DOI:
10.1039/C5RA02728D
(Paper)
RSC Adv., 2015,
5, 28299-28304
Enhanced near-infrared emission by co-doping Ce3+ in Ba2Y(BO3)2Cl:Tb3+, Yb3+ phosphor
Received
12th February 2015
, Accepted 12th March 2015
First published on 12th March 2015
Abstract
Ce3+–Tb3+–Yb3+ tri-doped Ba2Y(BO3)2Cl phosphors with intense near-infrared (NIR) emission and broad band absorption in the near ultraviolet region were synthesized by a convenient solid-state reaction in a reducing atmosphere, and the structure and the phase purity of all samples were characterized using X-ray diffraction (XRD). The visible and NIR emission spectra together with corresponding decay curves have also been measured to investigate the energy transfer (ET) processes and analysis ET mechanisms and efficiencies in Ce3+–Tb3+–Yb3+ tri-doped system. In addition, steady and dynamic luminescent properties of Tb3+/Ce3+–Yb3+ co-doped Ba2Y(BO3)2Cl phosphors were also measured as a comparison. As an excellent sensitizer, Ce3+ ion could significantly enlarge the absorption cross-section and greatly enhance the NIR emission intensity of Yb3+ ion. It is believed that Ce3+–Tb3+–Yb3+ tri-doped Ba2Y(BO3)2Cl phosphor is a promising down-conversion (DC) solar spectral convertor to enhance the efficiency of the silicon solar cells.
1. Introduction
Due to the global energy crisis, much attention has been paid to green and renewable energy. Solar cells are recognized as the most promising devices to convert solar energy to electricity, while silicon solar cells occupy a dominant position in the solar market.1–3 The major energy loss in crystalline Si (c-Si) solar cells is a spectral mismatch between the incident solar spectrum and the silicon spectral response, which is the main reason for the lower photoelectric conversion efficiency.4 The photons with lower energy than the band gap (Eg ≈ 1.12 eV, λ ≈ 1100 nm) of silicon are not absorbed, while the photons with much higher energy than the band gap lose their excess energy through thermalization of hot charge carriers.5 A promising method to reduce the thermalization effect is to turn one ultraviolet-visible (UV-Vis) high energy photon into one or more available near infrared (NIR) low energy photons via down-conversion (DC) luminescent materials, including down shift (DS, shift short-wavelength light to long-wavelength light) and quantum cutting (QC, split one incident high energy photon into two near infrared photons) processes. Since efforts are being made to enhance the efficiency of silicon solar cells, the highest efficiency is not limited to 30% (the Shockley–Queisser limit) via spectral modification with DC phosphors.6,7
Recently, RE3+–Yb3+ (RE = Tb3+, Pr3+, Tm3+, Ho3+ or Er3+) ion pairs co-doped QC materials have been extensively investigated, in which Yb3+ ion is a suitable emitter and acceptor with its emission around 1000 nm NIR and matches well with the band gap of c-Si due to its simple energy levels of 2F5/2 and 2F7/2 (separated by ∼10
000 cm−1).8 According to the quantum cutting concept, one blue photon absorbed by RE3+ due to the absorption of Tb3+: 7F6 → 5D4 around 486 nm, Pr3+: 3H4 → 3P2 around 445 nm, Ho3+: 5F3 → 5I8 around 447 nm and so on could be divided into two NIR photons of Yb3+ ion by the 2F5/2 → 2F7/2 transition, which may lower the adverse thermalization effect caused by excess energy.9 However, these RE3+ ions suffer from narrow absorption cross sections (in the order of 10−21 cm2) and low absorption intensity due to the forbidden 4f–4f transitions. Thus, the NIR emission from Yb3+ is so weak that application of RE3+–Yb3+ (RE = Tb3+, Pr3+, Tm3+, Ho3+ or Er3+) co-doped QC materials in silicon solar cells is limited.10–13 Unlike other RE3+ ions, Ce3+ ions with allowed 4f–5d transitions generally have broad and strong absorption (in the order of 10−18 cm2) in the UV-Vis region.14 Ce3+ ion could be used as an efficient sensitizer and transfer its energy absorbed from the UV-Vis region to Tb3+ or Yb3+ effectively.15 In addition, cooperative energy transfer (CET) mechanisms of RE3+ → 2Yb3+ (e.g. RE = Ce3+, Tb3+, Pr3+, Tm3+) were theoretically realizable and had been investigated in detail, which is a possible way of energy transfer (ET) in RE3+–Yb3+ pair.16,17 According to our previous result, phosphor Ba2Y(BO3)2Cl:Ce3+, Tb3+ exhibited both a blue emission from Ce3+ and a green emission from Tb3+ under near ultraviolet light excitation in the range 320–390 nm, energy transfer from Ce3+ to Tb3+ was efficient.18 Therefore, the energy transfer from Ce3+ to Tb3+–Yb3+ ion pairs can occur.
In this paper, we investigated Ce3+–Tb3+–Yb3+ tri-doped Ba2Y(BO3)2Cl phosphor. The visible and NIR emission spectra and corresponding decay curves were measured to prove the occurrence of ET in the present system, and the ET mechanisms were systematically studied and discussed. The results demonstrate that Ce3+ ion could efficiently sensitize the Tb3+–Yb3+ pair and enhance the NIR emission of Yb3+ ion greatly.
2. Experimental
All the Ba2Y(BO3)2Cl:Tb3+/Ce3+, Yb3+ and Ba2Y(BO3)2Cl:Ce3+, Tb3+, Yb3+ powder samples were prepared by a conventional solid-state reaction in a reducing atmosphere. Analytical grade BaCO3, BaCl2·2H2O, H3BO3 (excess 5 mol% to compensate for the evaporation), and high purity (99.99%) rare earth oxides Y2O3, Yb2O3, Tb4O7, CeO2 were used as raw materials. The composition for each sample was weighed in proper stoichiometric ratio and mixed thoroughly in an agate mortar. The mixture samples were preheated at 500 °C for 2 h in air. After being cooled to room temperature, the sample was thoroughly reground and heated for 4 h in a CO atmosphere at 900 °C to ensure the complete reduction of Ce3+ and Tb3+. It was then cooled to room temperature and crushed into a fine powder.
The X-ray diffraction (XRD) patterns were performed on a Rigaku-Dmax 3C powder diffractometer (Rigaku Corp, Tokyo, Japan) with Cu-Kα radiation (λ = 1.54065 Å) to identify the structure and phase purity. The photoluminescence emission (PL) and excitation (PLE) spectra and decay curves were recorded using an Edinburgh FLS920 spectrofluorometer with a 450 W Xe lamp as excitation source. Decay curve measurements of Ce3+ ion were obtained using a 375 nm nanosecond flash-lamp (nF900) as the excitation source. Photoluminescent spectra of all samples were tested three times to reduce the error and all of the measurements were performed at room temperature.
3. Results and discussions
3.1 Phase and structure
Fig. 1 shows the X-ray diffraction (XRD) patterns of Tb3+ solely doped, Tb3+–Yb3+ co-doped and Ce3+–Tb3+–Yb3+ tri-doped Ba2Y(BO3)2Cl samples, respectively. The diffraction peaks of all samples agree well with those of the standard profile (JCPDS no. 79-0967) Ba2Yb(BO3)2Cl and not any diffraction peaks from impurity. It is noted that all samples show similar XRD patterns in our experiments, which indicate that the obtained samples are single and pure phases, and RE3+ (Ce3+/Tb3+/Yb3+) ions completely enter the lattices even at high concentrations. Here, most of the rare earth ion dopants would prefer to occupy a Y3+ site in the Ba2Y(BO3)2Cl compound according to the close effective ionic radius and valence of the cations. Compound Ba2Y(BO3)2Cl shares the same structure with Ba2Yb(BO3)2Cl, which crystallizes in a monoclinic structure with the space group P21/m, having the lattice parameters of a = 6.397 Å, b = 5.279 Å, c = 11.222 Å.19
 |
| Fig. 1 XRD patterns of phosphors Ba2Y(BO3)2Cl:Tb3+, Ba2Y(BO3)2Cl:Tb3+, Yb3+ and Ba2Y(BO3)2Cl:Ce3+, Tb3+, Yb3+ along as well with the standard profile for Ba2Yb(BO3)2Cl (JCPDS card no. 79-0967). | |
3.2 Analysis of photoluminescence spectra and energy transfer processes
3.2.1 Luminescence and ET in Tb3+–Yb3+ or Ce3+–Yb3+ codoped Ba2Y(BO3)2Cl. Photoluminescence excitation (PLE) and emission (PL) spectra of the Ba2Y(BO3)2Cl:0.03Tb3+, 0.20Yb3+ and Ba2Y(BO3)2Cl:0.03Ce3+, 0.20Yb3+ samples are presented in Fig. 2a and b, respectively. Monitoring the characteristic green emission of Tb3+ at 542 nm, PLE spectrum shown in Fig. 2a consists of a weak band around 280 nm and several weak peaks in the 300–500 nm due to the lowest spin-forbidden 4f → 5d transitions and the forbidden 4f → 4f transitions of Tb3+ ion.20 The amplifying excitation peak centered at 486 nm is assigned to the Tb3+: 7F6 → 5D4 transition, which is usually used as excitation peak in Tb3+–Yb3+ co-doped quantum cutting systems.21 The excitation spectrum profile monitoring at 972 nm from the 2F5/2 → 2F7/2 transition of Yb3+ is similar to that of monitoring at 542 nm, which indicates the occurrence of ET from Tb3+ to Yb3+, and the trivial difference might be from different detectors of the Vis and NIR spectrum measurements. Herein, both the emission and inset excitation intensities of Tb3+ ion are magnified 40 times for the sake of clarify. Under blue excitation at 486 nm, emissions peaking at 542, 583, 620 nm are observed, which can be assigned to the forbidden 5D4 → 7FJ (J = 5, 4, 3) transitions of Tb3+.22 Meanwhile, the characteristic NIR emission band located at 900–1150 nm is also observed, due to the 2F5/2 → 2F7/2 transition of Yb3+. The NIR emission spectra separately excited by 355 nm and 486 nm are shown in the insert of Fig. 2a, and the former integrated intensity is 6 times that of the latter. With the increase of Yb3+concentration, it is clearly found that the emission intensities of Tb3+ rapidly decrease while the Yb3+ NIR emission intensities grow simultaneously, as shown in Fig. 2c, which further confirms the occurrence of ET from Tb3+ to Yb3+.
 |
| Fig. 2 (a) PLE spectra (λem = 972 and 542 nm) and Vis-NIR PL spectrum (λex = 486 nm) of sample Ba2Y(BO3)2Cl:0.03Tb3+, 0.20Yb3+; (b) PLE spectra (λem = 972 and 417 nm) and Vis-NIR PL spectrum (λex = 355 nm) of sample Ba2Y(BO3)2Cl:0.03Ce3+, 0.20Yb3+ and dependence of normalized integrated PL intensity in Vis and NIR region on Yb3+ concentration x in Ba2Y(BO3)2Cl:0.03Tb3+, xYb3+ (c) and Ba2Y(BO3)2Cl:0.03Ce3+, xYb3+ (d). | |
Fig. 2b exhibits the PLE and PL spectra of Ba2Y(BO3)2Cl:0.03Ce3+, 0.20Yb3+ phosphor. Upon 355 nm excitation, the phosphor generates the blue emission from Ce3+ and NIR emission from Yb3+. For the PLE spectra monitoring both the 5d → 4f transition of Ce3+ at 417 nm and the 2F5/2 → 2F7/2 transition of Yb3+ at 972 nm, which are similar except for the intensity concluding an intense excitation band of Ce3+: 4f → 5d centered at 355 nm, and this indicates the energy transfer from Ce3+ to Yb3+.23 It is observed that the emission of Ce3+ decreases and the emission of Yb3+ increases with increasing the contents of Yb3+, whereas the emission intensity of Yb3+ reaches the maximum at x = 0.2 due to concentration quenching effect (as shown in Fig. 2d). Regular changes in the emission intensities from Ce3+ or Yb3+ also provide powerful evidence for an ET process from Ce3+ to Yb3+.
In order to further prove for the occurrence of the energy transfer from Tb3+/Ce3+ to Yb3+ and gain understanding of the ET process, the decay curves of the Tb3+: 5D4 → 7F5 emission at 542 nm in Ba2Y(BO3)2Cl:0.03Tb3+, xYb3+ and the Ce3+: 5d → 4f emission at 417 nm in Ba2Y(BO3)2Cl:0.03Ce3+, xYb3+ were recorded in Fig. 3a and b. The Tb3+ 542 nm emission without Yb3+ shows a nearly single exponential decay, whereas the Ce3+ 417 nm emission without Yb3+ deviates from single exponential decay because of the energy transfer between possible distribution of Ce3+ in different cation sites (two Ba2+ cites and one Y3+ site).14 For a sample without any Yb3+, the decay time of 3.46 ms and 20.31 ns are obtained for Tb3+ and Ce3+ by fitting. With the introduction of Yb3+ and increasing its concentration, their decay curves became nonexponential and the lifetime of the sensitizer Tb3+ or Ce3+ decreases. The average lifetime (τ) of sensitizer Tb3+/Ce3+ in Tb3+/Ce3+–Yb3+ co-doped Ba2Y(BO3)2Cl samples is given by:24
|
 | (1) |
where
I(t) is the luminescence intensity at time
t. The decay life time of Tb
3+/Ce
3+ drops gradually with Yb
3+ co-doping, and their lifetimes are about 3.40, 3.33, 3.32, 3.08, 2.73 ms and 10.44, 4.90, 2.83, 2.38, 1.38 ns for Tb
3+ and Ce
3+ in 0.03Tb
3+–
xYb
3+ or 0.03Ce
3+–
xYb
3+ co-doped Ba
2Y(BO
3)
2Cl, respectively.
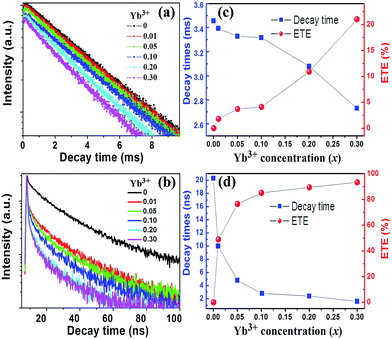 |
| Fig. 3 Decay lifetime of (a) Tb3+: 5D4 → 7F5 (542 nm) luminescence in Ba2Y(BO3)2Cl:0.03Tb3+, xYb3+ under excitation of 486 nm and (b) Ce3+: 5d → 4f (417 nm) luminescence of Ba2Y(BO3)2Cl:0.03Ce3+, xYb3+ under excitation of 375 nm; lifetime and energy transfer efficiency as function of Yb3+ concentrations in Tb3+–xYb3+ (c) and Ce3+–xYb3+ (d) co-doped Ba2Y(BO3)2Cl. | |
Fig. 3c and d present the emission average lifetime and energy transfer efficiency of Tb3+/Ce3+–Yb3+ co-doped Ba2Y(BO3)2Cl samples as function of Yb3+ concentrations. According to the decay times, the energy transfer efficiency (ETE, ηETE) can be calculated by using the following equations:25
where
τxYb and
τ0Yb denote lifetime of the sensitizer with and without Yb
3+ content. Accordingly, the ET efficiency (
ηETE) are 2.0, 3.8, 4.0, 11.0, 21.1% for Ba
2Y(BO
3)
2Cl:0.03Tb
3+,
xYb
3+ and 48.6, 75.9, 86.1, 88.3 and 93.2% for Ba
2Y(BO
3)
2Cl:0.03Ce
3+,
xYb
3+ with an increase in content of Yb
3+ from 0.01 to 0.3, respectively. The
ηETE value rises evenly with increasing Yb
3+ doping content, but the ET efficiency is much lower in Ba
2Y(BO
3)
2Cl:0.03Tb
3+,
xYb
3+ compared to that of Ba
2Y(BO
3)
2Cl:0.03Ce
3+,
xYb
3+, which may be due to the lower and inefficient forbidden 4f → 4f absorption cross-sections of Tb
3+ in UV-visible region compared to that of the allowed 4f → 5d of Ce
3+. It could be expected that the NIR emission intensity is stronger in Ce
3+–Yb
3+ co-doped Ba
2Y(BO
3)
2Cl compared with that of Tb
3+–Yb
3+ co-doped Ba
2Y(BO
3)
2Cl, which will be discussed in the following paragraph. In addition, it is found that the energy transfer efficiency of Ce
3+ → Yb
3+ is much higher than that of Ce
3+ → Tb
3+ in comparison with that of our previous results,
18 which indicates that the energy absorbed by Ce
3+ is more easily transfer to Yb
3+.
3.2.2 Luminescence in Ce3+–Tb3+–Yb3+ tri-doped Ba2Y(BO3)2Cl phosphor. According to the above-mentioned and previous results, the energy transfer Ce3+ → Tb3+ and Tb3+ → Yb3+ have occurred in the Ba2Y(BO3)2Cl host, and it is possible for the energy transfer from Ce3+ to Tb3+ → Yb3+ ion pairs to take place. Fig. 4 displays the PLE and PL spectra of Ce3+–Tb3+–xYb3+ tri-doped Ba2Y(BO3)2Cl phosphor. As the representative PLE spectra (shown in Fig. 4a), they include a broad excitation band at 320–390 nm with a maximum at 355 nm corresponding to the Ce3+: 4f → 5d transition. It is found that the profiles of PLE spectra obtained by monitoring at 542 nm (Tb3+: 5D4 → 7F5 emission), 417 nm (Ce3+: 5d → 4f emission) and 972 nm (Yb3+: 2F5/2 → 2F72 emission) are similar to that of Ce3+ solely doped Ba2Y(BO3)2Cl except relative intensity, which illuminates that the possible appearance of energy transfer from Ce3+ to Tb3+/Yb3+/Tb3+–Yb3+. Under excitation of 355 nm light, the PL spectra of Ba2Y(BO3)2Cl:0.03Ce3+, 0.03Tb3+, xYb3+ in visible and near infrared region as function of Yb3+ content are shown in Fig. 4b. The PL spectra are composed of a typical broad unsymmetrical doublet emission bands from the parity allowed transitions from the 5d excited state to the 2F7/2 and 2F5/2 ground states of Ce3+ (ref. 26) and a series of sharp lines emission in 480–680 nm region from the 5D4 → 7FJ (J = 6, 5, 4 and 3) transitions of Tb3+ in visible region, as well as the NIR emission band centered at 972 nm accompanied by several weak shoulders in 900–1150 nm region due to transitions among the different stark levels of 2FJ (J = 5/2, 7/2) of Yb3+. Seen from the PL spectra, it is observed that the intensity of Tb3+ emission (integrated from 480 to 680 nm) and Ce3+ emission (integrated from 370 to 480 nm) decreases monotonously with the increase of Yb3+ concentration from 0 to 0.3, while the NIR emission intensity of Yb3+ (integrated from 900 to 1150 nm) firstly increases with Yb3+ content growth and then begins to decrease after reaching a maximum at x = 0.20 due to concentration quenching effect. In order to clearly visualise the variation, the inset of Fig. 4b displays the variable curves of normalized PL intensity from Ce3+, Tb3+ and Yb3+ on the Yb3+ concentration x, respectively.
 |
| Fig. 4 (a) PLE and (b) PL spectra of Ba2Y(BO3)2Cl:0.03Ce3+, 0.03Tb3+, xYb3+ (x = 0, 0.01, 0.05, 0.10, 0.20, 0.30) samples as functions of NIR emission center Yb3+ concentration x. Inset of (b) shows the dependence of Vis and NIR normalized integrated emission intensity on Yb3+ concentration x. | |
The energy transfer process is complicated in Ce3+–Tb3+–Yb3+ tri-doped Ba2Y(BO3)2Cl sample. To further understand the energy transfer in this system, the decay curves of the sensitizer Ce3+ and Tb3+ in sample Ba2Y(BO3)2Cl:0.03Ce3+, 0.03Tb3+, xYb3+ (x = 0–0.3) are plotted against the concentration of Yb3+, and are shown in Fig. 5. With increasing Yb3+ concentration, the decay of Ce3+ and Tb3+(5D4) in tri-doped Ba2Y(BO3)2Cl:0.03Ce3+, 0.03Tb3+, xYb3+ sample speeds up (as shown in Fig. 5a and b), similar to those in co-doped Ba2Y(BO3)2Cl:0.03Ce3+, xYb3+ and Ba2Y(BO3)2Cl:0.03Tb3+, xYb3+. The effective decay times are calculated to be about 12.82, 9.63, 2.89, 2.60, 1.77, 1.28 ns for Ce3+ and 3.56, 3.26, 3.18, 3.06, 2.77, 2.48 ms for Tb3+, therefore the ηETE values are 36.8, 52.6, 85.8, 87.2, 91.2, 93.7% (ηETE-Ce) and 0, 8.6, 10.7, 14.0, 22.2, 30.3% (ηETE-Tb) according to the decline of Ce3+ and Tb3+ lifetime, respectively. The corresponding lifetimes and energy transfer efficiency variable curves as function of Yb3+ contents are also shown clearly in Fig. 5c and d. The ηETE-Ce values calculated on the basis of the decrease of Ce3+ lifetime do not start from 0 whereas the ηETE-Tb values do start from 0, because ηETE-Ce value is the sum of ET efficiency of Ce3+ → Tb3+ and Ce3+ → Yb3+, but ηETE-Tb is the only ET efficiency value of Tb3+ → Yb3+. Comparison with the co-doped system, it is found that the ET efficiency was higher in a tri-doped sample than those of the corresponding co-doped samples. Thus we deduced that the NIR emission intensity in Ba2Y(BO3)2Cl:0.03Ce3+, 0.03Tb3+, xYb3+ should be stronger than that of corresponding Ba2Y(BO3)2Cl:0.03Ce3+, xYb3+ or Ba2Y(BO3)2Cl:0.03Tb3+, xYb3+, which is strongly related to the energy transfer processes.
 |
| Fig. 5 Decay curves of (a) Ce3+: 5d → 4f (417 nm) emission in Ba2Y(BO3)2Cl:0.03Ce3+, 0.03Tb3+, xYb3+ (x = 0, 0.01, 0.05, 0.10, 0.20, 0.30) under excitation of 375 nm and (b) Tb3+: 5D4 → 7F5 (542 nm) emission under excitation of 355 nm; the variation trend of lifetime (Ce3+ and Tb3+) and ET efficiency of (Ce3+ → Tb3+/Yb3+ and Tb3+ → Yb3+) are shown in (c) and (d). | |
3.2.3 Energy transfer processes in Ce3+–Tb3+–Yb3+ tri-doped Ba2Y(BO3)2Cl. Based on the above-mentioned results, the possible ET processes were analyzed in detail by schematic energy level diagram with feasible transitions, which are shown in Fig. 6. In the present Ce3+–Tb3+–Yb3+ tri-doped system, the possible energy transfer processes include Ce3+ → Yb3+, Ce3+ → Tb3+, Tb3+ → Yb3+ and Ce3+ → Tb3+ → Yb3+ four procedures. For the first energy transfer process ① Ce3+ → Yb3+, it is usually considered that the cooperative down-conversion (or quantum cutting, QC) probably dominates the process because the energy of Ce3+: 5d → 4f transition is more than twice as high than the energy difference between the 2F7/2 and 2F5/2 levels of Yb3+.27 Up to now, no direct experimental proof could support the existence or inexistence of QC, whereas many measured Yb3+ quantum yield (QY) in the samples with so-called “QC” are far below 100%.28,29 The authors considered the ET Ce3+ to Yb3+ may include both Ce3+ → 2Yb3+ (1a) and Ce3+ → Yb3+ (1b) two processes: the former is a CET process and possible existence in theory, and the latter is possibly through a slow non-radiative relaxation from an intermediate Ce4+–Yb2+ charge transfer state companied with energy loss instead of directly via Ce3+: 5d → 2Yb3+: 2F5/2.29–31 The energy transfer processes ② Ce3+ → Tb3+ have been investigated in many hosts,32,33 in which part of excited energy of Ce3+ could transfer to Tb3+ due to the Ce3+ excited 5d state energy level is close to that of the 5D3 and 5D4 of Tb3+, resonant energy transfer could take place and promote the electrons of Tb3+ from 7F6 ground state to 5D3 and other excited levels. Then non-radiative relaxation occurs from the high energy levels 5D3 to the 5D4 levels, resulting in several sharp line emissions from 5D4 → 7FJ (J = 6, 5, 4 and 3) transitions of Tb3+ and peaking 486, 542, 583 and 620 nm, respectively (2).34 For the energy transfer ③ Tb3+ → Yb3+, the energy of Tb3+(5D4) level is approximately two times as high as the energy difference between the 2F7/2 and 2F5/2 levels of Yb3+, which probably makes CET or QC process Tb3+(5D4) → 2Yb3+(2F5/2) occur, the energy of the excited Tb3+ ion is transferred to two different Yb3+ ions (3a). A Tb3+–Yb3+ cross-relaxation mechanism is also possible to excite one Yb3+ with a subsequent energy loss through multi-phonon decay process in Tb3+ ion (3b).35 On the basis of the above-mentioned three ET processes, the fourth energy transfer ④ Ce3+ → Tb3+ → Yb3+ is easy to understand. Upon excitation by 355 nm n-UV light, the Ce3+ ion in the ground state 2F5/2 is excited to the higher excited state 5d level, part of the excited state electrons relax to the ground state 2F5/2 and 2F7/2 of Ce3+ in the form of broad band blue emissions, part of the excited state electrons energy transfer to Tb3+, then the part of the accepted energy of Tb3+ transfer to Yb3+ exhibiting the NIR emission of Yb3+: 2F5/2 → 2F7/2. Here, Tb3+ ions play the role of bridge to form a new energy transfer pathway. Yb3+ NIR emission could involve four energy transfer processes in Ce3+–Tb3+–Yb3+ tri-doped Ba2Y(BO3)2Cl.
 |
| Fig. 6 Schematic energy level diagram and the possible ET progresses in Ce3+–Tb3+–Yb3+ tri-doped Ba2Y(BO3)2Cl phosphor. | |
3.2.4 Comparison of NIR emission in the co-doped and tri-doped samples. The aim of the present paper is to enhance the NIR emission from Yb3+ transitions through the Ce3+–Tb3+–Yb3+ ion pairs tri-doped Ba2Y(BO3)2Cl sample. In order to compare NIR emission intensity in a co-doped system with that of a tri-doped system, Fig. 7 shows the PL spectra in NIR region of 0.03Tb3+, 0.20Yb3+ and 0.03Ce3+, 0.20Yb3+ co-doped Ba2Y(BO3)2Cl, as well as Ba2Y(BO3)2Cl:0.03Ce3+, 0.03Tb3+, 0.20Yb3+ sample with optimal composition. It is observed that the NIR emission intensity of samples doped with Tb3+ under the excitation of 486 nm (Tb3+: 7F6 → 5D4) is lower than that of samples excited with 355 nm, which due to the weak absorption and narrow absorption cross-section of Tb3+ at 486 nm. Under excitation of 355 nm, the NIR emission intensity increases with the order ITb–Yb < ICe–Yb < ICe–Tb–Yb, which is consistent with the results of the energy transfer efficiency. Meanwhile, the NIR emission integrated intensity of Yb3+ ion in tri-doped Ce3+–Tb3+–Yb3+ system is 1.4 times of the co-doped Ce3+–Yb3+ sample and 23 times as much as that of the co-doped Tb3+–Yb3+ sample, which confirms the highly efficient excitation and subsequent Ce3+ → Yb3+, Tb3+ → Yb3+ and Ce3+ → Tb3+ → Yb3+ possible ET processes in the tri-doped Ce3+–Tb3+–Yb3+ material. Results reveal that the introduction of Ce3+ as a sensitizer with a large absorption cross-section could efficiently turn the adverse high energy photons in the near ultraviolet region into favorable low energy NIR photons effectively absorbed by silicon solar cells. The Ce3+–Tb3+–Yb3+ tri-doped sample could be a potential DC solar spectral convertor by reducing the energy loss to improve the photoelectric conversion efficiency of silicon solar cells.
 |
| Fig. 7 The NIR emission spectra of (a) Ba2Y(BO3)2Cl:0.03Tb3+, 0.20Yb3+; (b) Ba2Y(BO3)2Cl:0.03Ce3+, 0.20Yb3+and (c) Ba2Y(BO3)2Cl:0.03Ce3+, 0.03Tb3+, 0.20Yb3+ phosphors. | |
4. Conclusions
In summary, steady and dynamic photoluminescent properties and the energy transfer mechanism in the Ce3+–Tb3+–xYb3+ tri-doped Ba2Y(BO3)2Cl phosphor were systematically investigated, and four possible ET processes Ce3+ → Tb3+, Ce3+ → Yb3+, Tb3+ → Yb3+ and Ce3+ → Tb3+ → Yb3+ were proposed. As a comparison, the Ce3+/Tb3+–xYb3+ co-doped Ba2Y(BO3)2Cl were also studied. It is found that the energy transfer efficiency of Ce3+ → xYb3+ and Tb3+ → xYb3+ in the tri-doped system is larger than that of the double-doped system. The NIR emission intensity of Yb3+ in a tri-doped sample under excitation of 355 nm is 1.4 times of Ce3+–Yb3+ co-doped sample and is 23 times of Tb3+–Yb3+ co-doped phosphors with optimal composition. Moreover, Ce3+ ion significantly enlarges the absorption cross-section in the near ultraviolet region (320–390 nm) due to the allowed 4f → 5d transition and greatly enhances the NIR emission of Yb3+ ion where the c-Si solar cell exhibits the greatest spectral response. Results indicate that Ba2Y(BO3)2Cl:Ce3+, Tb3+, Yb3+ phosphor is a promising spectral convertor for enhancing photoelectric conversion efficiency of silicon solar cells.
Acknowledgements
This work was supported by the high-level talent project of Northwest University, National Natural Science Foundation of China (no. 11274251), Ph.D. Programs Foundation of Ministry of Education of China (20136101110017), Technology Foundation for Selected Overseas Chinese Scholar, Ministry of Personnel of China (excellent), Natural Science Foundation of Shaanxi Province (no. 2014JM1004) and Foundation of Key Laboratory of Photoelectric Technology in Shaanxi Province (12JS094).
References
- O. Morton, Nature, 2006, 443, 19–22 CrossRef CAS PubMed.
- A. Kraft, C. Wolf, J. Bartsch, M. Glatthaar and S. Glunz, Sol. Energy Mater. Sol. Cells, 2015, 136, 25–31 CrossRef CAS PubMed.
- B. M. van der Ende, L. Aarts and A. Meijerink, Phys. Chem. Chem. Phys., 2009, 11, 11081–11095 RSC.
- B. S. Richards, Sol. Energy Mater. Sol. Cells, 2006, 90, 1189–1207 CrossRef CAS PubMed.
- W. Shockley and H. J. Queisser, J. Appl. Phys., 1961, 32, 510–519 CrossRef CAS PubMed.
- M. B. Spitzer and H. P. Jenssen, Sol. Energy Mater. Sol. Cells, 2013, 108, 241–245 CrossRef CAS PubMed.
- D. Q. Chen, Y. S. Wang and M. C. Hong, Nano Energy, 2012, 1, 73–90 CrossRef CAS PubMed.
- L. Zhao, L. L. Han and Y. H. Wang, Opt. Mater. Express, 2014, 4, 1456–1464 CrossRef CAS.
- T. Trupke, M. A. Green and P. Würfel, J. Appl. Phys., 2002, 92, 1647–1668 CrossRef PubMed.
- W. J. Zhu, D. Q. Chen, L. Lei, J. Xu and Y. S. Wang, Nanoscale, 2014, 6, 10500–10504 RSC.
- Q. Y. Zhang, G. F. Yang and Z. H. Jiang, Appl. Phys. Lett., 2007, 91, 051903 CrossRef PubMed.
- V. D. Rodríguez, V. K. Tikhomirov, J. Méndez-Ramos, A. C. Yanes and V. V. Moshchalkov, Sol. Energy Mater. Sol. Cells, 2014, 122, 46–50 CrossRef PubMed.
- K. Deng, T. Gong, L. Hu, X. Wei, Y. Chen and M. Yin, Opt. Express, 2011, 19, 1749–1754 CrossRef CAS PubMed.
- H. Jing, C. F. Guo, G. G. Zhang, X. Y. Su, Z. Yang and J. H. Jeong, J. Mater. Chem., 2012, 22, 13612–13618 RSC.
- X. Y. Huang, D. C. Yu and Q. Y. Zhang, J. Appl. Phys., 2009, 106, 113521 CrossRef PubMed.
- H. Zhang, X. Y. Liu, F. Y. Zhao, L. H. Zhang, Y. F. Zhang and H. Guo, Opt. Mater., 2012, 34, 1034–1036 CrossRef CAS PubMed.
- S. Li, Z. Hou, Z. Cheng, H. Lian, P. Ma, C. Li and J. Lin, RSC Adv., 2013, 3, 5491–5497 RSC.
- N. M. Zhang, C. F. Guo, H. Jing and J. H. Jeong, Spectrochim. Acta, Part A, 2013, 116, 556–561 CrossRef CAS PubMed.
- W. J. Schipper and G. Blasse, J. Alloys Compd., 1994, 203, 267–269 CrossRef CAS.
- Q. Zhang, J. Wang, G. Zhang and Q. Su, J. Mater. Chem., 2009, 19, 7088–7092 RSC.
- Q. Y. Zhang and X. Y. Huang, Prog. Mater. Sci., 2010, 55, 353–427 CrossRef CAS PubMed.
- D. Geng, G. Li, M. Shang, C. Peng, Y. Zhang, Z. Cheng and J. Lin, Dalton Trans., 2012, 41, 3078–3086 RSC.
- Y. Li, J. Wang, W. Zhou, G. Zhang, Y. Chen and Q. Su, Appl. Phys. Express, 2013, 6, 082301 CrossRef.
- X. Liu, Y. Teng, Y. Zhuang, J. Xie, Y. Qrao, G. Dong, D. Chen and J. Qiu, Opt. Lett., 2009, 34, 3565–3567 CrossRef CAS PubMed.
- P. Vergeer, T. J. H. Vlugt, M. H. F. Kox, M. I. Den Hertog, J. P. J. M. Van der Eerden and A. Meijerink, Phys. Rev. B: Condens. Matter Mater. Phys., 2005, 71, 014119 CrossRef.
- R. Yu, S. Zhong, N. Xue, H. Li and H. Ma, Dalton Trans., 2014, 43, 10969–10976 RSC.
- J. D. Chen, H. Guo, Z. Q. Li, H. Zhang and Y. X. Zhuang, Opt. Mater., 2010, 32, 998–1001 CrossRef CAS PubMed.
- H. Lin, S. Zhou, H. Teng, Y. Li, W. Li, X. Hou and T. Jia, J. Appl. Phys., 2010, 107, 043107 CrossRef PubMed.
- J. Ueda and S. Tanabe, J. Appl. Phys., 2009, 106, 043101 CrossRef PubMed.
- E. van der Kolk, O. M. Ten Kate, J. W. Wiegman, D. Biner and K. W. Krämer, Opt. Mater., 2011, 33, 1024 CrossRef CAS PubMed.
- D. W. Cooke, R. E. Muenchausen, B. L. Bennett, K. J. McClellan and A. M. Portis, J. Lumin., 1998, 79, 185–190 CrossRef CAS.
- Y. Zhu, Z. Sun, Z. Yin, H. Song, W. Xu, Y. Wang, L. Zhang and H. Zhang, Dalton Trans., 2013, 42, 8049–8057 RSC.
- C. F. Guo, H. Jing and T. Li, RSC Adv., 2012, 2, 2119–2122 RSC.
- L. L. Han, Y. H. Wang, Y. Z. Wang, J. Zhang and Y. Tao, J. Alloys Compd., 2013, 551, 485–489 CrossRef CAS PubMed.
- I. A. A. Terra, L. J. Borrero-González, T. R. Figueredo, J. M. P. Almeida, A. C. Hernandes, L. A. O. Nunes and O. L. Malta, J. Lumin., 2012, 132, 1678–1682 CrossRef CAS PubMed.
|
This journal is © The Royal Society of Chemistry 2015 |