3-triethoxysilylpropyl) imidazolium bromide (IL3).
1H-NMR (300 MHz, CDCl3): δ 0.68 (m, 2H), 0.95 (t, 3H), 1.24 (t, 9H), 1.39 (m, 2H), 1.92 (m, 4H), 3.73 (q, 6H), 4.35 (m, 4H), 7.27 (s, 1H), 7.41 (s, 1H), 10.45 ppm (s, 1H); 13C-NMR (75 MHz, CDCl3): δ 7.8, 13.3, 18.0, 19.2, 24.3, 31.9, 49.6, 51.5, 57.6, 118.9, 121.7, 134.6 ppm.
1-Propyl-3-(3-triethoxysilylpropyl) imidazolium bromide (IL4).
1H-NMR (300 MHz, CDCl3): δ 0.59 (m, 2H), 0.96 (t, 3H), 1.21 (t, 9H), 1.94 (m, 4H), 3.80 (q, 6H), 4.32 (m, 4H), 7.27 (s, 1H), 7.43 (s, 1H), 10.18 ppm (s, 1H); 13C-NMR (75 MHz, CDCl3): δ 6.8, 10.6, 18.2, 19.1, 23.4, 51.0, 51.3, 58.4, 121.3, 122.4, 134.5 ppm.
1-Ethyl-3-(3-triethoxysilylpropyl) imidazolium bromide (IL5).
1H-NMR (300 MHz, DMSO): δ 0.50 (m, 2H), 1.03 (t, 9H), 1.39 (t, 3H), 1.84 (m, 2H), 3.68 (q, 6H), 4.22 (m, 4H), 7.67 (s, 1H), 7.85 (s, 1H), 9.46 ppm (s, 1H); 13C-NMR (75 MHz, DMSO): δ 7.5, 15.3, 18.4, 23.8, 43.8, 51.0, 56.0, 119.7, 122.1, 134.3 ppm.
1-Butyl-3-(3-triethoxysilylpropyl) imidazolium iodide (IL6).
1H-NMR (300 MHz, DMSO): δ 0.52 (m, 2H), 0.89 (t, 3H), 1.04 (t, 9H), 1.23 (m, 2H), 1.78 (m, 4H), 3.72 (q, 6H), 4.19 (m, 4H), 7.68 (s, 1H), 7.83 (s, 1H), 9.28 ppm (s, 1H); 13C-NMR (75 MHz, DMSO): δ 7.5, 13.3, 18.2, 18.7, 24.0, 31.4, 48.5, 51.5, 57.7, 119.5, 122.2, 135.0 ppm.
Synthesis of IMIS and IMIS-Zn
Prior to immobilization, commercial silica were purified with hot piranha solution (H2O2 and H2SO4) followed by rinsing in water and drying under a stream of nitrogen. The pretreated silica and ionic liquid were co-dispersed in anhydrous toluene in flask. The mixture was refluxed for 24 h under nitrogen atmosphere. The resultant product was filtered and washed with dichloromethane to remove excess ionic liquid. After evaporating the residual solvent in vacuum oven, IMIS was obtained. The preparation process of IMIS is shown in reaction (1) of Scheme 2.
To combine IMIS with zinc bromide, IMIS (4 g) was added to a 100 mL flask containing zinc bromide solution (0.05 g mL−1 in acetonitrile). The mixture was refluxed for 24 h under nitrogen atmosphere. Acetonitrile was removed at a reduced pressure and excess zinc halide was removed by Soxhlet extraction with acetone for 24 h. The as-prepared IMIS combined with zinc salt is denoted as IMIS-Zn. The synthetic procedure is shown in reaction (2) of Scheme 2.
Coupling reactions
Allyl glycidyl carbonate (AGC) was synthesized by the coupling reaction between AGE and CO2 in the presence of IMIS or IMIS-Zn as illustrated in Scheme 3. All the reactions were carried out in a 55 mL stainless-steel reactor with a magnetic stirrer. In a typical reaction process, 0.5 g of catalyst was introduced into a reactor containing 40 mmol of AGE. The reaction was carried out under a preset pressure of carbon dioxide at different temperature.
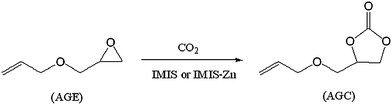 |
| Scheme 3 Synthesis of AGC from AGE and CO2 using IMIS or IMIS-Zncatalysts. | |
Characterization
The elemental analysis (EA) was carried out using Vario EL III. 2 mg of the samples were subjected to 1100 °C and sulfanilic acid was used as a standard. Solid-state NMR was carried out with 29Si and 13C frequencies of 79.5 and 100.6 MHz, respectively, on an INOVA-400 WB MAS probe. 29Si magic-angle spinning (MAS) spectra were measured at room temperature with the following conditions: magic-angle spinning at 5 kHz; π/2 pulse, 6.5 μs and a repetition delay of 60 s; 3928 scans which referenced to tetramethylsilane. 13C cross-polarization spectra were measured with a recycle delay of 5 s, 1024 scans with the following conditions: magic-angle spinning at 5 kHz; π/2 pulse, 7 μs. The spectra were referenced to tetramethylsilane. Fourier transformation infrared (FT-IR) spectra were obtained on an AVATAR 370 Thermo Nicolet spectrophotometer with a resolution of 4 cm−1. Thermal gravimetric analysis (TGA) was operated on a Netzsch STA 449 instrument with a heating rate of 10 °C min−1 from 40 °C to 800 °C under nitrogen atmosphere. AGE concentration was determined by gas chromatography (HP 6890 A) equipped with a HP-1 capillary column. The peak of AGC was identified by the authentic sample.
Results and discussion
Characterizations of catalysts
FT-IR analysis.
The imidazolium-based ionic liquids with varied alkyl chain lengths bearing different anions (Cl−, Br− and I−) were synthesized and immobilized onto the surface of the commercial silica gel. The resulting heterogeneous catalysts (IMISs) and pristine silica were characterized by means of FT-IR analysis. As shown in Fig. 1, IMISs exhibited the characteristic bands of aromatic C–H stretching (3130, 2950 and 2850 cm−1), ring stretching of the imidazolium molecule (1560 and 1460 cm−1), and the C–Si stretching (630 cm−1) vibrations (curves b–g). However, pristine silica didn't show these bands. This indicates that the ILs are successfully grafted onto the silica surface.
Elemental analysis (EA) results.
The amount of IL grafted was determined by means of EA. To get the maximum grafting amount of IL, an effective method is to control the amount of IL employed in the grafting reaction process. The effects of the employed amount of IL1 (3, 5 and 7 mmol ionic liquid per gram silica) on the grafting amount were studied for IMIS1. Table 1 shows that the highest grafting amount (1.32 mmol g−1) of IL can be obtained when 5 mmol of IL1 was used. Therefore, IMIS2–6 were prepared by using 5 mmol IL per gram silica. The corresponding grafting amounts of ILs determined by EA are summarized in Table 2. The grafting amount of IL on IMISs ranges from 0.93 to 1.32 mmol g−1. The grafting amount of the IL component in IMISs was also determined by thermogravimetric (TG) analysis. The corresponding results are summarized in Table 3. The weight loss measured by TGA is in good agreement with the calculated one from the EA results.
Table 1
EA and grafting amount of IL in IMIS1 with different amounts of IL1
IL1/mmol |
N (wt%) |
C (wt%) |
H (wt%) |
Amount of IL/mmol g−1 |
3 |
2.913 |
7.719 |
3.426 |
1.040 |
5 |
3.705 |
9.468 |
3.519 |
1.323 |
7 |
3.440 |
9.082 |
3.896 |
1.228 |
Table 2
EA and grafting amount of IL in different IMISs
Catalyst
|
N (wt%) |
C (wt%) |
H (wt%) |
Amount of IL/mmol g−1 |
IMIS1
|
3.705 |
9.468 |
3.519 |
1.32 |
IMIS2
|
2.604 |
8.209 |
3.486 |
0.93 |
IMIS3
|
3.641 |
10.840 |
3.480 |
1.30 |
IMIS4
|
2.977 |
7.796 |
3.021 |
1.06 |
IMIS5
|
3.502 |
9.277 |
2.884 |
1.25 |
IMIS6
|
3.612 |
11.063 |
3.605 |
1.29 |
Table 3 Weight loss from TGA and calculated values based on the EA results
Catalyst
|
Weight loss from TGA (%) |
Calculated weight loss (%) |
IMIS1
|
29.8 |
30.5 |
IMIS2
|
25.7 |
25.5 |
IMIS3
|
40.9 |
41.4 |
IMIS4
|
33.7 |
32.3 |
IMIS5
|
37.5 |
36.3 |
IMIS6
|
46.8 |
47.2 |
29Si and 13C MAS-NMR characterization.
To investigate the efficiency of grafting reaction, a solid-state 29Si MAS-NMR analysis of IMIS3 was carried out. As shown in Fig. 2, two peaks centered at −93 and −103 ppm corresponding to Q3 (Si(OSi)3 OH) and Q4 (Si(OSi)4) silicon atoms, respectively. The peaks situated at −61.9, −50.3 and −42.2 ppm are assigned to T3 (Si(OSi)3R), T2 (Si(OSi)2ROH) and T1 (Si(OSi)3R(OH)2) organosiloxane, which indicates the presence of organic functionalization moieties as the part of the silica.
Fig. 3 shows the 13C MAS-NMR spectrum of IMIS3. The chemical shifts at 135 and 150 ppm correspond to the three imidazole ring carbon atoms. The signal at 65 ppm is attributed to the carbon atoms connecting on imidazole ring and other carbon atoms give peaks from 25 to 47 ppm.
Synthesis of AGC from AGE and CO2
Effect of ionic liquid structure.
The effects of molecular structure (alkyl chain length and type of anion) of grafted ionic liquid on synthesis of AGC were studied and the results are shown in Table 4. IMIS1 showed the higher catalytic reactivity towards the conversion of AGE, but much lower selectivity than IMIS2 did. Therefore, IMIS2–6 were used to investigate the effect of catalyst structure. The AGE conversions of IMIS2–6 ranged from 20.6 to 77.8% with over than 95% of AGC selectivity. This high selectivity could be attributed to negligible amount of byproducts.
Table 4 Effect of different structure of IMIS on the synthesis of AGCa
Run |
Catalyst
|
Conversion (%) |
Selectivity (%) |
TONb |
Reaction conditions: AGE = 40 mmol; Catalyst = 0.5 g; T = 110 °C; CO2 pressure = 110 psig; reaction time = 3 h, without solvent.
Turnover number (TON): number of moles of product per mole of immobilized IL.
Reaction time = 6 h; other conditions are the same as previous one.
|
1c |
IMIS1
|
51.0 |
65.8 |
20.3 |
2c |
IMIS2
|
36.4 |
100.0 |
31.3 |
2 |
IMIS2
|
20.6 |
100.0 |
17.7 |
3 |
IMIS3
|
76.6 |
96.5 |
45.5 |
4 |
IMIS4
|
74.6 |
98.0 |
55.1 |
5 |
IMIS5
|
67.5 |
95.2 |
41.1 |
6 |
IMIS6
|
77.8 |
100.0 |
48.2 |
From runs 3, 4 and 5, it is observed that AGE conversions catalyzed by IMIS3, 4, 5 are 76.6%, 74.6% and 67.5%, respectively. This illustrates that AGE conversion increased with increasing alkyl chain length (from –Et to –Pr to –Bu) of the grafted ILs. These results are in agreement with the previous reports on the effects of alkyl chain length.17,26 It could be explained that increasing the bulkiness of alkyl chain forces the halide ions away from the cation more easily, resulting in less electrostatic interaction between anion and cation and consequently increasing the availability of the anion.
It is generally known that the type of anion in an IL has a significant effect on the catalytic performance of the IL.1,4,8IMISs with different halide anions, Cl− (IMIS2), Br− (IMIS3) and I− (IMIS6) were used to catalyze the coupling reaction between AGE and CO2. As is shown in runs 2, 3 and 6, the AGE conversion increased in the order of IMIS2 (20.6%) < IMIS3 (76.6%) < IMIS6 (77.8%). This phenomenon could be explained in terms of nucleophilicity of anions in supported ILs. It is well known that the ionic radius increases from Cl− < Br− < I− and electronegativity decreases from Cl− > Br− > I−, thus making the order of distance between the imidazole cation and anion as I− > Br− > Cl−, which is the order of nucleophilicity. Generally, anions with higher nucleophilicity are favorable for high AGE conversion.27 This fact could be responsible for the increasing AGE conversion in the order of Cl− < Br− < I−. It is noting that IMIS with the I− anion (IMIS6) showed not only high AGE conversion (77.8%) but also high selectivity (100%).
Effect of temperature, pressure and reaction time
Since IMIS3 showed good catalytic activity, the factors of reaction temperature, reaction time, and the pressure of CO2 were examined using IMIS3. Fig. 4 shows that the conversion of AGE increased sharply during the early stages. When the reaction proceeded for 2 and 3 h, the AGE conversion reached 93.5 and 98.9%, respectively. After 3 h, the AGE conversion remained nearly constant.
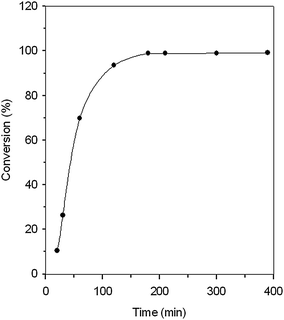 |
| Fig. 4 Time variant conversion of AGE for IMIS3 at 110 °C and CO2 pressure of 360 psig. | |
Table 5 lists the conversion of AGE at different reaction temperatures and pressures of CO2. As is shown in runs 3a–3d, the AGE conversion increased from 70.2% to 98.9% with increasing reaction temperature from 80 to 110 °C. In runs 3e–3 h, AGE conversion increased from 76.6% to 98.9% with increasing CO2 pressure from 110 to 360 psig. IMIS3 exhibited the highest catalytic activity under 240 psig of CO2 pressure and it decreased slightly at 360 psig. Such an effect of CO2 pressure on catalytic activity has also been observed in other catalytic systems.14,22 This is possible because the higher pressure can improve the concentration of CO2 by increasing its solubility, and can shift the equilibrium of the reaction towards the forming of AGC. However, when pressure increased from 240 to 360 psig, AGE conversion decreased a little. It can be explained that too high a CO2 pressure may retard the interaction between AGE and the catalyst by the dilution effect, resulting in a lower conversion.
Table 5 Effect of temperature, reaction time and CO2 pressure on the synthesis of AGCa
Run |
Catalyst
|
T/°C |
Pressure/psig |
Conversion (%) |
Reaction conditions: AGE = 40 mmol; catalyst = 0.5 g; reaction time = 3 h; without solvent.
|
3a
|
IMIS3
|
80 |
360 |
70.2 |
3b
|
IMIS3
|
90 |
360 |
88.0 |
3c
|
IMIS3
|
100 |
360 |
93.5 |
3d
|
IMIS3
|
110 |
360 |
98.9 |
3e
|
IMIS3
|
110 |
110 |
76.6 |
3f
|
IMIS3
|
110 |
180 |
99.0 |
3 g |
IMIS3
|
110 |
240 |
99.2 |
3 h |
IMIS3
|
110 |
360 |
98.9 |
Effect of zinc halide
In order to understand the role of zinc halide, IMIS-Zn was synthesized by the reaction of IMIS with zinc halide. The catalytic activities of IMIS-Zn towards coupling reaction of AGE and CO2 are shown in Table 6. In comparison with the catalyst system without zinc halide (Table 4), the zinc halide-combined complex catalyst bearing Cl− could effectively improve catalytic activity, while IMIS-Zn with Br− and I− anions exhibited lower activity than IMIS itself. These interesting results can be explained based on two different aspects namely (a) separation of counter anion and (b) charge-to-volume ratio on resultant zinc halide complex. With regard to (a), Zhong et al.28 reported that [Bmim]2[NiCl4], containing a distorted [NiCl4]2− tetrahedron structure, induced delocalization of electron density on donated Cl− ions. This increased the availability of the polarization of carbon dioxide. When IMIS was combined with ZnCl2, the resulting complex exhibited a similar structure.29 Therefore, IMIS-Zn bearing Cl− showed better reactivity than the non-zinc-halide-grafted material. With regard to (b), it is well known that the charge-to-volume ratio of halogen anion follows the order of I− < Br− < Cl−. Consequently, iodine- or bromine-based coordination complexes with lower charge-to-volume ratios possess a weaker polarization ability with respect to carbon dioxide, thus reducing the reactivity. Although the formation of a [ZnBr4]2− or [ZnI4]2− tetrahedron structure is favorable for high reactivity, the effect of the charge-to-volume ratio of iodine and bromine plays a predominant role in the reaction, resulting in the lower reactivity of IMIS-Zn bearing Br− and I− than the non-zinc-halide-grafted material.
Table 6
Catalytic performance of IMIS-Zn on the cycloaddition reactiona
Run |
Catalyst
|
Conversion (%) |
Selectivity (%) |
Reaction conditions: AGE = 40 mmol; catalyst = 0.5 g; T = 110 °C; CO2 pressure = 110 psig; reaction time = 3 h; without solvent.
Reaction time = 6 h, other conditions are the same as previous one.
|
1b |
IMIS1-Zn
|
74.7 |
94.1 |
2b |
IMIS2-Zn
|
73.3 |
90.5 |
2 |
IMIS2-Zn
|
41.9 |
90.5 |
3 |
IMIS3-Zn
|
46.5 |
91.6 |
4 |
IMIS4-Zn
|
52.1 |
90.7 |
5 |
IMIS5-Zn
|
58.3 |
94.3 |
6 |
IMIS6-Zn
|
60.4 |
93.4 |
Effect of host materials
The effects of the textural properties of silica support on the catalytic activity of the corresponding heterogeneous catalysts towards the fixation CO2 on AGE were also investigated. IL3 was grafted onto the surfaces of three types of commercial silica with different surface areas and average pore size. As demonstrated in Table 7, the grafting amount of IL and AGE conversion increased from S1 < S3 < S2. This could be related to the substance diffusion within the silica matrix. In the case of S1, the low surface area of the support directly leads to the low amount of grafted ILs, resulting in the lowest AGE conversion although the large pore size of S1 allows the IL molecules to diffuse freely within the silica matrix. When the silica with smaller pore sizes (S3 and S2) were used, the substance diffusion effects played a main role during IL-immobilization. Therefore, although S3 possesses higher surface area than S2, S3-IL demonstrates a lower grafting amount of IL than S2-IL. Therefore, S3-IL exhibits lower conversion than S2-IL.
Table 7 Effect of the different type of commercial silica on the grafting amount of IL and their reactivity in synthesis of AGCa
Catalyst
|
Commercial silica |
Grafted silica |
IL amount/mmol g−1 |
Conversion (%) |
Surface area/m2 g−1 |
Average pore size/nm |
Surface area/m2 g−1 |
Average pore size/nm |
Reaction conditions: AGE = 40 mmol; catalyst = 0.5 g; T = 110 °C; CO2 pressure = 110 psig; reaction time = 3 h; without solvent.
|
S1-IL
|
70–170 |
26–34 |
101 |
7.9 |
0.360 |
52.7 |
S2-IL
|
500 |
6.0 |
209 |
1.9 |
1.300 |
76.6 |
S3-IL
|
800 |
2.2 |
38 |
1.5 |
0.996 |
62.1 |
Recycling experiments have also been carried out to investigate the reusability of the resulting heterogeneous catalysts. Fig. 5 shows the performance of the catalyst IMIS3 and IMIS2-Zn in the coupling reaction of AGE and CO2. The results indicated that these catalysts were stable for up to five consecutive runs without any considerable loss of their initial activities.
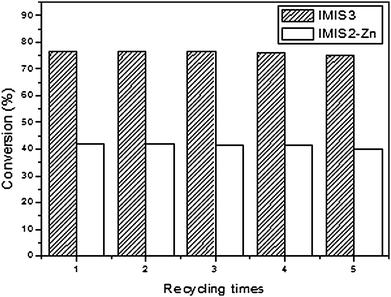 |
| Fig. 5 Recycling experiments for IMIS3 and IMIS2-Zn at 110 °C and CO2 pressure of 110 psig for 3 h. | |
Conclusions
IMISs were successfully synthesized by grafting ionic liquids onto the surface of commercial silica. The resulting heterogeneous catalysts exhibited varied catalytic activity in the coupling reaction of AGE and CO2 depending on the factors such as the molecular structure of IL, reaction temperature, CO2 pressure and type of zinc salt as well as the textural properties of the silica supports. It was found that longer alkyl chains, higher nucleophilicity of the anions and higher reaction temperature were favorable for high AGE conversion. CO2 pressure at 240 psig exhibited the highest AGE conversion. It is worth noting that IMIS-Zn, a combination of zinc salt with IMIS, can effectively increase catalytic activity for IMIS-Zn with Cl−, while the catalytic activity decreases for the other anions. In addition, the textural properties of silica supports have a great influence on the amount of immobilized IL and catalytic activity, owing to the confined reactant diffusion effects within the support matrix. The recycling experiments confirmed that the IMISs exhibited good reusability.
Acknowledgements
This work was supported by the Korea Science and Engineering Foundation (R01-2009-0053022) and Brain Korea 21 program.
References
- D. J. Darensbourg and M. W. Holtcamp, Coord. Chem. Rev., 1996, 153, 155–174 CrossRef CAS
.
- D. H. Gibson, Coord. Chem. Rev., 1999, 185–186, 335–355 CrossRef CAS
.
- M. Shi and Y. M. Shen, Curr. Org. Chem., 2003, 7, 737–745 CAS
.
- T. Sakakura, J. C. Choi and H. Yasuda, Chem. Rev., 2007, 107, 2365–2387 CrossRef CAS
.
- N. Kihara, N. Hara and T. Endo, J. Org. Chem., 1993, 58, 6198–6202 CrossRef CAS
.
- T. Iwasaki, N. Kihara and T. Endo, Bull. Chem. Soc. Jpn., 2000, 73, 713–719 CrossRef CAS
.
- T. Yano, H. Matsui, T. Koike, H. Ishiguro, H. Fujihara, M. Yoshihara and T. Maeshima, Chem. Commun., 1997, 1129–1130 RSC
.
- B. M. Bhanage, S. Fujita, Y. Ikushima and M. Arai, Appl. Catal., A, 2001, 219, 259–266 CrossRef CAS
.
- H. Yasuda, L. N. He and T. Sakakura, J. Catal., 2002, 209, 547–550 CrossRef CAS
.
- M. Tu and R. J. Davis, J. Catal., 2001, 199, 85–91 CrossRef CAS
.
- H. Kawanami and Y. Ikushima, Chem. Commun., 2000, 2089–2090 RSC
.
- Y. M. Shen, W. L. Duan and M. Shi, Adv. Synth. Catal., 2003, 345, 337–340 CrossRef CAS
.
- R. Srivastava, D. Srinivas and P. Ratnasamy, Catal. Lett., 2003, 91, 133–139 CrossRef CAS
.
- J. J. Peng and Y. Q. Deng, New J. Chem., 2001, 25, 639–641 RSC
.
- H. Xie, S. Li and S. Zhang, J. Mol. Catal. A: Chem., 2006, 250, 30–34 CrossRef CAS
.
- F. Ono, K. Qiao, D. Tomida and C. Yokoyama, J. Mol. Catal. A: Chem., 2007, 263, 223–226 CrossRef CAS
.
- H. Kawanami, A. Sasaki, K. Matsui and Y. Ikushima, Chem. Commun., 2003, 896–897 RSC
.
- J. Sun, S. Fujita and M. Araj, J. Organomet. Chem., 2005, 690, 3490–3497 CrossRef CAS
.
- J. Sun, S. Fujita, F. Zhao and M. Araj, Green Chem., 2004, 6, 613–616 RSC
.
- H. S. Kim, J. J. Kim, H. Kim and H. G. Jang, J. Catal., 2003, 220, 44–46 CrossRef CAS
.
- M. Alvaro, C. Baleizao, D. Das, E. Carbonell and H. Garcia, J. Catal., 2004, 228, 254–258 CrossRef CAS
.
- L. F. Xiao, F. W. Li, J. Peng and C. Xia, J. Mol. Catal. A: Chem., 2006, 253, 265–269 CrossRef CAS
.
- S. Udayakumar, S. W. Park, D. W. Park and B. S. Choi, Catal. Commun., 2008, 9, 1563–1570 CrossRef CAS
.
- S. Udayakumar, M. K. Lee, H. L. Shim, S. W. Park and D. W. Park, Catal. Commun., 2009, 10, 659–664 CrossRef CAS
.
- Y. Xie, Z. Zhang, T. Jiang, J. He, B. Han, T. Wu and K. Ding, Angew. Chem., Int. Ed., 2007, 46, 7255–7258 CrossRef CAS
.
- S. S. Wu, X. W. Zhang, W. L. Dai, S. F. Yin, W. S. Li and Y. Q. Ren, Appl. Catal., A, 2008, 341, 106–111 CrossRef CAS
.
- J. Sun, L. Wang, S. Zhang, Z. Li, X. Zhang, W. Dai and R. Mori, J. Mol. Catal. A: Chem., 2006, 256, 295–300 CrossRef CAS
.
- C. Zhong, T. Sasaki, M. Tada and Y. Iwasawa, J. Catal., 2006, 242, 357–364 CrossRef CAS
.
- T. Sasaki, M. Tada, C. Zhang, T. Kume and Y. Iwasawa, J. Mol. Catal. A: Chem., 2008, 279, 200–209 CrossRef CAS
.
|
This journal is © The Royal Society of Chemistry 2009 |