Capabilities of a homogenized 266 nm Nd:YAG laser ablation system for LA-ICP-MS
Received
30th August 2001
, Accepted 18th October 2001
First published on 20th November 2001
Abstract
A 266 nm Nd:YAG laser ablation system equipped with homogenizer optics to convert any type of laser beam profile into a “flat-top” laser profile is described. The influences of the homogenizer arrays on energy distribution across the laser beam and the energy density on the ablation characteristics in relation to signal intensity measured by ICP-MS were investigated. Energy density and related ablation rate for various materials were studied and figures of merit are summarized. It is shown that beam homogenization influences the ablation rate and the ablation characteristics in general. Highly transparent samples, such as quartz, can be ablated at 266 nm Nd:YAG and the homogenized beam leads to less cracking of the material (uncontrolled if insufficiently high energy density is applied to the sample surface). The effect of reduced ablation rate per pulse of a homogenized laser beam in comparison to a Gaussian beam profile has an influence
on elemental fractionation, which is shown by the quantification of the well-characterized T1-G glass sample from the MPJ-DING glass series.
Introduction
The development of laser ablation inductively coupled plasma mass spectrometry (LA-ICP-MS) as a solid sampling technique has been widely described.1–4 From the early experiments with IR lasers,5 the development moved quickly towards the use of shorter wavelengths and UV lasers. Shorter wavelengths improved the laser–sample interaction primarily for transparent samples, such as quartz or fluorite. Several types of lasers with different wavelengths are now in use depending on application and budget. Today's most widespread used laser ablation systems for ICP-MS or inductively coupled plasma optical emission spectrometry (ICP-OES) are based on Nd:YAG lasers operating at the fourth harmonic at 266 nm in the ultraviolet.
Commercially available Nd:YAG laser ablation systems reach a maximum pulse energy of ∼6 mJ at 266 nm leaving the laser head. Some of this energy is lost in the optical beam delivery path especially if an aperture for spot shaping is used. In this case an increasing part of the energy is lost using smaller apertures to obtain small ablation craters. An alternative is the use of a beam compressor lens system, which maximizes the energy extraction through the aperture for each spot size. This leads to higher energy densities and towards smaller crater sizes on the sample, but is not suitable for obtaining a constant energy density over the most commonly used range of crater diameters (5–300 µm). The influence of the wavelength on elemental fractionation, as defined in ref. 6, has been studied in detail.7–9 Elemental fractionation is still
seen as one of the drawbacks of this technique,10–15 even if only a limited number of elements are affected.
Mank and Mason reported a significant influence of the crater depth-to-diameter ratio on elemental fractionation during the ablation process,16 which as a result requires (in order to visualize this effect) a constant sample uptake rate independent of the crater diameter. In comparison to the more “flat-top” beam profiles of excimer lasers, the Gaussian beam profile of a Nd:YAG laser makes it more difficult to ensure a homogeneous illumination of the laser beam onto the sample surface. The advantage of an ArF 193 nm excimer laser ablation system, equipped with homogenizing optics, has been demonstrated in ref. 17. This system allows the adjustment of the crater size between 4 and 120 µm, while maintaining constant energy densities of up to 40 J cm−2, and has been successfully applied to a number of applications.18,19
A comparison of various wavelengths (157, 248, 213 and 266 nm), as reported in refs. 7 and 8, indicated that the wavelength has less influence on elemental fractionation. In contrast, a comparison between a 266 nm Nd:YAG and a 193 nm excimer laser ablation system20 indicated significantly reduced elemental fractionation using the shorter wavelength, which allows the conclusion that the optical system used has an influence on the ablation process. Due to the low acceptance of excimer lasers, partially based on price, size and the use of a fluorine-containing gas, the improvement of the ablation characteristics of the most used 266 nm Nd:YAG laser are of great interest. Jeffries et al.21 showed that a low energy quintupled Nd:YAG (213 nm) laser ablation system improved the ablation characteristics and
reduced elemental fractionation, in comparison to a quadrupled Nd:YAG (266 nm) system. However, the energy density of such a 213 nm system seems to be at the low end of the optimized conditions necessary to ablate the whole range of opaque and transparent samples.
This work describes the design of a high power 266 nm Nd:YAG laser ablation system equipped with homogenizing optics. The new optical system of the 266 nm Nd:YAG laser ablation system is partially adopted from that of the excimer laser system described in ref. 17. Results obtained concerning beam homogeneity, ablation rate, signal stability, reproducibility and preliminary results on elemental fractionation are reported. A well-characterized reference standard MPJ-DING22 was used to compare the quantitative results achievable using the new laser ablation system.
Experimental and instrumentation
A frequency quadrupled Nd:YAG laser (Brilliant B, Quantel, Les Ulis, France) with a wavelength of 266 nm was used to build a high power, homogenized 266 nm Nd:YAG laser ablation system. The maximum output energy of the laser is 70 mJ per pulse at 10 Hz with a beam size of 0.8 × 0.5 mm, a pulse width of 5.9 ns at 1064 nm and a pulse-to-pulse stability of better than 10% at 70 mJ. The laser repetition rate can be varied from 2 to 20 Hz.
A schematic diagram of the beam path is shown in Fig. 1. After the initial beam is expanded to illuminate a larger area of the first lens array, two mirrors separate the remaining part of the frequency doubled green laser light (532 nm). The beam profile, produced by limited quality of the second harmonic and fourth harmonic generator crystals, is split up by a first lens array (9 × 9 lenses, 3.5 mm aperture, Limo, Dortmund, Germany) and is intercepted by a second similar array. The condenser lens after the second lens array collimates the light to a homogeneous square field (the dimensions of which can be varied) after the field lens. The size of the illuminated field is controlled by the distance between the alongside the beam movable mounted first lens array and the fixed-mounted second lens array. The illuminated square field can be varied between 3.5 × 3.5 mm
and 10 × 10 mm.
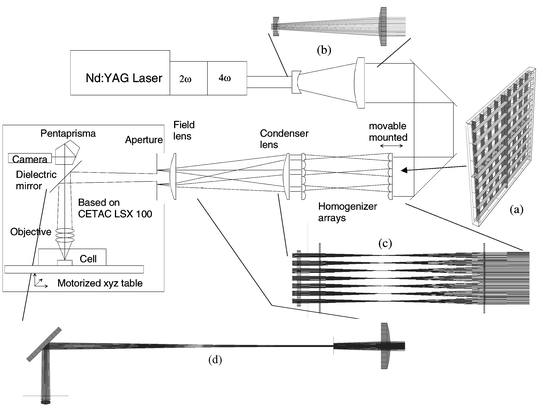 |
| Fig. 1
Schematic view of the newly developed laser ablation system: (a–d), ray tracing results for parts of the beam path.
| |
The field lens is needed to adjust the position of the focal point of the array, which otherwise could damage the optical elements following the aperture. The aperture wheel consists of round holes from 3 to 0.1 mm diameter, to obtain crater diameters between 300 to 10 µm using a 5× objective, or between 150 to 5 µm using a 10× objective. The mirror following the aperture steers the beam onto the objective, which generates the image of the aperture on the sample. The properties of the objectives used are summarized in Table 1. The 10× objective provides significantly higher energy densities together with a smaller minimum spot size in comparison to the 5× objective. However, if the application does not require high spatial resolution but requires lower energy densities, the 5× objective can be an advantage. All optical elements are anti-reflection coated and mounted on an optical rail.
Table 1
Properties of the objectives used
S. D. Acquisition (Omaha, USA)
OFR (Caldwell, USA)
|
Magnification |
5× |
10× |
Supplier |
CETACa |
OFRb |
Number of elements |
3 |
5 |
Working distance |
40 mm |
20 mm |
UV transmittance |
80% |
50% |
AR-coating |
All elements |
All elements |
Demagnification ratio |
10 |
20 |
Energy density range |
3.5–15 J cm−2 |
10.5–45 J cm−2 |
Overall energy density can be varied from 3.5 to 45 J cm−2 by adjusting the lens array distance together with the selection of the tested objectives. Fig. 2 shows the energy density on the sample depending on the array distance and the selection of the objective. The overfilling of the second lens array determines the maximum distance between the arrays (22 cm), while the risk of damaging the second array by the focal point of the first lens array determines the minimum distance (20 cm). It is possible to obtain lower energy densities than 3.5 J cm−2 by using a beam splitter or by reducing the flash lamp voltage. However, the drawback of reducing the flash lamp voltage is a loss of pulse-to-pulse stability from the laser. Owing to the imaging of the aperture, the energy density over all crater sizes (10–300 µm) is constant within
15%. The pulse energies after the cell window were measured with a laser energy meter (Gentec, duo, ED-500, Canada).
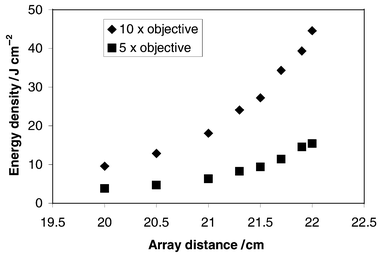 |
| Fig. 2
Influence of lens array distance on energy density on the sample surface.
| |
The front end of the ablation system is based on a CETAC LSX-100. The sample observation optics and the motorized stage are used for sample positioning and ICP-MS optimization. The sample is observable, with a CCD camera on a monitor, through a penta prism above the dielectric mirror, which steers the beam into the objective. Both transmitted and reflected light observation allows sample positioning before and during the ablation process.
The samples were placed in a Plexiglas cell with a volume of 20 cm3. The cell has an inlet through a nozzle (0.5 mm id) and an outlet (4 mm id), and is closed with an air-tight seal using a fused silica anti-reflection coated window (2.0 mm thick). Owing to the similar transport characteristics observed for the different ablation cells,23 the 20 cm3 ablation volume cell was used for all experiments.
The laser-induced aerosols were measured using an Elan 6000 (Perkin-Elmer/SCIEX, Toronto, Canada) ICP-MS. Quantitative determinations of trace elements in all kinds of samples (minerals, glass, steel, polymers) were based on internal standardization using major elements to correct for differences in the ablated mass between the external standards and samples. Therefore, a linear dynamic range of up to 9 orders of magnitude and the capability of changing the mass resolution for individual isotopes were extensively used. Intensities up to 2 × 106 counts s−1 were measured using the pulse counting mode and up to 2 × 109 counts s−1 using the analogue mode. ICP-MS optimization was performed by using the scan mode of the LSX-100 on the reference material, NIST SRM 612 glass, which provided a constant flux of aerosol for several minutes into the ICP.
Operating conditions of the laser and the ICP-MS used for the experiments are summarized in Table 2.
Table 2
Operating conditions used for the laser ablation and ICP-MS system
Depending on the distance between the arrays and the objective used.
|
Laser type |
266 nm Nd:YAG frequency quadrupled (Quantel Brilliant B, Les Ulis, France) |
Homogenization |
2 crossed lens arrays, 9 × 9 lenses, 3.5 mm lens width, 2 mm substrate thickness (Limo, Dortmund, Germany) |
Output energy |
70 mJ at 10 Hz |
Energy densitya |
3.5 J cm−2–45 J cm−2 |
Repetition rate |
2 Hz–20 Hz |
Objectives |
10×, 5× |
Crater size |
20 µm, 40 µm, 70 µm, 100 µm, 150 µm (40 µm, 80 µm, 140 µm, 200 µm, 300 µm) |
Energy distribution |
Flat topped |
Pulse duration |
6 ns |
ICP-MS type |
Elan 6000 (Perkin-Elmer/Sciex, Toronto, Canada) |
Carrier gas flow |
1.3 l min−1 |
Intermediate gas flow |
1.0 l min−1 |
Plasma gas flow |
15.0 l min−1 |
RF power |
1400 W |
Detector mode |
Dual, pulse counting and analogue mode |
Dwell time |
10 ms |
Sampler cone |
Aluminium 0.8 mm orifice |
Settling time |
3 ms |
Isotopes |
7Li, 11B, 23Na, 27Al, 29Si, 39K, 42Ca, 45Sc, 51V, 53Cr, 57Fe, 59Co, 60Ni, 65Cu, 66Zn 69Ga, 72Ge, 75As, 85Rb, 88Sr, 89Y, 90Zr, 95Mo, 107Ag, 114Cd, 115In, 118Sn, 137Ba, 139La, 140Ce, 151Eu, 165Ho, 175Lu, 183W, 208Pb, 209Bi, 232Th, 238U |
Cell volume |
20 cm3 |
External standard |
SRM NIST 612 |
Internal standard |
Calcium |
Repetitions |
5–25 |
Samples |
MP-DING reference glasses |
Ablation duration |
60 s (single hole) |
Results and discussion
The beam profile of the 266 nm Nd:YAG laser (see burn pattern, Fig. 3a) was homogenized using two lens arrays, a condenser lens and a field lens. The beam path from the laser to the sample was ray traced with Optica for Mathematica (Wolfram Research, Inc., IL USA). Details of the beam path are shown in Fig. 1. Each homogenizer array consists of 2 × 9 cylindrical plano-convex lenses building a lens segment of 81 lenses (a). 10 single rays were traced through the beam expander (b) and 100 rays were traced through the two arrays and the condenser lens to determine their performance (c). Finally, a ray-trace of 100 rays through the field lens, aperture and mirror onto the objective is shown in (d). The ray trace of the beam through the objective onto the sample surface was not performed due to missing information about the optical components
within the objective. Fig. 3 shows the burn pattern on temperature sensitive paper of the initial beam profile behind the beam expander (Fig. 3a) and the homogenized square field in front of the aperture (Fig. 3b). In addition, the calculated grids of rays at these positions are shown in Fig. 3c and d. A more precise calculation of the homogeneity of the beam profile onto the aperture (Fig. 3d) was considered. Unfortunately, the computation time increases exponentially with the number of rays traced and was therefore not affordable. However the ray tracing calculations allowed the homogeneous laser beam delivery onto the sample surface to produce craters shown in Fig. 4. The crater images in the quartz and NIST SRM 610 samples illustrate
that the crater bottom is coarsely grained but level. The craters were acquired using a diameter of 150 µm (100 pulses), at a fluence of 45 J cm−2.
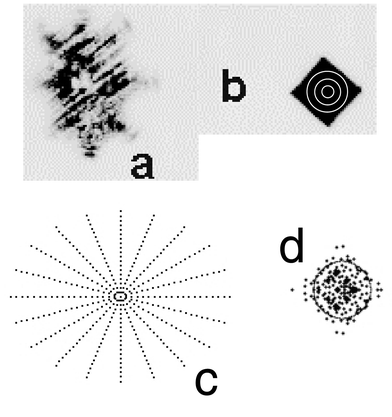 |
| Fig. 3
Comparison of the energy distribution of the initial laser beam (a) and the homogenized beam at aperture position (b) on thermo-sensitive paper together with the corresponding ray tracing results (c, d). The position of the aperture is for 3 different aperture sizes shown in (b).
| |
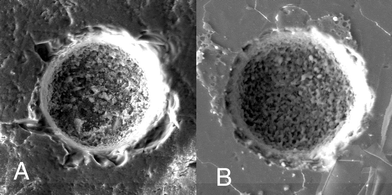 |
| Fig. 4
SEM pictures of craters in quartz (A) and NIST 610 Glass (B). Both craters were acquired using a diameter of 150 µm, 100 laser pulses and an energy density of 45 J cm−2.
| |
The imaging optics allowed the adjustment of energy densities from 3.5 to 45 J cm−2 with corresponding pulse energies of 0.5–6 mJ on the sample surface, as shown in Fig. 5. The homogeneous square field in front of the aperture is partly imaged (holes of 3 mm id maximum) onto the sample surface leading to crater sizes of 300 to 10 µm with a constant energy density on the sample surface. An energy density of 45 J cm−2 is sufficient to ablate the transparent samples, as shown on a natural quartz sample (Fig. 4A). The crater profile was measured using a Dektrak 8000 surface profilometer (Fig. 6). Unfortunately, the conical shaped crater profile measured using the centre line scan is also based on the shape of the profilometer needle and does not fully represent
the crater geometry. However, the crater profile measured indicates an almost flat crater bottom (within 10–15%) until a depth-to-diameter ratio of at least 1∶1 is reached. The ablation rates for different materials are summarized in Table 3. It has been shown that the ablation rate is matrix dependent and highly variable between metals and glass samples.24 Furthermore, in comparison to the other NIST 600 glasses, the more highly absorbing NIST SRM 610 led to the lowest sample ablation rate per pulse. This explains the wavelength and output energy dependence on penetration depth, as previously discussed in refs. 7 and 24. Figs. 7 and 8 demonstrate the linear dependence of the sample removal (nm per pulse) on the number of pulses and on the energy density (10–35 J cm−2).
In addition, the effect of the energy density on sensitivity was measured and is shown in Fig. 9. It can be seen, that the signal intensities (counts s−1 µg g−1) at a constant crater size for various elements are almost linearly dependent on the energy density. The deviation of the signal intensity between the lowest energy density and the highest energy density experiment is caused by the difference in the depth of ablation per pulse. A trend of increasing elemental ratios with increasing energy density, e.g., Pb/U (0.38 ± 0.3, min. 0.33 at 7 J cm−2 and max. 0.41 at 40 J cm−2) was observed, which can be partly explained by a change in the aspect ratios (diameter-to-depth16) towards higher fluency. However, measured ratios using five different
energy densities of fractionating and non-fractionating element ratios varied between 3%
(Eu/Tb, Eu/Th, Pb/Th) and 10%
(Th/U, Pb/U). The relatively low sensitivity, in comparison to earlier reported work from our group using a 193 nm excimer laser ablation system, depends on the carrier gas, since all experiments were carried out using argon as carrier gas. A significant increase in sensitivity obtained using helium as the carrier gas in conjunction with a 193 nm excimer laser system has not been observed using 266 nm for ablation.20
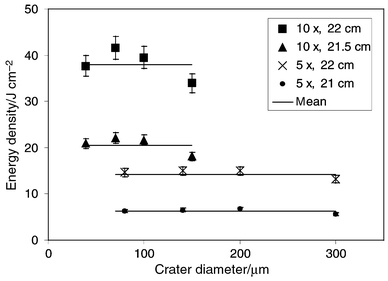 |
| Fig. 5
Calculated energy densities from measured pulse energies and crater area, which were measured after the objective. Energy densities between 5 and 40 J cm−2 are constant for apertures smaller than 3 mm. The relative laser output stability for an integration of 200 pulses is constant within 6%
(error bars). The 15% decrease in energy density for the largest crater diameter for both objectives is due to overfilling of the objective at apertures of 3 mm diameter.
| |
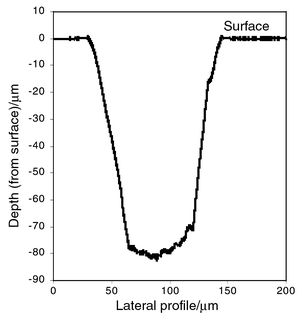 |
| Fig. 6
Depth profile in quartz using an energy density of 45 J cm−2.
| |
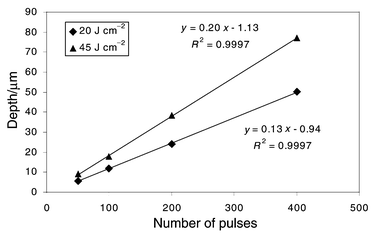 |
| Fig. 7
Illustration of the linearity of the ablation rates vs. depth for copper at two different energy densities. Each data point represents a depth measurement.
| |
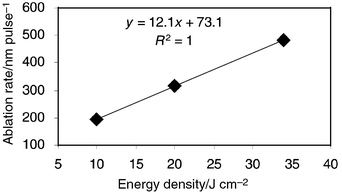 |
| Fig. 8
Ablation rates for borosilicate glass at energy densities between 10–35 J cm−2.
| |
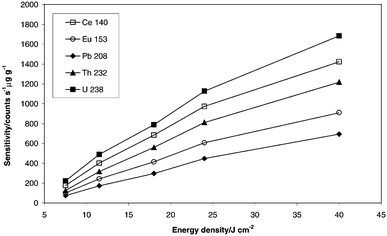 |
| Fig. 9
Correlation between sensitivity and energy density for 70 µm craters at a signal integration time of 70 s.
| |
Table 3
Ablation rate per pulse for different materials (Mean and standard deviation are calculated from 3 replicates)
Material |
Ablation rate/nm pulse−1 |
20 J cm−2 |
35 J cm−2 |
NIST 610 |
380 ± 40 |
490 ± 50 |
NIST 612 |
600 ± 60 |
860 ± 90 |
NIST 614 |
660 ± 70 |
960 ± 90 |
Glass |
300 ± 40 |
500 ± 60 |
Copper |
120 ± 20 |
190 ± 30 |
Signal intensities dependent on the crater diameter were acquired and are shown in Figs. 10 and 11. The average intensities depend almost linearly on the crater area, which is further evidence for an energy density on the sample surface that is independent of crater size. The calculated correlation coefficients are between 0.996 for Al and 0.9991 for Pb. In addition, various ratios such as Al/Ca and U/Pb were calculated for the first 20 s of transient signals, acquired using different crater sizes (40 µm to 150 µm) and medium energy density. It can be seen that the element ratios vary from 10 ± 2.8 to 8.3 ± 1.2 (40 µm to 150 µm) for Al/Ca and from 4 ± 3.6 (counting statistical error: ± 0.82) to 2.2 ± 0.5 (counting statistical error: ± 0.07)
(40 µm
to 150 µm) for U/Pb. The data indicate that elemental fractionation also contributes to the element ratios, especially within the first seconds of ablation. Fig. 12 shows a typical transient signal at a diameter-to-depth ratio of 1∶3 (depth = 300 µm). It can be seen that the signal structure is improved in comparison to previously shown 266 nm Nd:YAG ablation signals. The largest change in element ratios attributed to elemental fractionation is observed in the first 20 s after the start of the ablation (Fig. 13), whereas the remaining signal acquisition leads to stable signals. The changes in the element ratios in the first few seconds are taken as an indication of a variation in the particle size rather than aerosol composition,25 otherwise the elemental ratios in part C (Fig. 13) would not remain as stable. Therefore, it can be assumed that the particle fraction from the first few seconds of the ablation process is dependent on the ablation rate and these particles are only partly ionized in the ICP.8,14,26,27 Assuming that for each crater size the portion of “bigger” particles is equivalent, elemental fractionation will be more pronounced for small craters, which could explain the changes in the elemental ratios (see also Fig. 10).
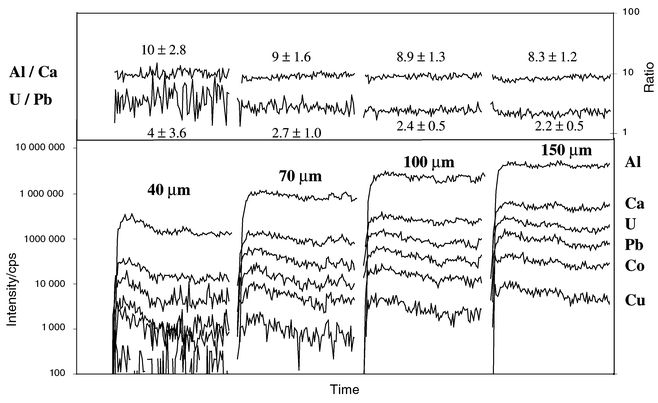 |
| Fig. 10
Time resolved transient signal intervals of 20 s long integration intervals and corresponding elemental ratios for 4 different crater sizes in NIST 612, obtained using an energy density of 20 J cm−2.
| |
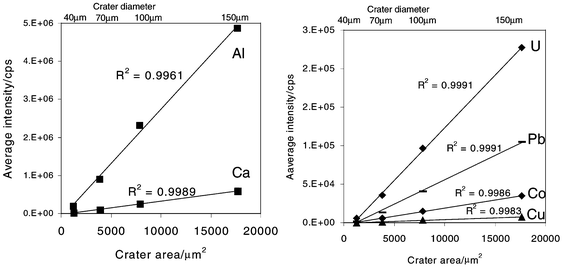 |
| Fig. 11
Transient signals acquired with 4 different crater diameters and 20 J cm−2 and their corresponding elemental ratios (integrated for a constant period of 20 s each) are shown for NIST 612.
| |
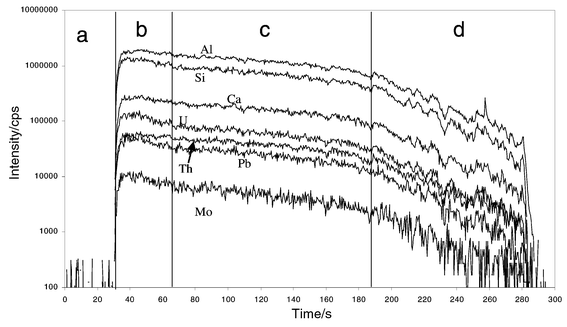 |
| Fig. 12
Background-corrected, time-resolved intensities for NIST 612 (10 Hz, 100 µm, 15 J cm−2). a: background, b: pre-ablation interval, c: signal interval used for quantification and d: depth related signal decrease.
| |
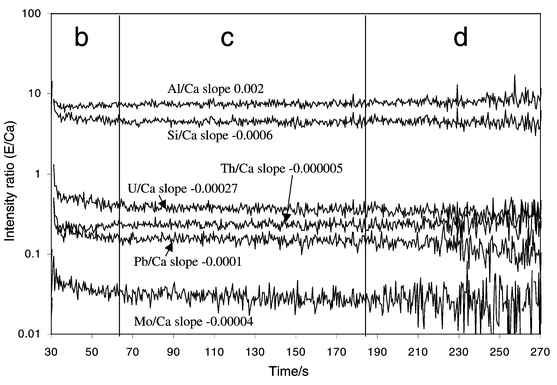 |
| Fig. 13
Intensity ratios (element/Ca) corresponding to the time resolved signals in Fig. 12. Signal interval used for quantification (c) shows no significant slopes for various fractionating and non-fractionating elemental ratios.
| |
Various glass samples (MPJ-DING) were analysed and the results are summarized in Table 4; comparing the reference values given in ref. 22, and data obtained using the LSX 200 266 nm Nd:YAG laser ablation system described in ref. 28. The analyses in our experiments were carried out with different crater diameters and the data were acquired according to the parameters recommended in ref. 29. In contrast to the recommended use of BCR-2G as external reference standard, the reference material SRM NIST 612 was used for quantification of major and trace elements in these samples (T1-G shown in Table 4). Data were acquired within three different days, using ICP-MS conditions that were optimized daily, including
energy densities (15–35 J cm−2) and crater diameters (70–150 µm). The reproducibility for 12 measured elements was 5% or better and, for most others, was better than 15%. The higher RSDs observed for some elements may be due to heterogeneous element distribution or statistical counting limitations. Concentrations for Ge, Ag, Cd, In and Tl are given, which were not reported in ref. 22. Almost all measured elements agree within 10% or less with the reference values, independent of concentration. The deviation of the As and Mo concentrations cannot be explained by interferences or by elemental fractionation, because elements affected by elemental fractionation, such as Pb and Sn, Cu, Ni, agree to within a few percent with data acquired using solution nebulization.22 However, the element concentrations of Pb
and Cu in particular differ significantly from data acquired using a non-homogenized 266 nm Nd:YAG ablation systems28 and non-matrix matched calibrations. Therefore, our non-matrix matched calibration is in agreement even for fractionating elements (Pb, Cu, Ni etc.).27
The signal structure as well as the ablation rate per pulse of the designed 266 nm Nd:YAG ablation system are indications that the use of homogenizer arrays in combination with an output energy above 40 mJ (at 266 nm) improves the ablation characteristics of this wavelength significantly.
Table 4
Trace and major element determination in T1-G (Italian Alps quartz glass) using Ca (5.06 wt%) as internal standard.
Element |
μg g−1 |
This work |
Ref. 22
|
Ref. 28
|
Li |
22 ± 3 |
20 |
— |
B |
4.4 ± 0.8 |
5 |
— |
Na (wt.%) |
2.1 ± 0.3 |
2.33 |
— |
Al (wt.%) |
10 ± 0.4 |
9.00 |
— |
Si (wt.%) |
26 ± 2 |
27.3 |
— |
Ti (wt.%) |
0.45 ± 0.02 |
0.44 |
— |
Sc |
25.2 ± 0.5 |
26.7 |
— |
V |
188 ± 19 |
190 |
— |
Cr |
20 ± 4 |
22 |
21.0 |
Mn |
1000 ± 50 |
1010 |
— |
Co |
20 ± 2 |
19 |
18.2 |
Ni |
11 ± 2 |
13 |
8.62 |
Cu |
18 ± 4 |
21 |
9.9 |
Ga |
19.6 ± 0.7 |
18.6 |
18.9 |
Ge |
2.4 ± 0.5 |
— |
— |
As |
1.6 ± 0.3 |
0.71 |
— |
Rb |
86 ± 6 |
80 |
61.7 |
Sr |
272 ± 6 |
283 |
— |
Y |
21.0 ± 1.1 |
23.2 |
26.6 |
Nb |
9.1 ± 0.7 |
9.1 |
8.4 |
Mo |
1.4 ± 0.3 |
5.4 |
— |
Ag |
0.2 ± 0.06 |
<0.6 |
— |
Cd |
0.5 ± 0.2 |
<30 |
— |
In |
0.5 ± 0.09 |
<0.3 |
— |
Sn |
2.3 ± 0.2 |
2.1 |
11.6 |
Cs |
2.7 ± 0.4 |
2.9 |
2.02 |
Ba |
390 ± 17 |
382 |
345 |
La |
71 ± 2 |
69 |
71.3 |
Ce |
129 ± 6 |
127 |
— |
Eu |
1.17 ± 0.03 |
1.21 |
1.20 |
Tb |
0.79 ± 0.06 |
0.82 |
0.76 |
Ho |
0.86 ± 0.07 |
0.83 |
0.91 |
Tm |
0.38 ± 0.05 |
0.35 |
0.37 |
Lu |
0.36 ± 0.03 |
0.35 |
0.39 |
Ta |
0.49 ± 0.03 |
0.45 |
0.47 |
W |
0.74 ± 0.16 |
0.86 |
— |
Tl |
0.2 ± 0.07 |
— |
— |
Pb |
13.5 ± 1.46 |
13 |
6.35 |
Th |
31 ± 1.5 |
30 |
29.8 |
U |
1.8 ± 0.14 |
1.67 |
1.39 |
Conclusion
The designed laser ablation system equipped with homogenizer optics, used in combination with ICP-MS, shows an alternative strategy to improve the laser energy transfer onto the sample surface for a 266 nm Nd:YAG laser, which leads to a lateral homogeneous distributed energy across the ablation area. This is independent of the crater diameter and allows flexible adjustment of spatial resolution for each individual analysis. It is demonstrated that energy densities, adjustable between 4 and 45 J cm−2, allow the ablation of various opaque and UV-transparent samples. This has been a major limitation for commercially available 266 nm Nd:YAG laser ablation systems. The time-resolved ablation signal structure is significantly improved by using a homogeneous energy density across the beam in comparison to gaussian beam profile laser ablation systems. The effect of elemental fractionation, which previously led to biased data for fractionating elements
such as Pb, Ni and Cu, is reduced, as shown for T1-G. The increase in energy density leads to an increase in ablation rate per pulse, which is crater size-independent and is illustrated by the linear relationship of sensitivity vs. energy density of ≤ 23 J cm−2. The ablation crater shown for quartz indicates that the 266 nm lasers used in LA-ICP-MS need to have sufficient output energy(>40 mJ) to allow beam homogenization and to reach energy densities above the threshold ablation conditions for highly transparent samples. The advantage of beam homogenization using lens arrays is illustrated by successful application of non-matrix matched external calibration. Quantitative data obtained even for elements that suffer from the effects of elemental fractionation are in agreement with reference values.22 This may be due to the particle size distribution of the removed material,
which is part of further investigations.
Acknowledgements
The authors acknowledge the support by ETH Zurich, Swiss National Science Foundation and S.D. Acquisition (Omaha, USA).
References
- S. A. Darke and J. F. Tyson, J. Anal. At. Spectrom., 1993, 8, 145 RSC
.
- S. F. Durrant, J. Anal. At. Spectrom., 1999, 14, 1385 RSC
.
- J. S. Becker and H.-J. Dietze, Int. J. Mass Spectrom., 2000, 197, 1 CrossRef CAS
.
- D. Günther, I. Horn and B. Hattendorf, Fresenius' J. Anal. Chem., 2000, 368, 4 CrossRef CAS
.
- A. L. Gray, Analyst, 1985, 110, 551 RSC
.
- B. J. Fryer, S. E. Jackson and H. P. Longerich, Can. Min. J., 1995, 33, 303 Search PubMed
.
- R. E. Russo, X. L. Mao, O. V. Borisov and H. Liu, J. Anal. At. Spectrom., 2000, 15, 1115 RSC
.
- H. Liu, O. V. Borisov, X. Mao, S. Shuttleworth and R. E. Russo, Appl. Spectrosc., 2000, 54, 1435 Search PubMed
.
- D. Figg and M. S. Kahr, Appl. Spectrosc., 1997, 51, 1185 Search PubMed
.
- M. Motelica-Heino, O. F. X. Donard and J. M. Mermet, J. Anal. At. Spectrom., 1999, 14, 675 RSC
.
- Z. Chen, J. Anal. At. Spectrom., 1999, 14, 1823 RSC
.
- S. M. Eggins, L. P. J. Kinsley and J. M. G. Shelley, Appl. Surf. Sci., 1998, 129, 278 CrossRef
.
- D. Figg, J. B. Cross and C. Brink, Appl. Surf. Sci., 1998, 127, 287 CrossRef
.
- M. E. Taylor, D. L. Blaney and G. Cardell, Appl. Surf. Sci., 2000, 165, 166 CrossRef CAS
.
- T. Hirata, J. Anal. At. Spectrom., 1997, 12, 1337 RSC
.
- A. J. G. Mank and P. R. D. Mason, J. Anal. At. Spectrom., 1999, 14, 1143 RSC
.
- D. Günther, R. Frischknecht, C. A. Heinrich and H.-J. Kahlert, J. Anal. At. Spectrom., 1997, 12, 939 RSC
.
- M. Guillong and D. Günther, Spectrochim. Acta, Part B, 2001, 56, 1219 CrossRef
.
- D. Günther, A. Audetat, R. Frischknecht and C. A. Heinrich, J. Anal. At. Spectrom., 1998, 13, 263 RSC
.
- D. Günther and C. A. Heinrich, J. Anal. At. Spectrom., 1999, 14, 1369 RSC
.
- T. E. Jeffries, S. E. Jackson and H. P. Longerich, J. Anal. At. Spectrom., 1998, 13, 935 RSC
.
- K. P. Jochum, D. B. Dingwell, A. Rocholl, B. Stoll and A. W. Hofmann, Geostand. Newsl., 2000, 24, 87 Search PubMed
.
- D. Bleiner and D. Günther, J. Anal. At. Spectrom., 2001, 16, 1 Search PubMed
.
- I. Horn, M. Guillong and D. Günther, Appl. Surf. Sci., 2001, 7215, 1
.
- D. Günther, D. Bleiner, M. Guillong, B. Hattendorf and I. Horn, Chimia, 2001, 10, 1 Search PubMed
.
- M. L. Alexander, M. R. Smith, J. S. Hartman, A. Mendoza and D. W. Koppenaal, Appl. Surf. Sci., 1998, 127, 255 CrossRef
.
- P. Goodall, S. G. Johnson and E. Wood, Spectrochim. Acta, Part B, 1995, 50, 1823 CrossRef
.
- J. S. Becker, C. Pickhardt and H.-J. Dietze, Mikrochim. Acta, 2000, 135, 71 CrossRef CAS
.
- H. P. Longerich, S. E. Jackson and D. Günther, J. Anal. At. Spectrom., 1996, 11, 899 RSC
.
|
This journal is © The Royal Society of Chemistry 2002 |