DOI:
10.1039/B009571K
(Paper)
CrystEngComm, 2001,
3, 21-26
Morphology of barium sulfate synthesized with barium(II)–aminocarboxylate chelating precursors
Received
29th November 2000
, Accepted 15th January 2001
Abstract
The morphology of barium sulfate (BaSO4), synthesized using the hydrothermal reaction of BaII–aminocarboxylate chelating precursors, was investigated. The precursors ethylenediaminetetraacetic acid (EDTA), bis(2-aminoethyl)ethyleneglycoltetraacetic acid (EGTA) and nitrilotriacetic acid (NTA) were dissolved by heating, resulting in the formation of BaSO4 with rod-like, rhombohedral and spindle-shaped morphologies, respectively. Changing the chelating reagent resulted in the formation of BaSO4 with differing morphologies. There was a correlation between the morphology of BaSO4 and the dissociation constant of the BaII–aminocarboxylic acid complexes. When the dissociation constant was small, BaSO4 formed rhombohedral morphologies, when it was large, spindle-shaped morphologies were formed. N 1s XPS measurements indicated that the aminocarboxylic acid was adsorbed on the surface of the precipitated particles. Comparison of the peak intensity X-ray diffraction patterns of EDTA and EGTA showed growth of the {101} face when EDTA was used.
Introduction
Barium sulfate is a white pigment used in cosmetics and as a filler in papermaking or as a coating material. When synthesizing barium sulfate as a powder, controlling its morphology is very important. However, conventional processes do not control the morphology of sedimentary barium sulfate. There has been a great deal of research on the morphology control of barium sulfate using various additives,1,2 including organic acids3–7 such as alkyldiphosphonates. The morphology changes that occur during the synthesis of barium sulfate with added organic acids seem to be governed by the inhibition of the growth of specific crystal surfaces. This results from either the substitution of an organic acid ion for a sulfate ion or the binding of an organic acid ion to a barium ion.
The interaction between the barite crystal surface and the solution or chelating reagent has also been studied. Molecular model calculations have demonstrated that the crystal growth rate of the barite surfaces differ with the surface energy or attachment energy.8,9 EDTA, a typical chelating agent, dissolves barite effectively by forming BaII–EDTA surface complexes; their desorption results in an increased etch pit formation rate.10 Research on barite dissolution in aqueous diethylenetriaminepentaacetic acid (DTPA) suggested that the active sites of the DTPA molecule bind to Ba2+ cations exposed on the (001) surface.11 Kinetic studies on the dissolution of barium sulfate in aqueous EDTA and DTPA indicate that the surface reaction is the rate-controlling step in the dissolution process and not the mass transfer.12
The synthesis of monodispersed particles of slightly soluble inorganic materials, such as barium sulfate,13,14 metal oxides15 and phosphate salts,16–18 by homogeneous precipitation using the dissociation or decomposition of the metal–EDTA complexes has been reported. Preparation of powder by hydrothermal synthesis uses the metal–aminocarboxylate complex as a precursor. This method can be used to control the morphology, and even occurs at low temperatures. Although the mechanism by which aminocarboxylates regulate the morphology of the powder is not clear, they appear to contribute to the supply of metal ions by the dissociation of metal complex. They also affect the flocculation control of primary particles and the adsorption or binding to specific particle surfaces.
In this study, barium sulfate was synthesized by a hydrothermal method using BaII–aminocarboxylic acid complex as a precursor. The effect of the chelating agent on the morphology of barium sulfate was examined. The effect of the type of aminocarboxylic acid on the morphology of barium sulfate is discussed from two perspectives: (1) dissociation reactions involving the BaII–aminocarboxylic acid complex that influences the supply of Ba2+ ions and (2) adsorption reactions involving the aminocarboxylic acids that affect the growth and flocculation of the BaSO4 crystal.
Experimental
Reagents
Reagent grade barium chloride, sodium sulfate and aminocarboxylate (acb) were used as the starting materials. The aminocarboxylates (Dojindo Co.) used were: nitrilotriacetic acid (NTA), N-(2-hydroxyethyl)iminodiacetic acid (HIDA), N-(2-hydroxyethyl)ethylenediamine-N, N′, N′-triacetic acid (HEDTA), ethylenediamine-N,N, N′, N′-tetraacetic acid, disodium salt, dihydrate (EDTA), propylene-1,2-diamine-N, N, N′, N′-tetraacetic acid (PDTA), 2-hydroxypropylene-1,2-diamine-N, N, N′, N′-tetraacetic acid (HPDTA), trans-1,2-diaminocyclohexane-N, N, N′, N′-tetraacetic acid, monohydrate (CyDTA), O, O′-bis(2-aminoethyl)ethyleneglycol-N, N, N′, N′-tetraacetic acid (EGTA), triethylenetetramine-N, N, N′, N′′, N′′′, N′′′-hexaacetic acid (TTHA).
Synthesis
Ba2+–aminocarboxylate solutions were prepared by mixing 0.16 M aminocarboxylic acid and 0.08 or 0.12 M BaCl2 solution in distilled, deionized water, together with the desired amount of 1 M NaOH solution to adjust the pH to 10. After 0.08 to 0.80 M Na2SO4 solution was added and the pH adjusted to 12, the total concentration of aminocarboxylate, [acb], was 2.56⊕×⊕10−2 M. In this paper, [X] denotes the total molar concentration of compound X. A given quantity (30 cm3) of a mixed solution at the desired concentration was poured into a glass bottle (DURAN®) with an inner volume of 50 cm3. The vessel was then sealed with a screw cap, placed in a thermostatically regulated oven, and heated at 25 to 150
°C for 2 to 12 h. The precipitated powder was then recovered using a membrane filter, washed with distilled, deionized water, and dried in an oven at 105
°C for 2 h under an air atmosphere. The crystalline phase identification was performed by X-ray diffraction (XRD) using nickel-filtered Cu-Kα radiation. The precipitate morphology and size were examined by scanning electron microscopy (SEM). The concentration of Ba2+ in the filtrate was determined by inductively coupled plasma atomic emission spectrometry (ICP-AES).
Results and discussion
Morphology of BaSO4 synthesized with various BaII–aminocarboxylate precursors
All the white precipitates formed were identified by XRD as orthorhombic barium sulfate.19 The conditions used were: 2.56⊕×⊕10−2 M aminocarboxylate, [acb]/[Ba2+] molar ratios from 1.5 to 2, [SO42−]/[acb] molar ratios from 0.5 to 5, and temperatures from 25 to 150
°C.
Fig. 1 shows SEM photographs of barium sulfate particles precipitated using various aminocarboxylates as chelating agents under the following conditions: 2.56⊕×⊕10−2 M aminocarboxylate, [acb]/[Ba2+] molar ratio of 2, [SO42−]/[acb] molar ratios from 0.5 to 5, temperatures of 90 or 150
°C and a time of 2 h. The morphology of the barium sulfate synthesized was rhombohedral using EGTA or TTHA, rod- or needle-shaped using EDTA, PDTA, HPDTA or CyDTA, and spindle-shaped using NTA, HIDA or HPDTA. The morphology changed considerably with different chelating agents.
![SEM photographs showing the different morphology of particles precipitated in the BaII–aminocarboxylate–Na2SO4 solutions after 2 h. The [acb]/[Ba2+] molar ratio was 2. Aminocarboxylates: (a) HIDA; (b) NTA; (c) HPDTA; (d) HEDTA; (e) EDTA; (f) TTHA; (g) EGTA; (h) PDTA; (i) CyDTA. Temperature: (a) 90 °C, (b)–(i) 150 °C. [SO42−]/[acb] molar ratio: (a) 0.1, (c) 0.5, (b),(d),(e),(g)–(i) 2, (f) 4.](/image/article/2001/CE/b009571k/b009571k-f1.gif) |
| Fig. 1
SEM photographs showing the different morphology of particles precipitated in the BaII–aminocarboxylate–Na2SO4 solutions after 2 h. The [acb]/[Ba2+] molar ratio was 2. Aminocarboxylates: (a) HIDA; (b) NTA; (c) HPDTA; (d) HEDTA; (e) EDTA; (f) TTHA; (g) EGTA; (h) PDTA; (i) CyDTA. Temperature: (a) 90 °C, (b)–(i) 150 °C. [SO42−]/[acb] molar ratio: (a) 0.1, (c) 0.5, (b),(d),(e),(g)–(i) 2, (f) 4.
| |
In this study, barium sulfate was synthesized by the hydrothermal method by way of the dissociation of precursor BaII–aminocarboxylate complexes. Equations for the chemical reactions are shown below. Eqn. 1 shows the dissociation of the BaII–aminocarboxylate complex:
|  | (1) |
where
n and
m range from 3 to 6 and 0 to 6, respectively, depending on the pH of the solution and the aminocarboxylate.
Eqn. 2 shows the
proton dissociation reaction of aminocarboxylic acid:
|  | (2) |
Under these conditions all of the
protons dissociated in solution (
m⊕=⊕0). Therefore,
eqn. (1) could be simplified to give
eqn. (3).
|  | (3) |
Eqn. 4 shows the precipitation reaction of barium sulfate:
|  | (4) |
The equation for the formation of barium sulfate
via dissociation of the Ba
II–aminocarboxylate complex [
eqn. (5)] is deduced from
eqns. (3) and (4):
|  | (5) |
The dissociation constants,
Kd, of the Ba
II–aminocarboxylate complexes at 25
![[thin space (1/6-em)]](https://www.rsc.org/images/entities/char_2009.gif)
°C are listed in
Table 1. When spindle-shaped particles were formed, the dissociation constants of complexes of the Ba
2+ ion and aminocarboxylates, such as
NTA, HIDA and HPDTA, were relatively large. On the other hand, rhombohedral or rod-like particles formed when the dissociation constants of complexes of the Ba
2+ ion and aminocarboxylates, such as PDTA, CyDTA, EGTA, TTHA, EDTA and HEDTA, were relatively small.
Table 1
Thermodynamic data used to predict the dissociation constant, Kd, of the BaII–aminocarboxylate complexes
Aminocarboxylate |
–log Kd |
T/K |
I
/mol dm−3 a |
ΔG°298/kJ mol−1 |
ΔS°298/J mol−1 K−1 |
Ref. |
I denotes the ionic strength.
|
HIDA |
3.37 |
298.15 |
0.1 |
19.2 |
−16.7 |
20
|
NTA |
4.8 |
298.15 |
0.1 |
27 |
−71 |
20
|
HPDTA |
4.91 |
298.15 |
0.1 |
28.0 |
– |
21
|
HEDTA |
6.2 |
298.15 |
0.1 |
35 |
−41.8 |
20
|
EDTA |
7.8 |
298.15 |
0.1 |
45 |
−79 |
20
|
TTHA |
8.22 |
303.15 |
0.1 |
36.7 |
– |
20
|
EGTA |
8.3 |
298.15 |
0.1 |
47 |
−33.5 |
20
|
PDTA |
8.53 |
298.15 |
0.1 |
48.7 |
−138 |
20
|
CyDTA |
8.6 |
298.15 |
0.1 |
49 |
−41 |
20
|
The formation or dissociation of a metal ion–carboxylate complex is temperature dependent.22–28 Therefore, the changes in the dissociation constants, Kd, of the BaII–aminocarboxylate complexes with temperature were estimated. The standard Gibbs free energy changes, ΔG°Tr, and the standard entropy changes, ΔS°Tr, of the BaII–aminocarboxylate complexes used for the calculation are listed in Table 1.
The standard Gibbs free energy change, ΔG°T, and the dissociation constant, Kd,T, at T K were calculated from eqns. (6) and (7), respectively.
|  | (6) |
|  | (7) |
Tr denotes the reference temperature, 298.15 K and Δ
Cp° denotes the standard partial molar heat capacity. Owing to the lack of partial molar heat capacity data for Ba
II–aminocarboxylate complexes other than Ba
II–EDTA, the temperature changes were estimated from
eqn. (8), in which the partial molar heat capacity is approximated as 0. The standard Gibbs free energy change for the Ba
II–EDTA complex was calculated from
eqn. (9), in which the molar heat capacity was approximated as being constant
29,30 without temperature dependency.
|  | (8) |
|  | (9) |
The relative differences between the dissociation constants calculated from the approximations of
eqns. (8) and (9) were about 6% at 100
![[thin space (1/6-em)]](https://www.rsc.org/images/entities/char_2009.gif)
°C and 13% at 150
![[thin space (1/6-em)]](https://www.rsc.org/images/entities/char_2009.gif)
°C. The approximation of
eqn. (8) is sufficient for comparing the temperature dependency of the dissociation constants.
The change in the dissociation constants of complexes between NTA, HIDA, HEDTA, EDTA and EGTA and Ba2+ ions with temperature is shown in Fig. 2. Increasing temperature thermodynamically promoted dissociation of the complex.
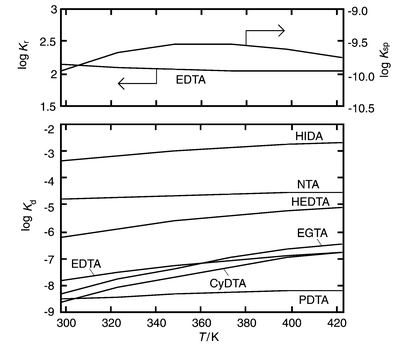 |
| Fig. 2
Temperature dependence of the equilibrium constants for the overall dissociation and precipitation reaction, Kr, solubility product of barium sulfate, Ksp, and the dissociation of BaII–aminocarboxylate complexes, Kd.
| |
On the other hand, the solubility product, Ksp, i.e. the reciprocal of Kp, of barium sulfate increased slightly with a temperature increase from 25 to 100
°C, and then decreased at temperatures above 100
°C (Fig. 2).31,32 In the case of the BaII–EDTA complex, the equilibrium constant, Kf, for the formation of BaSO4via dissociation of the BaII–aminocarboxylate complex [eqn. (5)] hardly changed with temperature (Fig. 2). When barium sulfate was synthesized in a BaII–EDTA solution, the particle size and degree of precipitated Ba2+ ion increased with temperature.33 It was concluded that the dissociation rate of the BaII–EDTA complex was related to the supply of barium ion to the solution.
Thermodynamic calculations suggested that the precipitated BaSO4 particles aggregated and assumed a spindle-shaped morphology using BaII–NTA, –HIDA and –HPDTA complexes because of their relatively large dissociation constants. When the dissociation constant was large, the complex was unstable and the Ba2+ ion was easily released into the solution. Therefore, the rate of barium sulfate precipitation was fast and many nuclei were produced. This inhibited the growth of primary particles, allowing the particles to aggregate.
Effect of factors on the morphology of BaSO4 particles
Chelating agents, such as EDTA, EGTA and NTA, which resulted in barium sulfate particles with rod-like, rhombohedral, and spindle-shaped morphology, respectively, were compared to examine the effects of reaction conditions, such as the molar ratio of [SO42−]/[acb], temperature and reaction time, on the morphology of barium sulfate. The cause of the morphological change was examined by surface analysis and crystallography.
Effect of reaction conditions.
EDTA33.
Under the experimental conditions, [EDTA]/[Ba2+] molar ratio from 1.5 to 2, [SO42−]/[EDTA] molar ratio from 2 to 5, 60 to 150
°C, and solution pH from 10 to 12, the morphology rarely changed and the BaSO4 was needle- or rod-shaped. The size of the particles increased with the [SO42−]/[EDTA] molar ratio. The length of the long axis of the particles varied from 1 to 5 µm.
EGTA.
Under the experimental conditions, [EGTA]/[Ba2+] molar ratio 1.5, [SO42−]/[EGTA] molar ratio from 2 to 5, 90 to 150
°C, and reaction time from 1 to 4 h, the BaSO4 was commonly rhombohedral and the morphology was independent of the [SO42−]/[EGTA] molar ratio and reaction time. The particle size increased with reaction time. The length of the long axis of the particles ranged from 2 to 7 µm. Under constant experimental conditions, [EGTA]/[Ba2+] molar ratio 1.5, [SO42−]/[EGTA] molar ratio 5, and reaction time 2 h, the barium sulfate synthesized at 90 to 150
°C (Fig. 3) was rhombohedral and the morphology was independent of temperature. The particle size rarely changed.
![SEM photographs of BaSO4 particles precipitated in BaII(EGTA)2−–Na2SO4 solutions after 2 h. [EGTA]/[Ba2+]⊕=⊕1.5, [SO42−]/[EGTA]⊕=⊕5, at (a) 90 °C, (b) 120 °C and (c) 150 °C.](/image/article/2001/CE/b009571k/b009571k-f3.gif) |
| Fig. 3
SEM photographs of BaSO4 particles precipitated in BaII(EGTA)2−–Na2SO4 solutions after 2 h. [EGTA]/[Ba2+]⊕=⊕1.5, [SO42−]/[EGTA]⊕=⊕5, at (a) 90 °C, (b) 120 °C and (c) 150 °C.
| |
NTA.
Fig. 4 shows SEM photographs of BaSO4 particles formed with a [NTA]/[Ba2+] molar ratio of 2, [SO42−]/[NTA] molar ratio of 0.5, reaction time 2 h, 25 to 150
°C. The particles formed spindles and the morphology was independent of the temperature. The length of the long axis of the particles varied from 0.6 to 1.2 µm. Primary particles aggregated and formed the spindle morphology.
![SEM photographs of BaSO4 particles precipitated in BaII(NTA)−–Na2SO4 solutions after 2 h. [NTA]/[Ba2+]⊕=⊕2, [SO42−]/[NTA]⊕=⊕0.5, at (a) 25 °C, (b) and (c) 90 °C and (d) 120 °C.](/image/article/2001/CE/b009571k/b009571k-f4.gif) |
| Fig. 4
SEM photographs of BaSO4 particles precipitated in BaII(NTA)−–Na2SO4 solutions after 2 h. [NTA]/[Ba2+]⊕=⊕2, [SO42−]/[NTA]⊕=⊕0.5, at (a) 25 °C, (b) and (c) 90 °C and (d) 120 °C.
| |
XPS analysis.
N 1s XPS was used to measure the barium sulfate synthesized using three kinds of aminocarboxylate, EDTA, EGTA and NTA. The N 1s XPS spectra for the surface of the barium sulfate particle and the spectra after argon ion sputtering for 15 s are shown in Fig. 5. The peak was detected near 400 eV for the particles of barium sulfate synthesized using EDTA or NTA as the chelating agent.34,35 This binding energy is in agreement with the values for the nitrogen atom contained in amines, imines and amides.34,35 This peak was not detected in the case of barium sulfate synthesized with EGTA. After argon ion sputtering, the area of the peak around 400 eV decreased for EDTA and NTA. When the barium sulfate was synthesized from EDTA or NTA, a very small amount of the aminocarboxylate was adsorbed on the particle surface. This should have influenced the particle morphology. However, since the precipitation rate of barium sulfate is high when using NTA, NTA was thought to be adsorbed on the particle surfaces irregularly, rather than on a specific surface. Consequently, the barium sulfate particles aggregated into spindles.
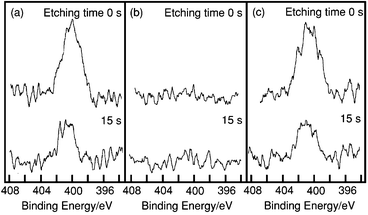 |
| Fig. 5
N 1s XPS spectra of the surface of BaSO4 precipitated in BaII–aminocarboxylate–Na2SO4 solution after 2 h at 120 °C after ion sputtering for 0 and 15 s. Aminocarboxylates: (a) EDTA, (b) EGTA, (c) NTA.
| |
Surface structure of the synthesized particles.
The peak intensities of the (101) and (021) faces on the X-ray diffraction patterns of barium sulfate synthesized from EDTA and EGTA are shown in Table 2. The peak intensity ratio of (101)/[(101)⊕+⊕(021)] using EGTA was smaller than that using EDTA. The surface analysis suggested that dissociated EDTA was adsorbed on the {101} surface. If EDTA was absorbed, the growth of crystal in the direction of [101] should be inhibited and there should be growth in the [010] direction. If such inhibition occurred, the morphology of the barium sulfate should change from rhombohedral, the stable crystal shape when conditions on the {021} and {001} surfaces are in equilibrium, to rod-like, produced by growth from the {101} surface (Fig. 6). EGTA was not thought to be absorbed on the surface of barium sulfate; therefore, the morphology of barium sulfate may become a stable rhombohedron using this chelating agent.
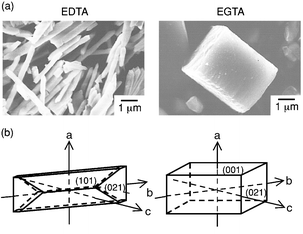 |
| Fig. 6
The morphology of BaSO4 precipitated in BaII–aminocarboxylate–Na2SO4 solutions: (a) SEM photographs, (b) schematic representation.
| |
Table 2
Effect of complexing agent and temperature on the intensity ratio of specific peaks in the X-ray diffraction patterns of synthesized BaSO4. [acb]/[Ba2+]⊕=⊕1.5, [SO42−]/[acb]⊕=⊕5, 2 h
Aminocarboxylate |
T/°C |
I(101)/[I(021)⊕+⊕I(101)]a |
I denotes the peak intensity of the XRD pattern.
|
EGTA |
150 |
0.13 |
|
90 |
0.24 |
EDTA |
150 |
0.39 |
|
90 |
0.32 |
The Ba2+ and SO42− ions appeared alternately on the {101} surface and one S–O bond of the sulfate ion was directed out of the surface (Fig. 7). This should cause polarization of the surface charge of the barium sulfate crystal.6,8,36 The aminocarboxylate should be absorbed on this surface. On the other hand, since the oxygen atom of the sulfate ion was mainly arranged on the {021} surface (Fig. 7), few carboxylates should adsorb on the {021} surface.
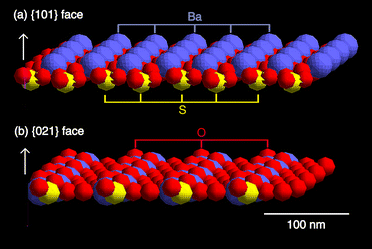 |
| Fig. 7
Plain view of the surface structure of (a) {101} face and (b) {021} face of BaSO4.
| |
Synthesis of BaSO4 using mixed aminocarboxylates
Mixed prexursors with EDTA and EGTA.
Fig. 8 shows SEM photographs of barium sulfate particles synthesized with an ([EDTA]⊕+⊕[EGTA])/[Ba2+] molar ratio of 2 and a [SO42−]/([EDTA]⊕+⊕[EGTA]) molar ratio of 5, at 150
°C for 2 h, when the [EGTA]/[EDTA] molar ratio was 1, 3 or 15. When the [EGTA]/[EDTA] molar ratio was 1 or 3, the barium sulfate was rod-like. The morphology became rhombohedral when the molar ratio was increased to 15. The amount of precipitated barium ion under the conditions described above is shown in Fig. 9. The [EGTA]/[EDTA] molar ratio hardly affected the amount of precipitated barium ion. Therefore, the dissociation constants of the BaII–EDTA and BaII–EGTA complexes were roughly equal at 150
°C.
![SEM photographs of BaSO4 particles precipitated in BaII(EDTA)2−–BaII(EGTA)2−–Na2SO4 solutions after 2 h at 150 °C. ([EDTA]+[EGTA])
/[Ba2+]⊕=⊕2, [SO42−]/([EDTA]⊕+⊕[EGTA])⊕=⊕5, [EGTA]/[EDTA]: (a) EDTA only, (b) 1, (c) 3, (d) 15 and (e) EGTA only.](/image/article/2001/CE/b009571k/b009571k-f8.gif) |
| Fig. 8
SEM photographs of BaSO4 particles precipitated in BaII(EDTA)2−–BaII(EGTA)2−–Na2SO4 solutions after 2 h at 150 °C. ([EDTA]+[EGTA])
/[Ba2+]⊕=⊕2, [SO42−]/([EDTA]⊕+⊕[EGTA])⊕=⊕5, [EGTA]/[EDTA]: (a) EDTA only, (b) 1, (c) 3, (d) 15 and (e) EGTA only.
| |
![Effect of the [EGTA]/[EDTA] molar ratio on the amount of precipitated Ba2+ ion in BaII(EDTA)2−–BaII(EGTA)2−–Na2SO4 solutions at 150 °C as a function of time. ([EDTA]⊕+⊕[EGTA])/[Ba2+]⊕=⊕2, [SO42−]/([EDTA]⊕+⊕[EGTA])⊕=⊕5, [EGTA]/[EDTA]: ●⊕=⊕1, ✦⊕=⊕3 and ▲⊕=⊕15.](/image/article/2001/CE/b009571k/b009571k-f9.gif) |
| Fig. 9
Effect of the [EGTA]/[EDTA] molar ratio on the amount of precipitated Ba2+ ion in BaII(EDTA)2−–BaII(EGTA)2−–Na2SO4 solutions at 150 °C as a function of time. ([EDTA]⊕+⊕[EGTA])/[Ba2+]⊕=⊕2, [SO42−]/([EDTA]⊕+⊕[EGTA])⊕=⊕5, [EGTA]/[EDTA]: ●⊕=⊕1, ✦⊕=⊕3 and ▲⊕=⊕15.
| |
Mixed precursors with EDTA and NTA.
Fig. 10 shows SEM photographs of barium sulfate particles synthesized under the conditions ([EDTA]⊕+⊕[NTA])/[Ba2+] molar ratio 2, [SO42−]/([EDTA]⊕+⊕[NTA]) molar ratio 2, 150
°C, 2 h, when the [NTA]/[EDTA] molar ratio was 1, 3 or 7. With an increase in the [NTA]/[EDTA] molar ratio, the morphology of the barium sulfate particles changed from rod-like to spindle-shaped via a spherical shape. The particle size increased with the [NTA]/[EDTA] molar ratio. The degree of precipitated barium sulfate ion under the same conditions is shown in Fig. 11. With an increase in the [NTA]/[EDTA] molar ratio, the morphology was affected greatly by NTA and the amount of precipitated barium ion increased. Therefore, the difference between the dissociation constants of the BaII–NTA and BaII–EDTA complexes was large at 150
°C.
![SEM photographs of BaSO4 particles precipitated in BaII(EDTA)2−–BaII(NTA)−–Na2SO4 solutions after 2 h at 150 °C. ([EDTA]⊕+⊕[NTA])
/[Ba2+]⊕=⊕2, [SO42−]/([EDTA]⊕+⊕[NTA])⊕=⊕2, [NTA]/[EDTA]: (a) EDTA only, (b) 1, (c) 3, (d) 7 and (e) NTA only.](/image/article/2001/CE/b009571k/b009571k-f10.gif) |
| Fig. 10
SEM photographs of BaSO4 particles precipitated in BaII(EDTA)2−–BaII(NTA)−–Na2SO4 solutions after 2 h at 150 °C. ([EDTA]⊕+⊕[NTA])
/[Ba2+]⊕=⊕2, [SO42−]/([EDTA]⊕+⊕[NTA])⊕=⊕2, [NTA]/[EDTA]: (a) EDTA only, (b) 1, (c) 3, (d) 7 and (e) NTA only.
| |
![Effect of the [NTA]/[EDTA] molar ratio on the amount of precipitated Ba2+ ion in BaII(EDTA)2−–BaII(NTA)−–Na2SO4 solutions at 150 °C as a function of time. ([EDTA]⊕+⊕[NTA])
/[Ba2+]⊕=⊕2, [SO42−]/([EDTA]⊕+⊕[NTA])⊕=⊕2, [EGTA]/[EDTA]: ●⊕=⊕1, ✦⊕=⊕3, ▲⊕=⊕7 and ■⊕=⊕NTA only.](/image/article/2001/CE/b009571k/b009571k-f11.gif) |
| Fig. 11
Effect of the [NTA]/[EDTA] molar ratio on the amount of precipitated Ba2+ ion in BaII(EDTA)2−–BaII(NTA)−–Na2SO4 solutions at 150 °C as a function of time. ([EDTA]⊕+⊕[NTA])
/[Ba2+]⊕=⊕2, [SO42−]/([EDTA]⊕+⊕[NTA])⊕=⊕2, [EGTA]/[EDTA]: ●⊕=⊕1, ✦⊕=⊕3, ▲⊕=⊕7 and ■⊕=⊕NTA only.
| |
Summary
The morphology of barium sulfate was studied by hydrothermal synthesis using BaII–aminocarboxylate chelating precursors. When the aminocarboxylate was changed, the precipitated barium sulfate did not change its crystal structure, but changed its morphology dramatically. Using BaII–aminocarboxylate complexes with small dissociation constants, such as EDTA and EGTA, the barium sulfate was rod-like or rhombohedral. Using BaII–aminocarboxylate complexes with large dissociation constants, such as NTA, the barium sulfate was spindle-shaped. When using the same aminocarboxylate, reaction conditions, such as the molar ratio of [SO42−]/[EDTA] and temperature, hardly affected the morphology of the barium sulfate.
Barium sulfate synthesized with the BaII–EDTA complex had a larger peak intensity ratio of XRD patterns for (101)/(021) than that synthesized using BaII–EGTA. This indicated preferential growth on the {101} face when using EDTA, as compared to using EGTA. The chelating agent was not detected on the surface of barium sulfate synthesized from the BaII–EGTA complex, and the barium sulfate formed a stable rhombohedral crystal under equilibrium conditions. On the other hand, the chelating agent was observed on the surface of barium sulfate synthesized with the BaII–EDTA complex, and the morphology was rod-like. This likely occurred because the EDTA was adsorbed on a specific surface of the precipitated barium sulfate once it dissociated from the barium ion.
Acknowledgements
The computational simulation and modeling was performed by Computer-Assisted Molecule Design System with Cerius2 (Molecular Simulations Inc.) in ICRS, Tohoku University. We thank Mr Kei Takahashi and Dr Satoshi Uchida for their dedicated system support.
References
- M. C. van deer Leaden and G. M. van Rosmalen, J. Colloid Interface Sci., 1995, 171, 142 CrossRef.
- S. Kipp, R. Lacmann, M. Reichelt and W. Schröer, Chem. Eng. Technol., 1996, 19, 543 CrossRef CAS.
- R. J. Davey, S. N. Black, L. A. Bromley, D. Cottier, B. Dobbs and J. E. Rout, Nature, 1991, 353, 549 CrossRef CAS.
- S. N. Black, L. A. Bromley, D. Cottier, R. J. Davey, B. Dobbis and J. E. Rout, J. Chem. Soc., Faraday Trans., 1991, 87, 3409 RSC.
- L. A. Bromley, D. Cottier, R. J. Davey, B. Dobbis, S. Smith and B. R. Heywood, Langmuir, 1993, 9, 3594 CrossRef CAS.
- A. L. Rohl, D. H. Gay, R. J. Davey and C. R. A. Catlow, J. Am. Chem. Soc., 1996, 118, 642 CrossRef CAS.
- W. J. Benton, I. R. Collins, I. M. Grimsey, G. M. Parkinson and S. A. Rodger, Faraday Discuss., 1993, 95, 281 RSC.
- S. E. Redfern and S. C. Parker, J. Chem. Soc., Faraday Trans., 1998, 94, 1947 RSC.
- P. Hartman and C. S. Strom, J. Cryst. Growth, 1989, 97, 502 CrossRef CAS.
- D. Bosbach, C. Hall and A. Putnis, Chem. Geol., 1998, 151, 143 CrossRef CAS.
- K.-S. Wang, R. Resch, K. Dunn, P. Shuler, Y. Tang, B. E. Koel and T. F. Yen, Colloids Surf., A, 1999, 160, 217 CrossRef CAS.
- K. Dunn and T. F. Yen, Environ. Sci. Technol., 1999, 33, 2821 CrossRef CAS.
- K. Takiyama, Bull. Chem. Soc. Jpn., 1958, 31, 950 CrossRef CAS.
- S. Tohno, H. Takano and K. Takahashi, J. Aerosol Res., 1993, 8, 160 CAS.
- H. Nishizawa, T. Tani and K. Matsuoka, J. Am. Ceram. Soc., 1984, 67, C-98 CrossRef CAS.
- Y. Fujishiro, H. Yabuki, K. Kawamura, T. Sato and A. Okuwaki, J. Chem. Tech. Biotechnol., 1993, 57, 349 CrossRef CAS PubMed.
- H. Ito, Y. Fujishiro, T. Sato and A. Okuwaki, Br. Ceram. Trans., 1995, 94, 146 CAS.
- Y. Fujishiro, H. Ito, T. Sato and A. Okuwaki, J. Alloys Compd., 1997, 252, 103 CrossRef CAS.
- Joint Commiittee on Powder Diffraction Standards, Diffraction Data File No. 24–1035. JCPDS International Centre for Diffraction Data, Swarthmore, PA, 1974. Search PubMed.
-
A. E. Martell and R. M. Smith, Critical Stability Constants, Plenum, New York, 1974, vol. 1. Search PubMed.
- L. C. Thompson and S. K. Kundra, J. Inorg. Nucl. Chem., 1966, 28, 2945 CrossRef CAS.
- S. A. Wood, D. J. Wesolowski and D. A. Palmer, Chem. Geol., 2000, 167, 231 CrossRef CAS.
- P. Bénézeth and D. A. Palmer, Chem. Geol., 2000, 167, 11 CrossRef.
- M. K. Ridley, D. A. Palmer, D. J. Wesolowski and R. M. Kettler, Geochim. Cosmochim. Acta, 1998, 62, 2279 CrossRef CAS.
- M. K. Ridley, D. A. Palmer, D. J. Wesolowski and R. M. Kettler, J. Solution Chem., 1998, 27, 195 CrossRef CAS.
- D. A. Palmer and K. E. Hyde, Geochim. Cosmochim. Acta, 1993, 27, 1393 CrossRef.
- D. A. Palmer and S. E. Drummond, J. Phys. Chem., 1988, 92, 6795 CrossRef CAS.
- P. Prapaipong, E. L. Shock and C. M. Koretsky, Geochim. Cosmochim. Acta, 1999, 63, 2547 CrossRef CAS.
- J. K. Hovey, L. G. Hepler and P. R. Tremaine, J. Solution Chem., 1986, 15, 977 CrossRef CAS.
- J. K. Hovey, L. G. Hepler and P. R. Tremaine, Can. J. Chem., 1988, 66, 881 CrossRef CAS.
- K. Raju and G. Atkinson, J. Chem. Eng. Data, 1988, 33, 490 CrossRef CAS.
- C. Monnin, Chem. Geol., 1999, 153, 187 CrossRef CAS.
- M. Uchida, A. Sue, T. Yoshioka and A. Okuwaki, J. Mater. Sci. Lett., 2000, 19, 1373 CrossRef CAS.
- L. J. Gerenser, J. M. Grace, G. Apai and P. M. Thompson, Surf. Interface Anal., 2000, 29, 12 CrossRef CAS.
- S. Gélinas, J. A. Finch and A. J. Vreugdenhil, Int. J. Min. Proc., 2000, 59, 1 CrossRef.
- N. L. Allan, A. L. Rohl, D. H. Gay, C. R. A. Catlow, R. J. Davey and W. C. Mackrodt, Faraday Discuss., 1993, 98, 273 RSC.
|
This journal is © The Royal Society of Chemistry 2001 |