DOI:
10.1039/B413048K
(Paper)
New J. Chem., 2005,
29, 205-209
Structure and medium effects on hydraphile synthetic ion channel toxicity to the bacterium E. coli
Received (in Montpellier, France) 23rd August 2004, Accepted 18th October 2004
First published on 6th December 2004
Abstract
Synthetic hydraphile channels were systematically varied at the side arm position and tested under neutral and acidic conditions against the Gram-negative bacterium E. coli. Side arm structure is a critical determinant of channel activity within the bilayer and greatly influences toxicity. The biological activity data correlate remarkably well with the results of Na+ transport studies conducted using synthetic liposome systems. Experiments to determine minimum inhibitory concentrations (MIC) were conducted under both neutral and acidic conditions. Hydraphile toxicity was diminished at pH = 5.5, compared to that at pH = ∼7, providing an example of a pH-dependent, synthetic, non-peptide ion channel.
Introduction
The number and variety of synthetic ion channels has increased dramatically since the pioneering work of Tabushi et al. in the early 1980s.1 Recent reviews record the development of several novel channels or mimics that arise from quite different conceptual approaches.2 Synthetic ion channels have typically, although not always, been characterized in terms of their ion transport function. Synthetic ion channels that mediate ion movement could be, in principle at least, toxic to cells. Toxic effects, if observed, would probably result from insertion of the synthetic channel into the plasma membrane followed by rapid, unregulated ion transport through it. Ion gradients are very closely regulated in even the most primitive organisms. The disturbance of ion gradients would cause osmotic stress in the organism, and disruption would ultimately cause cell death. Indeed, the toxicity of several natural ionophores, including valinomycin,3 the cecropins,4 gramicidin5 and melittin,6 is thought to result from an ion gradient disruption mechanism. We anticipated that these hydraphile compounds would behave as do the natural peptides noted above. Our confidence was bolstered by the fact that the hydraphiles have been extensively characterized in planar bilayer conductance experiments,7 and very recently by whole cell patch clamp experiments.8Relatively few of the interesting synthetic channel compounds developed during recent years have been characterized in phospholipid bilayers. Notable among those for which bilayer conductance data have been reported are channels developed in the groups of Kobuke,9 Fyles,10 Ghadiri,11 Reusch,12 Voyer,13 Matile,14 Koert,15 Woolley16 and in our own lab.17 Characterization of further biological activity inherent in these synthetic channels has been carried out only recently. Biological data have been reported for Voyer’s peptide-linked crown ethers,18 Ghadiri’s stacked cyclic peptide nanotubes19 and our synthetic hydraphiles.20 Biological activity has been reported in the form of red blood cell hemolysis,21 antimicrobial activity for the peptide nanotube22 and for the hydraphile channels.23 In the latter studies, we demonstrated that the hydraphiles exhibit toxicity to E. coli, the magnitude of which depends on the channel’s spacer chains and overall length. We now show that the toxicity of hydraphiles to E. coli is also influenced by side arm structure and by the environmental pH.
Results and discussion
Compounds used in this study
Hydraphiles are structures typically comprised of three diaza-18-crown-6 units that are separated by two identical oligomethylene spacers. The two distal crowns act both as head groups in the amphiphilic sense24 and as portals for ion conduction. The central macrocycle is an ion relay25 that serves the same purpose as the recently discovered “water- and ion-filled capsule” identified in the solid state structure of the KcsA channel of Streptomyces lividans.26 The hydraphile compounds used in this study, 1–5, possess a central diaza-18-crown-6 “central relay unit” flanked by dodecamethylene [(CH2)12] spacer chains. The spacers are connected to the two distal headgroup macrocycles. These distal 18-crown-6 residues are aza-18-crown-6 in 1 but are diaza-18-crown-6 residues in 2–5. In 5, the distal macrocycles are connected by a second identical spacer-crown-spacer fragment.The side arm in each compound plays a significant role in determining the ion transport ability of the hydraphiles. The compounds we have studied most extensively are 3 and 4, which have, respectively, benzyl and dodecyl side arms.27 Structural and fluorescence studies28 indicate that the hydraphiles arrange in the bilayer with the distal macrocycles at opposite ends of the membrane’s insulator regime and we presume that the dodecyl side chains of 4 align with the phospholipids’ fatty acid chains.28 Compound 1 is unique in this group in that it possesses neither a side chain nor a protonatable nitrogen atom. Compounds 2–5 all have at least three diaza-18-crown-6 residues; 5 contains four.
Ion transport in liposomes
Compound 1 showed no detectable sodium transport activity using the Riddell 23Na NMR assay29 that is conducted in liposomes.27 In these same studies, compounds 2, 3 and 4 had relative rates of 28, 39 and 28, respectively, compared to the transport activity of gramicidin (= 100) determined simultaneously. In compound 5, the dodecyl side chains of 4 are linked by a fourth diaza-18-crown-6, which comprises an additional central relay unit. This compound is the most active hydraphile tested to date, with a relative rate of approximately 87 compared to gramicidin.30 The sodium transport results indicate that an N–H is the minimum side arm necessary for activity, while other groups can alter function resulting in highly active channels such as 5.Biological assay
Compounds 1–5 were tested against E. coli to assay the importance of the side arm for bactericidal activity. A suspension of E. coli DH5α cells with ampicillin (AMP) resistance was grown at 37 °C in Luria Bertani (Miller) broth media (100 µg ml−1 AMP). The AMP-resistant strain of E. coli is vital in this growth medium, while the antibiotic suppresses the development of other organisms. In typical experiments, 10 flasks were inoculated with these bacteria (standard inoculum size of 5 × 105 CFU ml−131). Each flask was injected with a sample of the hydraphile. Two-fold serial dilutions normally began at a high concentration of ∼180 µM. Growth in each flask was monitored for 24 h at 37 °C. The minimum inhibitory concentration (MIC) for each hydraphile was determined by direct visual observation. In a series of 2-fold dilutions in flasks or tubes containing bacteria, vitality will be observed as opacity in the aqueous phase. The cutoff value (MIC value) is determined by observation of the point at which too little bacterial growth is apparent to make the suspension opaque. The MIC is reported here as the lowest serial 2-fold dilution that prevented bacterial growth as outlined by the National Committee for Clinical Laboratory Standards (NCCLS).32 Each compound was assayed three times at every reported concentration, using an independent dilution of compound for each experiment. The results are recorded in Table 1 along with published MIC values for valinomycin and melittin. Note that we independently obtained a MIC value for valinomycin in our cell system of >60 µM. To put these values in perspective, note that the published MIC for the common antibiotic penicillin is ∼8 µM. The data obtained in these studies are summarized in Table 1.
Table 1 MICa values to E. coli for hydraphile channel compounds and natural ionophores
| Distal macrocycle | MIC/µM | Na+ transport rated |
---|
pH 5.5 | pH 6.0 | pH 7.1 |
---|
Minimum inhibitory concentration. See ref. 37. See ref. 38. Established from 23Na NMR data from ref. 27, 1995. Value added for comparison, calculated from experimental data that used a different standard. |
---|
1 | Aza-18-crown-6 | — | — | >175 | <2 |
2 | Diaza-18-crown-6 | >175 | 87 | 22 | 28 |
3 | N-Benzyldiaza-18-crown-6 | 75 | 38 | 4.7 | 39 |
4 | N-Dodecyldiaza-18-crown-6 | 0.53 | 0.53 | 2.1 | 28 |
5 | (CH2)12
〈N18N〉 (CH2)12 | 7.9 | 1.8 | 0.99 | 87e |
Valinomycin | — | — | — | >100b | — |
Melittin | — | — | — | ∼18c | — |
Structure-activity relationship
The MIC determined for 1 at pH = 7.1 is 175 µM. We consider such a high concentration value to indicate that 1 is (essentially) biologically inactive. This lack of activity corresponds nicely with the very low sodium ion transport data measured previously in a synthetic liposome system.27 Replacing the 13-O of 1 by N–H affords 2, which shows an ∼8-fold increase in activity (MIC = 22 µM). Compound 3, the “benzyl channel,” is similar in structure except that benzyl groups replace the hydrogens at the N position in the distal macrocycles of 2. This structural change (from 2 to 3) affords another activity increase, this time by about five-fold (MIC = 4.7 µM). When the tris(macrocycle) framework is N-substituted by 12-carbon side chains (4), activity increases again by about two-fold to 2.1 µM. Linking the dodecyl side chains through an additional macrocycle (5) leads to another two-fold enhancement in activity, to a MIC of ∼1 µM. Overall, the structural changes from 1 to 5 lead to an activity increase of nearly 200-fold.It is interesting to compare the toxicity of these compounds to E. coli with the Na+ transport rates that have been measured in synthetic liposomes. As noted above, we anticipated that those compounds that transport cations most effectively through a bilayer would be most toxic. Thus, a high MIC value is expected to correlate with a low ion transport rate, and vice versa. Such a correlation would certainly not prove the mechanism of toxicity for 1–5, but it would be in concert with such a hypothesis. The Na+ transport rates determined in liposomes by 23Na NMR methods are shown in the right hand column of Table 1. The MIC values and transport rates are plotted in Fig. 1.
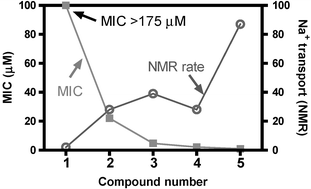 |
| Fig. 1 Comparison of MIC (E. coli, in µM) vs. Na+ transport rate, determined by NMR in liposomes for 1–5. | |
The minimum bactericidal concentration (MBC) was also determined for 2–5 and found to be twice the respective MIC values for these compounds. Thus, benzyl channel 3 inhibits growth at 5 µM and kills all of the DH5α cells at a concentration of 10 µM. This agrees with our previously reported23 IC50 value of 10 µM, which was determined using the classic colony counting technique.23 Taken together, these data indicate that N–H, and not O, is the minimum “side arm” necessary for the hydraphile to be active in the present context. The most toxic compounds result from adding a hydrophobic anchor chain at this position. The aliphatic 12-carbon side chain increases the activity so that 4 is toxic at a MIC of 2.1 µM. The most biologically active compound, 5, is also the most active sodium transporter as judged by the NMR-liposome assay.
Assay of efficacy for control compounds
A mixture of N-benzyl-4,13-diaza-18-crown-6 (PhCH2〈N18N〉H, 6) and N,N’-didodecyl-4,13-diaza-18-crown-6 [CH3(CH2)11〈N18N〉(CH2)11CH3, 7] containing the two structural elements present in benzyl channel 3, but not covalently linked, was considered. A stoichiometric 2∶1 mixture of 6 and 7 was inactive at concentrations of 7 as high as 327 µM. This is equivalent to a concentration of benzyl channel (3) that is nearly 100-fold higher than observed when the separate elements are assembled. 6 was also tested alone in order to separately assay the toxicity of the channel head group/entry portal unit. It was found to be inactive at concentrations as high as 668 µM. It is also interesting to note that the corresponding compound of 3, in which the central macrocycle (central relay unit) is replaced by a 4,4′-dioxabiphenyl unit33 [i.e., PhCH2〈N18N〉 (CH2)12–OPhPhO–(CH2)12〈N18N〉CH2Ph] is inactive at a concentration as high as 170 µM.Dependence of the biological activity on pH
Data obtained by 23Na NMR studies suggested that hydraphile channels were less active at lower pH than at neutral pH. It seems reasonable that protonation of any macrocycle nitrogen atom through which a cation must pass should hinder the transport process simply as a result of charge-charge repulsion. Although the six pKA values for channels 2–5 have not been determined, we have measured the pKA values for N,N′-dibenzyl-4,13-diaza-18-crown-6.27 The relevant data for the studies reported here are as follows: dibenzyldiaza-18-crown-6 (PhCH2〈N18N〉CH2Ph), pK1 = 7.5 and pK2 = 6.83. These pKA values suggest that 3 will be partially protonated even at physiologic pH. Dibutyldiaza-18-crown-6 (Bu 〈N18N〉Bu) was studied as an analog for aliphatic compounds such as 4 and 5. The protonation constants for Bu 〈N18N〉Bu are pK1 = 9.40, pK2 = 7.97. The pKA is the pH at which the respective nitrogen is 50% protonated. Thus, 3 is expected to be less extensively protonated than either 4 or 5 at any acidic pH.The effect of pH on biological activity was assayed using E. coli grown in Luria Bertani (LB) Miller broth media (normally pH = 7.1). Lower pH values were achieved by adding an appropriate amount of 10 mM malonic acid to the buffered broth. In this way, the effect of solution pH values of 5.5, 6.0 and 7.1 could be studied. In all cases, pH values were confirmed by measurements with a pH electrode. As shown in Table 1 (see above), the toxicity of the hydraphiles used in this study depended significantly on medium pH. Fig. 2 plots the data presented in Table 1 for compounds 2–5. The two maximal values of MCI >175, for 2 at pH = 5.5 and 3 at pH = 7.1, are represented as 175 µM, although the MIC was indeterminately higher than that. In any case, such a high value means that the compound is inactive.
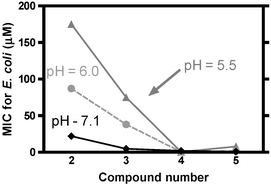 |
| Fig. 2 Dependence of MIC on the medium pH for compounds 2–5. | |
The activities (toxicity, MIC values) are large when the compounds are benign and low when toxicity is high. Fig. 2 clearly shows the trend in activity as a function of pH. Compounds 2, 3 and 5 show a consistent reduction in MIC values (higher toxicity) as the pH is increased from 5.5 to 7.1. The trend is different for aliphatic channel 4, although it is generally a more potent toxin, so the pH-related differences are small. The apparent anomaly in the latter result implies that structure-activity control of toxic behavior may be possible in this family of compounds, in environments of varying acidity. The general trend is clearly that hydraphile toxicity is significantly reduced under acidic conditions.
Fluorescence studies
Our present structural studies, together with the whole cell patch clamp studies we accomplished previously,8 strongly suggest a channel-mediated mechanism for cell death. Nevertheless, fluorescence-based depolarization experiments were undertaken to confirm that hydraphile compounds do indeed permeate the E. coli inner cell membrane. The membrane dye diSC3(5) (3,3′-dipropylthiadicarbocyanine iodide) was utilized in a fashion similar to that described in previous reports22,34 to demonstrate ion transport activity. In short, membranes that have an interior, negative membrane potential readily absorb this dye. When so absorbed, the dye self-quenches and little or no fluorescence is observed. Membrane depolarization is thus detected as an increase in fluorescence intensity as the channel mediates transport between the cells and external medium. Fig. 3 shows that hydraphiles 2–4 cause a rapid depolarization of the E. coli membrane at concentrations equal to the MBC (see Fig. 3 and caption). Similar behavior was noted for 5 at its MBC (data not shown). Control studies using dibenzyldiaza-18-crown-6 did not show appreciable changes in fluorescence and hence do not transport ions across the bacterial membrane. Valinomycin depolarized the membrane when present at a concentration of 9 µM, as shown in Fig. 3. Taken together, these structural and fluorescence studies demonstrate that hydraphiles function as ion transporters in the bilayer of E. coli.
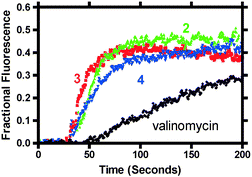 |
| Fig. 3 Percent diSC3(5) dye release for compounds 2 (22 µM), 3 (9.4 µM) and 4 (4.2 µM) and valinomycin (9 µM). Each compound was added after ∼60 s and membrane depolarization was essentially complete within 30 s after that. | |
Effects on biological activity of pH changes
Extensive characterization studies have shown that the two distal diazacrowns in the tris(macrocycle) channels serve as headgroups in the amphiphilic sense. They also serve as entry portals, although whether cations pass through the rings or by them is unknown. A study of channels related to benzyl channel 3 showed that sodium cation transport followed Hammett-type dependence.35 This suggests that ion transport will be even more sensitive to the protonation state of nitrogen in the distal macrocycles. Indeed, protonation of the macroring nitrogen atoms will have at least three effects. First, the presence of one or two positive charges will repel sodium cations that must pass through the channel to enter the cellular cytoplasm. Second, protonated nitrogen can support the formation of a network of water molecules that hydrogen bond to each other and to the macrocyclic rings. Such an organized assembly would either require to be broken up or surpassed by transient cations. Either process would be energetically less favorable than passage of the cations through a less organized, non-protonated headgroup structure. A third possible effect is that protonation would stabilize the position of the channel in the bacterial (in the present case) membrane. This could be either advantageous or deleterious to ion transport, depending on whether or not the low energy conformation was conducting.The effect of changes in pH will be felt not only by the channel structures but by the bacteria. Ghadiri, Granja, and their coworkers conducted important studies of the effect of synthetic peptide nanotube channels against E. coli, methicillin-resistant Staphylococcus aureus, and red blood cells. Twenty-five different peptide sequences were analyzed and it was found that, generally, activity increased when neutral amino acid side chains were substituted by lysine(s) and decreased when replaced by aspartic or glutamic acids. Lysine contains a basic amino group that is protonated at physiologic pH. Thus, replacement of a neutral residue with lysine increases positive charge. Likewise, aspartic or glutamic acids are ionized at pH 7.4, making them anionic residues. The authors state specifically that “the reduced activity of the peptide that contains glutamic acid is probably due to unfavourable electrostatic interactions of the carboxylate side chain with the bacterial membrane constituents. Increasing the number of basic residues from two to three […] yields high activities against MRSA, with (two lysine-containing) sequences […] exhibiting moderate activities against E. coli.”
It seems likely that the results presented here reflect the influence of pH on both the bacteria and on the channels that insert in their membranes. The hydraphile compounds used in this study all possess at least six basic nitrogen atoms and yet their activity diminishes with pH. The contrast may result from the fact that nitrogen is within the putative pore in the hydraphile case and a side chain residue with the nanotube-forming peptide case. It is striking, however, that transport rates determined by an NMR method in synthetic liposomes correlate (inversely) so well with the observed toxicity. Further, the pH effect is consistent in trend, although variable in magnitude, across a range of hydraphile structures.
Conclusion
The data presented here demonstrate that hydraphile synthetic channels exhibit significant toxicity to the Gram-negative bacterium E. coli. In particular, hydraphiles having hydrophobic side chains are very toxic to E. coli. Sodium transport rates have previously been determined by NMR methods in liposomes for these compounds. There is an excellent correlation between high sodium ion transport and high toxicity to bacteria. The most active compound against the bacterium is also the most active sodium ion transporter studied to date. The biological activity of these compounds is also pH dependent. Increasing the acidity of the medium from 7.1 to 6.0 and then to pH 5.5 has a deleterious effect on hydraphile toxicity and on sodium ion transport, as judged by preliminary NMR studies. The experiments presented here are, to our knowledge, the first example of a pH-regulated, synthetic, non-peptidic ion channel.Experimental
The hydraphile channels used in this study were previously reported. Descriptions of their syntheses may be found in ref. 27 for 1–4 and ref. 8 for 5. In all cases, new samples of the known compounds had melting points (where applicable) and 1H- and 13C NMR spectra that corresponded to the structures and to the published data.Assay of antimicrobial activity
The MIC for each hydraphile is reported as the lowest serial 2-fold dilution that prevented bacterial growth as outlined by the NCCLS.32E. coli DH5α cells with a pBluescript plasmid having AMP resistance were tested using the standard inoculum size of 5 × 105 CFU ml−1.31 Cells were grown at 37 °C in 2 ml of Luria Bertani Miller media (10 g L−1 peptone, 5 g L−1 yeast extract, 10 g L−1 NaCl, 100 µg ml−1 ampicillin) that were 2-fold serially diluted with hydraphile test compound. The MIC specified in the text was the lowest hydraphile concentration that inhibited growth after 24 h as judged by visual turbidity. Each compound was assayed three times at every reported concentration, using an independent dilution of compound for each experiment. The MBC was determined as the lowest macrodilution that killed >99.9% of bacteria in the culture. This was determined by plating aliquots of the test suspensions onto petri dishes and counting the number of colony forming units after overnight growth.Fluorescence membrane depolarization studies
3,3′-Dipropylthiadicarbocyanine iodide was added (0.5 µM final concentration) to a buffer solution (0.5 mM HEPES, 200 mM dextrose, pH = 7.5) followed by E. coli DH5α cells that were centrifuged, washed, and resuspended in the same buffer (final OD approx. 0.05). The fluorescence was monitored in real time using a Perkin–Elmer Model LS 50B fluorescence spectrophotometer, at an excitation wavelength of 640 nm, and an emission wavelength of 670. Fluorescence intensity was allowed to stabilize, KCl was added (60 mM final), and the intensity was allowed to stabilize again. Addition of KCl alone does not affect membrane potential, in accord with previous studies.36 Finally, the desired compound was added at an appropriate concentration (typically MBC concentration) and changes in fluorescence monitored. Total dye release was achieved by using Triton X-100 at a final concentration of 5 mM. The solution was vigorously mixed throughout the experiment.Acknowledgements
We thank the NIH for a grant (GM 36262) that supported this work and a Training Grant (T32-GM08492-11) that supported WML.References
- I. Tabushi, Y. Kuroda and K. Yokota, Tetrahedron Lett., 1982, 23, 4601–4604 CrossRef CAS.
-
(a) G. W. Gokel and A. Mukhopadhyay, Chem. Soc. Rev., 2001, 30, 274–286 RSC;
(b) G. W. Gokel, Cell Biochem. Biophys., 2001, 35, 211–231 Search PubMed;
(c) N. Sakai and S. Matile, Chem. Commun., 2003, 2514–2523 RSC;
(d) G. W. Gokel, P. H. Schlesinger, N. K. Djedovic, R. Ferdani, E. C. Harder, J. Hu, W. M. Leevy, J. Pajewska, R. Pajewski and M. E. Weber, Bioorg. Med. Chem., 2004, 12, 1291–1304 CrossRef CAS.
-
(a) M. A. Andersson, R. Mikkola, R. M. Kroppenstedt, F. A. Rainey, J. Peltola, J. Helin, K. Sivonen and M. S. Salkinoja-Salonen, Appl. Environ. Microbiol., 1998, 64, 4767–4773 CAS;
(b) K. A. Mereish, R. Solow, Y. Singh and R. Bhatnager, Med. Sci. Res., 1989, 17, 869–871 Search PubMed.
- A. Matanic and C. V. Castilla, Int. J. Antimicrob. Agents, 2004, 23, 382–389 CrossRef.
- H. W. Huang, Novartis Found. Symp.: Gramicidin and Related Ion Channel-Forming Peptides, 1999, 225, 188–206 Search PubMed.
- L. Béven and H. Wròblewski, Res. Microbiol., 1997, 148, 163–175 CrossRef CAS.
- E. Abel, G. E. M. Maguire, E. S. Meadows, O. Murillo, T. Jin and G. W. Gokel, J. Am. Chem. Soc., 1997, 119, 9061–9062 CrossRef CAS.
- W. M. Leevy, J. E. Huettner, R. Pajewski, P. H. Schlesinger and G. W. Gokel, J. Am. Chem. Soc., 2004 DOI:10.1021/ja046626x Search PubMed.
- Y. Kobuke, K. Ueda and M. Sokabe, J. Am. Chem. Soc., 1992, 114, 7618–7822 CrossRef CAS.
- T. M. Fyles, T. D. James and K. C. Kaye, J. Am. Chem. Soc, 1993, 115, 12315–12321 CrossRef.
- M. R. Ghadiri, J. R. Granja and L. K. Buehler, Nature (London), 1994, 369, 301–304 CrossRef CAS.
- S. Das, U. D. Lengweiler, D. Seebach and R. N. Reusch, Proc. Natl. Acad. Sci. USA, 1997, 94, 9075–9079 CrossRef CAS.
- J.-C. Meillon and N. Voyer, Angew. Chem., Int. Ed. Engl., 1997, 36, 967–969 CrossRef CAS.
- N. Sakai, C. Ni, S. M. Bezrukov and S. Matile, Bioorg. Med. Chem. Lett., 1998, 8, 2743–2746 CrossRef CAS.
- A. Schrey, A. Vescovi, A. Knoll, C. Rickert and U. Koert, Angew. Chem., Int. Ed., 2000, 39, 900–902 CrossRef CAS.
- V. Borisenko, D. C. Burns, Z. Zhang and G. A. Woolley, J. Am. Chem. Soc., 2000, 122, 6364–6370 CrossRef CAS.
-
(a) E. Abel, E. S. Meadows, I. Suzuki, T. Jin and G. W. Gokel, Chem. Commun., 1997, 1, 1145–1146 RSC;
(b) E. Abel, G. E. M. Maguire, E. S. Meadows, O. Murillo, T. Jin and G. W. Gokel, J. Am. Chem. Soc., 1997, 119, 9061–9062 CrossRef CAS.
-
(a) N. Voyer, L. Potvin and E. Rousseau, J. Chem. Soc., Perkin Trans. 2, 1997, 8, 1469–1471 RSC;
(b) A. E. Zoeiby, M. Beaumont, E. Dubuc, F. Sanschagrin, N. Voyer and R. C. Levesque, Bioorg. Med. Chem., 2003, 11, 1583–1592 CrossRef CAS.
- M. R. Ghadiri, J. R. Granja and L. K. Buehler, Nature (London), 1994, 369, 301–304 CrossRef CAS.
- G. W. Gokel and A. Mukhopadhyay, Chem. Soc. Rev., 2001, 30, 274–286 RSC.
- Y. R. Vandenburg, B. D. Smith, E. Biron and N. Voyer, Chem. Commun., 2002, 16, 1694–1695 RSC.
- S. Fernandez-Lopez, H.-S. Kim, E. C. Choi, M. Delgado, J. R. Granja, A. Khasanov, K. Kraehenbuehl, G. Long, D. A. Weinberger, K. M. Wilcoxen and M. R. Ghadiri, Nature (London), 2001, 412, 452–455 CrossRef.
- W. M. Leevy, G. M. Donato, R. Ferdani, W. E. Goldman, P. H. Schlesinger and G. W. Gokel, J. Am. Chem. Soc., 2002, 124, 9022–9023 CrossRef CAS.
- S. Muñoz, J. Mallén, A. Nakano, Z. Chen, I. Gay, L. Echegoyen and G. W. Gokel, J. Am. Chem. Soc., 1993, 115, 1705–1711 CrossRef CAS.
- C. L. Murray, H. Shabany and G. W. Gokel, Chem. Commun., 2000, 2371–2372 RSC.
- D. A. Doyle, J. M. Cabral, R. A. Pfuetzner, A. Kuo, J. M. Gulbis, S. L. Cohen, B. T. Chait and R. MacKinnon, Science, 1998, 280, 69–77 CrossRef CAS.
- O. Murillo, S. Watanabe, A. Nakano and G. W. Gokel, J. Am. Chem. Soc., 1995, 117, 7665–7679 CrossRef CAS.
- E. Abel, G. E. M. Maguire, O. Murillo, I. Suzuki, S. L. De Wall and G. W. Gokel, J. Am. Chem. Soc., 1999, 121, 9043–9052 CrossRef CAS.
- F. G. Riddell, S. Arumugam, P. J. Brophy, B. G. Cox, M. C. H. Payne and T. E. Southon, J. Am. Chem. Soc., 1988, 110, 734–738 CrossRef CAS.
- H. Shabany and G. W. Gokel, Chem. Commun., 2000, 2373–2374 RSC.
- CFU = colony forming unit.
- National Committee for Clinical Laboratory Standards. See: Methods for Dilution Antimicrobial Susceptibility Tests for Bacteria that Grow Aerobically, NCCLS, Wayne, PA, USA, 5th edn., 2000, M7-A5 Search PubMed.
- C. L. Murray, H. Shabany and G. W. Gokel, Chem. Commun., 2000, 2371–2372 RSC.
- L. Zhang, M. G. Scott, H. Yan, L. D. Mayer and R. E. W. Hancock, Biochemistry, 2000, 39, 14504–14514 CrossRef CAS.
- O. Murillo, I. Suzuki, E. Abel and G. W. Gokel, J. Am. Chem. Soc., 1996, 118, 7628–7629 CrossRef CAS.
- H. Suzuki, Z. Y. Wang, M. Yamakoshi, M. Kobayashi and T. Nozawa, Anal. Sci., 2003, 19, 1239–1242 CrossRef CAS.
- D. Seshachalam, D. H. Frahm and F. M. Ferraro, Antimicrob. Agents Chemother., 1973, 3, 63–67 CAS.
- J. F. Stocker and J. R. Traynor, J. Appl. Bacteriol., 1986, 61, 383–388 CAS.
|
This journal is © The Royal Society of Chemistry and the Centre National de la Recherche Scientifique 2005 |
Click here to see how this site uses Cookies. View our privacy policy here.