DOI:
10.1039/B805758N
(Editorial)
J. Mater. Chem., 2008,
18, 2295-2297
Theme issue: materials chemistry for hydrogen storage and generation
Abstract
This theme issue of Journal of Materials Chemistry highlights some of the latest innovative research in materials relating to hydrogen generation and storage. This is one of the foremost examples of how materials chemistry can enable the new technologies that we require to solve today's major problems, here specifically in energy and environment. John Irvine, the Guest Editor, introduces the issue and the importance of the field.
Context
With the twin dilemmas of global warming and growing shortage of easily obtainable fossil fuel supplies we need to dramatically restructure our energy economy. Hydrogen is a carbon free energy vector, whose oxidation yields only water. Thus, there is very considerable interest in hydrogen as a vector especially for the future transport industry and transport accounts for around one third of energy usage in the developed world (Fig. 1). Hydrogen is not the best vector for stationary power due to conversion cycle inefficiencies;1 however, if a hydrogen network existed then it certainly would also be used for stationary power and heat. Importantly hydrogen may be considered as an appropriate vector for storage of excess power produced by intermittent sources or arising from ineffective load matching. There are two main technological barriers to the introduction of a hydrogen economy: storage and low-carbon-footprint production of low cost hydrogen. Materials chemistry is an essential element to finding solutions for these problems. |
| Fig. 1 Summary of the hydrogen economy. Upper part: production; lower part: uses. Reproduced from Hydrogen Energy and Fuel Cells: A vision of our future (EUR 20719 EN), Office for Official Publications of the European Communities, Luxembourg, 2003. ISBN: 92-894-5589-6 (http://ec.europa.eu/research/energy/pdf/hlg-vision-report-en.pdf). © European Communities, 2003. | |
Although, using current technology, hydrogen is not that good a vector in terms of energy storage density when compared to liquid hydrocarbons (Fig. 2), it is certainly a very attractive vector for renewable energy because of its relative ease of conversion. There is therefore considerable interest in improving both the volumetric and gravimetric energy densities for hydrogen storage. The US DOE has set very challenging targets of 90 g kg−1 and 81 g L−1 of H2 by 20152 with the objective of implementing wide scale application of hydrogen in transport. This target is being addressed by a wide range of approaches including compression and liquefaction, but primarily by materials approaches including metal hydrides, clathrates, metal organic frameworks, nanoscale carbons and chemical hydride stores. This is a rich and fertile area of materials chemistry.
![Volume of 4 kg of hydrogen compacted in different ways, with size relative to the size of a car. (Reprinted by permission from Macmillan Publishers Ltd. [Nature] (L. Schlapbach and A. Züttel, Nature, 2001, 414, 353–358), copyright (2001).)](/image/article/2008/JM/b805758n/b805758n-f2.gif) |
| Fig. 2 Volume of 4 kg of hydrogen compacted in different ways, with size relative to the size of a car. (Reprinted by permission from Macmillan Publishers Ltd. [Nature] (L. Schlapbach and A. Züttel, Nature, 2001, 414, 353–358), copyright (2001).) | |
Hydrogen can only be as environmentally friendly as the primary energy source used to produce it. It is desired therefore that renewable energy, or perhaps nuclear, will be the major source of sustainable hydrogen. At present, production of hydrogen from renewables is generally much more costly than from fossil fuels and so clean hydrogen is not economically favoured. It is projected that hydrogen production will become much more cost competitive by 2030 (Fig. 3), see HyWays roadmap for further details.3
 |
| Fig. 3 Portfolio analysis of 2030 hydrogen production pathways reproduced from http://www.hyways.de/docs/Brochures_and_Flyers/HyWays_Roadmap_FINAL_22FEB2008.pdf. © European Communities, 1995–2008. | |
There are a number of possible hydrogen production technologies, photochemical and photoelectrochemical routes utilising solar energy, thermolysis using solar concentrators, or nuclear reactor waste heat, electrolysis using low carbon electricity and catalytic and membrane-based chemical conversion routes from biomass (Fig. 4). Underpinning all of this is the materials chemistry, providing new materials, optimising structure at nano- and micro-scales and probing the relations between composition, structure and function.
 |
| Fig. 4 Technical schematic of possible hydrogen production pathways. Adapted from J. A. Turner, Science, 1999, 285, 687 and European Hydrogen & Fuel Cell Technology Platform. Deployment Strategy, August 2005, available from: https://http://www.hfpeurope.org/hfp/keydocs. | |
The purpose of this theme issue is to highlight some of the leading edge materials chemistry research addressing these key features that might one day enable a hydrogen economy. The topics addressed in this issue relate to electrolytic production of hydrogen, photochemical production of hydrogen and storage of hydrogen.
Electrolytic production of hydrogen
The electrolytic production of hydrogen from water is a long established process; however, there is considerable interest in improving both cost and energy efficiencies. The electrical efficiency of the process can be improved by utilising much higher temperatures where the electrical inputs are replaced by lower value thermal inputs and overpotential losses are decreased, hence, production cost is reduced. Hauch et al. review high temperature electrolysis of steam and report some very cost effective performances based upon state of the art solid oxide fuel cells applying these to solid oxide electrolysis cells (SOEC). Gorte et al. report on the use of ceria–stabilised zirconia materials as novel electrode components for both SOFCs and SOECs whilst Yang and Irvine report on the application of a perovskite SOEC cathode or cathode component that is compatible with start up in direct steam, which is desirable for smaller scale intermittent operation.Photochemical production of hydrogen
The direct conversion of water to hydrogen using solar energy is a very exciting possibility; however, for this to become a large scale applicable process considerable improvements in energy density and system efficiency need to be achieved by finding better materials and structures and achieving better understanding of the component functions. Two different approaches to the photochemical production of hydrogen are presented: photocatalysis of dispersed nanoparticles and development of photoanodes. van de Krol reviews the operating principles for photoelectrochemical water splitting and device concepts highlighting developments with nanostructured oxides. Hoffmann et al. report two studies based on nanocomposites involving CdS using TiO2 in one case and KNbO3 in the other to assist with charge separation in CdS. Grimes et al. have created TiO2 nanotube bundles and apply these as photoanodes to assist with water splitting. Augustynski et al. discuss metal oxide photoanodes and their coupling with photovoltaics to form a tandem photocell device.Storage of hydrogen
As discussed above the inability to store hydrogen at high density is a severe limitation for the prospects of a hydrogen economy, as if the density is insufficient then there are unacceptable losses in moving hydrogen around the energy system. New materials and concepts are urgently sought. The approaches discussed in this theme issue relate to metal hydrides, amides and chemical hydrides. Gregory reviews the materials chemistry of chemical hydrides. Hauback et al. have performed a combined modelling and experimental study of the transformation mechanisms of the aluminium deuteride system. The mixed lithium/sodium system is reported by Edwards et al., and the challenges and opportunities of using ammonia as a hydrogen vector are reviewed by Nørskov et al.I would like to thank all the authors for their efforts in submitting a rich variety of high-quality peer-reviewed contributions. I also wish to thank the editorial and production staff at the RSC for their superb assistance. Finally, I hope that this timely theme issue will provide a valuable reference and perspective for the research community working in this important field.
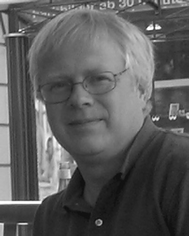 |
| Plate1 John Irvine | |
References
- E4 tech, Element Energy, Eoin Lees Energy (December 2004), A strategic framework for hydrogen energy in the UK, Final report to the Department of Trade and Industry, UK, available from: http://www.berr.gov.uk/files/file26737.pdf.
- http://www1.eere.energy.gov/vehiclesandfuels/pdfs/program/hydrogen_storage_roadmap.pdf.
- http://www.hyways.de/docs/Brochures_and_Flyers/HyWays_Roadmap_FINAL_22FEB2008.pdf.
|
This journal is © The Royal Society of Chemistry 2008 |
Click here to see how this site uses Cookies. View our privacy policy here.