DOI:
10.1039/B209122B
(Highlight)
Analyst, 2003,
128, 2-6
On-line infrared detection in aqueous micro-volume systems
First published on 20th December 2002
Abstract
Mid infrared spectroscopy is a non-destructive technique that can provide detailed information on important, molecule-specific features such as the conformation and functional groups of a large range of compounds. Infrared spectroscopy is now an established and frequently used technique for qualitative analysis, i.e. the identification of chemical constituents in a sample. In addition, its use for quantitative purposes has grown dramatically in recent years. It is important to realise that the analytical problem defines the mode of operation and implementation of the FTIR technique. This Highlight article focuses on the advantages and scope of on-line FTIR detection strategies. However, in common with all techniques, on-line FTIR detection has a number of potential shortcomings, which are also discussed.
Flow cells vs. solvent elimination
The simplest and probably most common analytical problem is to confirm the identity of a chemical compound, often a starting material in a synthesis or the synthesis product itself. Such measurements are made off-line, in a batch-wise mode. Ways of tackling these simple problems have been considered in great detail in many texts, and are not considered in this review. Confirming the identity of several constituents in a sample is a more challenging task that generally requires some kind of separation step before IR measurements can be performed. Chromatographic techniques have been applied for this1–3 and, recently, capillary electrophoresis.4,5 When using liquid chromatography (LC) the various separated fractions can still be measured off-line, but use of a flow cell in an on-line arrangement provides a more elegant solution. Advantages provided by flow cells include instrumental simplicity, low to moderate costs (due to the possibility of producing up to ca. 30 cells in a single batch) and real time spectral information. High liquid flow rates and the presence of non-volatile eluents such as buffers and salts do not generally cause problems in such situations.
However, a great deal of effort has been devoted to eliminating the eluent by applying a semi on-line or off-line approach rather than using a flow cell in an on-line set-up. The obvious reason for eliminating the eluent is that in most cases it absorbs intensely in the IR region, obscuring the absorption of the analytes. The complete and reproducible evaporation of a non-volatile aqueous solvent is not always straightforward, and rather sophisticated elimination approaches are often needed.1 Another important requirement for maximizing sensitivity is to limit the size of the evaporated spots to the minimal size of the available FTIR probe area.3 Nevertheless, the solvent elimination approach has become more popular than use of flow cells, even for combined reversed phase HPLC-FTIR analyses.
Disadvantages mentioned regarding the flow cell arrangement include difficulties in subtracting the eluent’s absorption from the IR signals and in applying elution gradients. A further problem cited is the need to use short optical path lengths, ranging from a few to approximately 50 μm, depending on the solvents employed and spectral regions of interest. Such short optical path lengths inevitably reduce the sensitivity of the analysis, according to Beer’s law. However, when hyphenating FTIR to CE, the required short optical path length can be advantageous since it is fully compatible with the narrow capillaries normally employed and helps prevent excessive dispersion of the separated analyte zones.4
Stopped or dynamic flow
Even if the analytical problem requires no separation step, use of a flow cell may still provide an efficient technical solution if mechanisation of the entire sample handling process is required. In such cases the sample solution can be fed into the system continuously, provided that the sample volume is not a critical issue. Rinse and calibration solutions can be introduced in a similar manner. If, on the other hand, the sample volume is small, a flow injection or sequential injection (SI) approach might be considered, using a suitable liquid carrier for the injected sample plug. In cases like this, provided that the sample dispersion is negligible, the acquisition of the IR spectrum can then be performed on a still sample, i.e. when the carrier/sample stream is stopped.6 When using SI systems, advantage can be taken of multiple, highly reproducible dispersion profiles in order to prepare suitable standards for establishing a chemometric calibration model (PLS). For this, a sequence of pure standards is injected simultaneously and, due to their interlaced dispersion profiles, several different calibration standards can be obtained during a single run.7 On the other hand, the sample dispersion inside the flow cell can be exploited for fast and reproducible monitoring of diffusion-based chemical reactions using the stopped flow technique.8
The absence of a separation step does not preclude identification and quantification of several analytes in a single sample. For instance, the introduction of multivariate regression methods has enabled the simultaneous quantification of several compounds in wine9,10 and the on-line monitoring of acetone–butanol fermentation processes.11 In addition, commercial FTIR instruments are now available from Foss (Hillerød, Denmark) for the routine, quantitative determination of various constituents in milk and wine (Milkoscan and Winescan, respectively).12 These instruments represent state-of-the-art FTIR detection of still, aqueous samples residing in micro-volume flow cells. Up to 12 components can be determined in milk in less than 45 s. Other applications of these instruments include the determination of constituents in fermented dairy products, soft and hard cheese, whey, fruit juice and honey.12
Hyphenating FTIR to a dynamic flow or separation system gives several advantages. For instance, use of a second-order system avoids the need for full knowledge of all interfering compounds during calibration. However, the IR absorption of most solvents and materials must still be overcome. The development of an appropriate flow cell that can simultaneously fulfil the specific demands posed by both the spectrometer and the separation technique is maybe the most challenging task in on-line hyphenation.
Flow cells for on-line hyphenation
Strategies used in the development of flow cells are mainly based upon either the transmission or the total reflection of IR light.
A. Transmission flow cells
It is not always necessary to develop a new, micro-machined flow cell. Instead, reversed phase HPLC and standard, commercially available, transmission flow cells have been used in some cases. For instance, Vonach et al. used a transmission flow cell, available from Perkin-Elmer, consisting of two IR-transparent CaF2 windows (transparent above 1100 cm−1) and a polymer spacer, yielding a flow cell with an optical path length of 25 μm and an inner cell volume of 10 μL. With this system carbohydrates, alcohols and organic acids in wine could be identified and measured with an absolute LOD of 4 μg.13 The cited authors believe that downscaling to microbore HPLC could further decrease this LOD level. De la Guardia and co-workers used a micro flow through cell with ZnSe windows and a lead spacer of ∼50 μm for the determination of cholesterol in animal grease14 and recently, caffeine in tea samples.15 The use of such a long optical path length was made possible by selecting a relatively IR-transparent mobile phase consisting of chloroform and acetonitrile (45∶55). However, deuterated solvents can also be considered to allow longer optical paths and to avoid spectral overlap between the eluent and analytes. Such solvents are expensive, but their cost may be justified when using micro-bore columns.16 Different on-line extraction methods have also been applied in order to circumvent the problems associated with water and IR-opacity.17
The flow cell incorporated in the instruments from Foss consists of two CaF2 disks bracketing a spacer with a rhomboid-shaped void, providing a flow channel with an optical path length of 37 μm (Milkoscan) or 47 μm (Winescan). The calibrations offered from the company are based on analyte-characteristic parts of the IR spectrum and a multivariate approach.
We have recently reported the successful on-line hyphenation of capillary electrophoresis (CE) with FTIR detection and attained LOD levels in the nano- to picogram range for samples injected into the capillary.4 The main obstacle when realising this hyphenation was the total IR absorption of the fused silica capillaries, requiring the development and incorporation of an IR-transparent CE-flow cell. The choice of starting material fell on CaF2 since it is transparent in the IR fingerprint region, it can be micromachined and it can withstand the aqueous buffers commonly used in CE separations. Further, it is non-conductive so it is not influenced by the high voltages applied during CE separations. Consequently, two CaF2 disks were used as starting material, on one of which a titanium layer was deposited by evaporation. This layer functioned as an optical aperture and was isolated from the separation buffer. By applying two streaks of SU-8 epoxy-based photo resist to each disk a 150 μm wide and 15 μm thick flow channel was formed. The area outside the flow channel was filled with UV-curing epoxy adhesive. The two disks were bonded together by cross-linking the SU-8 during a hard-baking process (200 °C, 1 h), giving the flow cell the chemical resistance and mechanical strength required. Using this technique, 40 flow cells could be constructed in a single batch on one occasion. Fig. 1 presents a schematic illustration of this flow cell. Formation of a small O-ring at the end of the sharply cut capillaries assured tight connection between the capillaries and the flow cell.
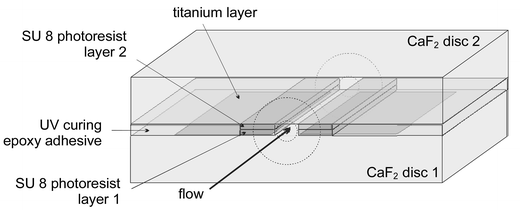 |
| Fig. 1 Schematic illustration of the CE flow cell we developed (reproduced with permission from the American Chemical Society). | |
B. ATR flow cells
Another approach is to use a flow cell based on attenuated total reflection (ATR), where the optical path length is dependent on the number of reflections, so very short path lengths can be achieved. One example of such a cell is the CIRCLE (cylindrical internal reflectance) cell,18,19 consisting of a cylindrical ZnSe crystal with cone-shaped ends mounted in a stainless steel cell. The solution to be analysed bathes the exposed area of the ZnSe crystal. The IR beam is focused on the entrance cone and reflected several times (typically 10) along the crystal. The resulting light is then collected and guided towards the detector. In these ATR-based flow cells only a small fraction of the available cell volume is probed by the IR light. If an increase in optical path length is desired the cells become excessively large. Louden and co-workers used a micro CIRCLE flow cell with a 25 μL cell volume for the multiple on-line hyphenation of reversed phase HPLC to UV-MS-FTIR-NMR using deuterated superheated D2O as mobile phase for the on-line analysis and characterisation of ecdysteroids in plant extracts20 and model pharmaceuticals.21 Dunn and co-workers integrated a mixing unit into a micro CIRCLE cell. This arrangement combines the advantages of time-resolved FTIR spectra and larger flow channels as the light bounces off the solution instead of going through it, as in transmission flow cells. Hence, potential problems such as cell clogging and high backpressure are avoided.6
Developments in mid infrared technology
A. Data acquisition rate vs. interferometers
The remarkable success of conventional Fourier transform interferometers is attributable to their high sensitivity and acquisition speed. Nevertheless, some measurements cannot be performed with these conventional interferometers, such as monitoring fast and irreproducible reactions or rapidly moving analytes in a separation flow system. Thus, when using a conventional interferometer in conjunction with CE separation, the speed of the separation will impose restrictions on the scanning velocity of the interferometer. This velocity is, in turn, governed by the desired number of co-additions of scans per spectrum and the spectral resolution. The residence time for an analyte is, under optimised CE separation conditions, typically 1–5 s thereby limiting the time available for data acquisition. Under such conditions there must be a trade-off between accumulating large numbers of scans to improve the signal-to-noise ratio and the spectral resolution between subsequent spectra. In a further study of the influence of scanning velocity, expressed as the Fourier frequency of the HeNe laser used to determine the optical path difference, we concluded that an increase in the scanner velocity from 80 kHz to 200 kHz only reduced the time required for the acquisition of 50 co-additions, with a spectral resolution of 16 cm−1, from 3.5 s to 2.2 s.4 This indicates that the time-consuming step between consecutive scans was the reversal of the moving mirror in the Michelson interferometer involved. Developments towards ultra-rapid scanning interferometers are therefore interesting in this context. Because of the difficulties involved in maintaining the alignment of a rapidly moving mirror, built-in optical tilt compensation is desirable. Manning and Griffiths22,23 proposed a tilt-compensated interferometer where the optical path difference can be generated by either tilting or translating one of the interferometer mirrors. The mirror rotates at a maximum rate of 500 kHz, producing two interferograms per revolution and, thus, 1000 scans per second.
B. Quantum Cascade lasers
Quantum Cascade (QC) lasers, invented in 1994 by Faist et al.,24 may provide a technical solution to the problem of combining aqueous solutions and mid-infrared detection systems. QC lasers are unipolar lasers based on inter-subband transitions, i.e. transitions between quantized energy states within one energy band of a semiconductor. The key for laser action, population inversion, is achieved by careful band-structure engineering and the designed structures are grown by molecule beam epitaxy (MBE). A characteristic feature of these lasers is their cascade structure, in which an electron normally passes 20–30 active regions (and up to 100 in tests25), in each of which other electrons are stimulated to emit light. Hence, emission powers in the milliwatt range can be attained in pulsed-mode at room temperature. Further, unlike the process in other semi-conducting laser sources, the wavelength is determined by quantum confinement, i.e. the thickness of the active layer rather than by the energy band-gap of the material. By tailoring the thickness of the active layer the QC laser emission can be tuned over a wide range (3–12 μm) using the same material.26 By exchanging the light source normally used in mid-infrared spectroscopy for a QC laser, the available optical path length and sensitivity can be increased. Further, the use of a QC laser instead of an interferometer results in real-time spectral data acquisition since no parts of the instruments are moving, and there is no need for mathematical calculations.
QC lasers have already been used successfully in the spectroscopic detection of trace gases.26 Their potential use as detectors for condensed phases has been demonstrated recently,27 in a study where the LOD of phosphates in soft drinks was improved 50 fold compared with the sensitivity obtained with state-of-the-art Fourier transform infrared spectrometers. In another assessment of these lasers it was shown that by using two different Fabry Pérot QC lasers, one emitting at 1080 cm−1 and the other at 1650 cm−1, i.e. overlapping with the strong O–H bending of water, the available optical path length could be increased 5 fold while still allowing functional group-specific detection of the amide I band (1650 cm−1).28 Edelmann et al. used a QC laser emitting in the water window at 1067 cm−1 hyphenated to a reversed phase HPLC system for the on-line separation and functional group-specific detection of sugars in red wine.29 Since no other constituents in red wine but the sugars absorbed at the available laser emission band, there was no need for the chromatography to be fully optimised or for all peaks to be resolved. On the contrary, the detection of partly resolved analytes was no problem.
Research in QC lasers is an expanding field and recently Gmachl et al.30 demonstrated a QC laser containing two substacks, each with a previously optimised QC structure. This resulted in a QC laser emitting simultaneously at two different wavelengths, 5.2 μm and 8.0 μm, with performance levels equal to those of the respective homogenous QC lasers. Further, by inserting an etch-stop layer, a tap, between the two substacks, the laser threshold could be manipulated, allowing the 8.0 μm laser to be turned on and off while the adjacent 5.2 μm QC laser was left undisturbed.
Developments in CE-IR hyphenation
The separation mechanism in normal capillary electrophoresis (CZE) is based on differences in analyte migration in an electric field. This is, in principle, the simplest mode of CE since the capillary is filled solely with buffer. Separation of both anionic and cationic analytes is possible by CZE and applications include the analysis of inorganic ions, amino acids, peptides, a wide range of enantiomers and numerous other ionic species.31–33 We strongly believe that IR detection can improve various types of CE, either in its FT mode or by utilising QC lasers as light sources. One example that has been described recently,5 is the on-line hyphenation of FTIR to micellar electrokinetic chromatography (MEKC), a hybrid of electrophoresis and chromatography introduced by Terabe in 1984.34 This hyphenation illustrates the potential ease and quality of this technique. Since the added micelles distribute themselves evenly throughout the capillary, stable infrared reference spectra can be recorded. Of course, increasing the amount of infrared-absorbing compounds in the buffer raises the limit of detection of FTIR systems, but still LOD values in the millimolar range have been obtained, i.e. the low nanogram range in absolute amounts. Fig. 2 shows an on-line MEKC-FTIR separation of five investigated analytes: caffeine, paracetamol, p-nitrobenzyl alcohol, m- and p-nitrophenol. As shown in the figure, all the analytes can be detected with some spectral information for structural determination—whether or not this information is sufficient depends on the application in question.
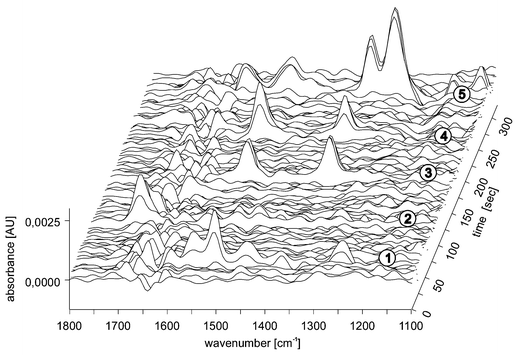 |
| Fig. 2 On-line FTIR detection of five analytes (1, paracetamol; 2, caffeine; 3, p-nitrobenzyl alcohol; 4, m-nitrophenol and 5, p-nitrophenol) separated by MEKC. Applied voltage, 250 V cm−1; capillary inner diameter, 50 μm; length to the detection point, 40 cm; electrolyte, 15 mM phosphate buffer at pH 7.0 and 40 mM SDS. The scanner velocity of the spectrometer was 150 kHz and for each spectrum 50 co-additions were made with a spectral resolution of 16 cm−1 (reproduced with permission from Wiley). | |
Conclusion
In summary, two fundamentally different IR detection approaches are available for the identification and quantification of several analytes in a sample, (i) acquisition of individual analyte IR spectra after a prior separation step (applied either on-line or off-line) or (ii) acquisition of a direct IR spectrum of the sample, without prior separation, combined with chemometrics. So what is the motive for using FTIR detection in micro-volume flow systems? In the case of routine analysis of milk and wine the answer is quite obvious: the information provided by the IR spectral data is analytically easier to interpret than corresponding information obtained in the UV-visible and near infrared spectral regions. In the case of LC-FTIR no obvious advantages are attained that would render currently existing detection techniques second choice.1 Finally, with respect to the CE-FTIR combination, it has three obvious inherent strengths: extremely small sample volumes are needed, the separation ability of CE is extraordinarily high, and the structural information and capacity for identifying sample constituents provided by IR is outstanding. The applications reported so far promise the successful separation and identification of a wide range of analytes in picogram quantities in the future.
An interesting point has been reached in the use of infrared detectors with aqueous solutions, where fruitful cooperation between diverse research fields is providing technical advances such as new interferometer models, QC lasers emitting at several emission bands and innovative flow cell designs that allow substantial numbers of them (up to ca. 30) to be produced in a single batch. The authors conclude that developments in the power and productivity of these systems will continue apace.
References
- G. W. Somsen, C. Gooijer and U. A. T. Brinkman, J. Chromatogr., A, 1999, 856, 213 CrossRef CAS.
- R. Schindler and B. Lendl, Anal. Commun., 1999, 36, 123 RSC.
- P. R. Griffiths, S. L. Pentoney, A. Giorgetti and K. H. Shafer, Anal. Chem., 1986, 58, 1349A CAS.
- M. Kölhed, P. Hinsmann, P. Svasek, J. Frank, B. Karlberg and B. Lendl, Anal. Chem., 2002, 74, 3843 CrossRef.
- M. Kölhed, P. Hinsmann, B. Lendl and B. Karlberg, Electrophoresis, in press Search PubMed.
- B. C. Dunn, J. R. Marda and E. M. Eyring, Appl. Spectrosc., 2002, 56, 751 Search PubMed.
- R. Schindler, M. Watkins, R. Vonach, B. Lendl, R. Kellner and R. Sara, Anal. Chem., 1998, 70, 226 CrossRef CAS.
- P. Hinsmann, M. Haberkorn, J. Frank, P. Svasek, M. Harasek and B. Lendl, Appl. Spectrosc., 2001, 55, 241 Search PubMed.
- R. Schindler, R. Vonach, B. Lendl and R. Kellner, Fresenius’ J. Anal. Chem., 1998, 362, 130 CrossRef.
- C. D. Patz, A. David, K. Thente, P. Kurbel and H. Dietrich, Wien- Wissenshaft, 1999, 54, 80 Search PubMed.
- M. Kansiz, J. R. Gapes, D. McNaughton, B. Lendl and K. C. Schuster, Anal. Chim. Acta, 2001, 438, 175 CrossRef CAS.
-
http://www.foss.dk
.
- R. Vonach, B. Lendl and R. Kellner, J. Chromatogr., A, 1998, 824, 159 CrossRef CAS.
- Y. Daghbouche, S. Garrigues and M. de la Guardia, Anal. Chim. Acta, 1997, 354, 97 CrossRef CAS.
- J. Ohnsmann, G. Quintas, S. Garrigues and M. de la Guardia, Anal. Bioanal. Chem., 2002, 374, 561 Search PubMed.
- K. Jinno, C. Fujimoto and G. Vematsu, Am. Lab., 1984, 16, 39 Search PubMed.
- G. W. Somsen, E. W. J. Hooijschuur, C. Gooijer, U. A. T. Brinkman, N. H. Velthorst and T. Visser, Anal. Chem., 1996, 68, 746 CrossRef CAS.
- M. Sabo, J. Gross, J. S. Wang and I. E. Rosenberg, Anal. Chem., 1985, 57, 1822 CrossRef CAS.
- R. S. Brown and L. T. Taylor, Anal. Chem., 1983, 55, 1492 CAS.
- D. Louden, A. Handley, R. Lafont, S. Taylor, I. Sinclair, E. Lenz, T. Orton and I. D. Wilson, Anal. Chem., 2002, 74, 288 CrossRef CAS.
- D. Louden, A. Handley, S. Taylor, I. Sinclair, E. Lenz and K. D. Wilson, Analyst, 2001, 126, 1625 RSC.
- P. R. Griffiths, B. L. Hirsche and C. J. Manning, Vib. Spectrosc., 1999, 19, 165 CrossRef CAS.
- C. J. Manning, AIP Conference Proceedings, 1998, 430, 84 Search PubMed.
- J. Faist, F. Capasso, D. l. Sivco, C. Sirtori, A. L. Hutchinson and A. Y. Cho, Science, 1994, 264, 553.
- F. Capasso, C. Gmachl, R. Paiella, A. Tredicucci, A. L. Hutchinson, D. L. Sivco, J. N. Baillargeon, A. Y. Cho and H. C. Liu, IEEE J. Sel. Top. Quantum Electron., 2000, 6, 931 CrossRef CAS.
- C. Gmachl, F. Capasso, D. L. Sivco and A. Y. Cho, Rep. Prog. Phys., 2001, 64, 1533 CrossRef CAS.
- B. Lendl, J. Frank, R. Schindler, A. Muller, M. Beck and J. Faist, Anal. Chem., 2000, 72, 1645 CrossRef CAS.
- M. Kölhed, M. Haberkorn, V. Pustogov, B. Mizaikoff, J. Frank, B. Karlberg and B. Lendl, Vib. Spectrosc., 2002, 29, 283 CrossRef CAS.
- A. Edelmann, C. Ruzicka, J. Frank, B. Lendl, W. Schrenk, E. Gornik and G. Strasser, J. Chromatogr., A, 2001, 934, 123 CrossRef CAS.
- C. Gmachl, D. L. Sivco, J. N. Baillargeon, A. L. Hutchinson, F. Capasso and A. Y. Cho, Appl. Phys. Lett., 2001, 79, 572 CrossRef CAS.
- R. A. Frazier, Electrophoresis, 2001, 22, 4197 CrossRef CAS.
-
D. Heiger, High Performance Capillary Electrophoresis–An Introduction, Agilent Technologies, 2000 Search PubMed.
- W. Thormann, I. S. Lurie, B. McCord, U. Marti, B. Cenni and N. Malik, Electrophoresis, 2001, 22, 4216 CrossRef CAS.
- S. Terabe, K. Otsuka, K. Ichikawa, A. Tsuchiya and T. Ando, Anal. Chem., 1984, 56, 111 CrossRef CAS.
|
This journal is © The Royal Society of Chemistry 2003 |
Click here to see how this site uses Cookies. View our privacy policy here.