DOI:
10.1039/D1TB02408F
(Review Article)
J. Mater. Chem. B, 2022,
10, 1520-1552
Nano dimensions/adjuvants in COVID-19 vaccines
Received
3rd November 2021
, Accepted 27th January 2022
First published on 15th February 2022
Abstract
A favorable outcome of the COVID-19 crisis might be achieved with massive vaccination. The proposed vaccines contain several different vaccine active principles (VAP), such as inactivated virus, antigen, mRNA, and DNA, which are associated with either standard adjuvants or nanomaterials (NM) such as liposomes in Moderna's and BioNTech/Pfizer's vaccines. COVID-19 vaccine adjuvants may be chosen among liposomes or other types of NM composed for example of graphene oxide, carbon nanotubes, micelles, exosomes, membrane vesicles, polymers, or metallic NM, taking inspiration from cancer nano-vaccines, whose adjuvants may share some of their properties with those of viral vaccines. The mechanisms of action of nano-adjuvants are based on the facilitation by NM of targeting certain regions of immune interest such as the mucus, lymph nodes, and zones of infection or blood irrigation, the possible modulation of the type of attachment of the VAP to NM, in particular VAP positioning on the NM external surface to favor VAP presentation to antigen presenting cells (APC) or VAP encapsulation within NM to prevent VAP degradation, and the possibility to adjust the nature of the immune response by tuning the physico-chemical properties of NM such as their size, surface charge, or composition. The use of NM as adjuvants or the presence of nano-dimensions in COVID-19 vaccines does not only have the potential to improve the vaccine benefit/risk ratio, but also to reduce the dose of vaccine necessary to reach full efficacy. It could therefore ease the overall spread of COVID-19 vaccines within a sufficiently large portion of the world population to exit the current crisis.
Introduction
To overcome the crisis generated by the COVID-19 pandemic, vaccination of the population seems to be one of the best options. While some vaccines are or were marketed and distributed by Moderna, Johnson and Johnson, Astra Zeneca, and Janssen, others are under development.1 An intensification of vaccine production seems necessary to be able to vaccinate a sufficiently large portion of the world population to achieve collective immunity while limiting the number of deaths caused by the pandemic. The offered vaccines can be categorized in two different types. The first one consists of vaccines whose content should directly mimic COVID-19, comprising in this case replicating adenoviruses (AZD1222 of astrazeneca, Ad5-nCOVB from CanSino biologics, Ad26COV2.S from Janssen), inactivated COV (CoronaVac of Sinovac or BBIBP-CorV of Sinopharm), or various COV proteins (NVX-COV2373 from Novavax, COVX-19 from PTY vaccine). The second one is composed of elements containing the genetic material able to drive the cellular production of proteins or elements mimicking COVID 19 behavior, i.e. essentially mRNA, which is encapsulated in or associated with liposomes or lipid nanoparticles to ensure mRNA protection against degradation (mRNA-1273 of Moderna and Comirnaty of Pfizer-BioNTech), or DNA (AG0302 of AnGes).
Considerable efforts, unparalleled in the history of medicine, have been deployed to bring to market efficient vaccines against COVID-19. These efforts have been largely successful, leading to the emergence of several efficient vaccines in a record time of just a few months. However, the COVID 19 crisis has given rise to a new problem, which was largely unanticipated, i.e. a worldwide infection requiring massive vaccination. The vaccine does not only need to reach a high efficacy, but also to be available in large quantities within the shortest possible time period.
To improve vaccine availability, existing vaccines could be injected in smaller doses while remaining effective. Alternatively, new vaccines, which are easier to mass-produce than existing ones, could be developed. Nanomaterials (NM) can help reaching either one of these two objectives thanks to a number of their properties such as the protection of the vaccine active principle (VAP) against degradation, the improvement of VAP solubility, the successful delivery of VAP to a site of interest, e.g. mucus where COVID 19 replication is favored due to the presence of ACE2 receptors in large quantity, the combination within the same unit of several different VAP that can act synergically against COVID 19.2,3 In fact, NM are already being introduced into Moderna's and BioNTech/Pfizer's vaccines to achieve some of these effects.4 Other vaccines would certainly also benefit from the presence of certain safe NM in their composition.
This review complements previous reports describing the use of NM in COVID-19 vaccines,2–7 in several respects, i.e. first by presenting NM under the angle of vaccine adjuvants, i.e. a different component from VAP essentially consisting of antigens in various forms, mRNA, or DNA, second by describing the presence of various nano-dimensions in COVID-19 vaccines, third by introducing NM as vaccine components that were originally tested against cancer before being used against COVID-19, i.e. that NM can in some cases undergo a shift of medical fields from being tested in cancerology to being used in virology, fourth by describing in detail the different types of NM that could be introduced in COVID-19 vaccines, fifth by pointing out NM advantages compared with standard vaccine adjuvants, and sixth by describing NM mechanisms of action, in particular immune ones.
I. Should nano components of vaccines be considered as adjuvants or active principles?
An adjuvant can be considered as a product added to a vaccine to strengthen the antigen-triggered immune response, e.g. by improving antigen tissue deposition. The most well-known adjuvants are Freund's adjuvant, a water-in-oil emulsion containing inactivated Mycobacterium tuberculosis bacteria,8 and alum, an alumina hydroxide/phosphate with which the antigen is precipitated.8
While at first glance this definition may seem clear, it can in fact be prone to different interpretations, especially when it comes to applying it to nanomaterials. The role of NM in vaccines is therefore examined to determine whether these materials should be considered as adjuvants or as active principles. First, NM can have a protective role towards antigens or mRNA/DNA and avoid their degradation, e.g. by preventing RNA degradation by RNase.9 Second, NM can serve as vehicle to carry VAP, either by facilitating VAP administration in a specific region of the organism, e.g. the mucus, or by enabling the targeting of certain cells of immune interests such as APC.10 Concerning these first two properties, they may be associated with adjuvant functions since the dominant activity remains due to the VAP, the nano-adjuvant enhancing vaccine efficacy. Third, NM-based vaccines often contain in addition to NM other substances, which display specific immune activities, e.g. CPG, a TLR-9 agonist, MPL-A, a TLR-4 agonist, or anti-PD1/anti-PDL-1 (Table 1). While in some cases, only these additional substances are considered as adjuvant,11 it seems that both such substances and NM can be classified as such.12 Fourth, instead of being only perceived as a compound, adjuvant can in some cases be associated with one or several property(ies) resulting from a specific assembly of different VAP. In this case, an adjuvant property can be due to the presence of a complex of nanometric size in a vaccine, originating for example from the concentration/aggregation of VAP, as it is the case for ssRNA concentrates,13 and self-aggregating peptides.14 Such adjuvant property can ease NM and associated VAP transportation through certain physiological barriers, in particular the mucous membrane,7 and the holes crossing angiogenic blood vessels,15 and NM efficient targeting of specific cells/pathogens.5 Taken as a whole, these properties can contribute to strengthen the immune response against pathogens, hence falling within the scope of the adjuvant definition.
Table 1 For different types of nano-formulated vaccines, information concerning their size, the category of vaccine to which they belong, the nano-adjuvants and active principle they contain, their mode of action, the immune response they generate with the efficacy induced against the pathogen when it is described, the treated disease, the implemented targeting when it is present, and the recommended mode of vaccine administration
Nano-formulation vaccine |
Size |
Type of vaccine |
Nano-adjuvant |
Active principle |
Mode of action |
Treated disease |
Immune response |
Targeting |
Admin |
Ref. |
ZnO NP + Ova |
12 nm (2.5 nm pore) |
Antigenic |
mZnO |
Ova |
Antigen-specific T cell response |
Multiple |
↑ CD4+ and CD8+ T-cells; ↑ IgG2a, IgG2b, IFN-γ; →Th1 immune response |
None |
Sub-cutaneous |
117
|
Calcium phosphate NP + DNA |
10–150 nm |
DNA |
CaP NP |
DNA encoding GRA14 |
Cellular and humoral immune responses against Toxoplasma infection |
Toxoplasma infection |
↑ IgG1 and IgG2a antibodies; ↑ lymphocyte proliferation; ↑ IFN-γ and IL-4; mice infected by parasite live 5 days longer when vaccinated |
None |
im |
178
|
HMS + VLP |
1 μm (silica) 20 nm (VLP) |
VLP |
Hollow mesoporous silica (HMS) |
VLP |
Cellular and humoral response against FMD |
Foot and mouth disease (FMD) |
↑ Antibody during >3 months; ↑ T-lymphocyte proliferation; ↑ IFN-γ |
None |
im |
181
|
PLGA-chitosan + pDNA in poloxamer gel |
350–450 nm |
DNA |
PLGA-chitosan |
pDNA |
Th1/Th2 immune response |
Rabies |
↑ GnRH antibodies induced during >9 weeks; ↑ IgG2a + IgG1 and cytokines IFN-γ and IL-4 |
Laser microporation (delivery through skin) |
im |
182
|
PE + PLGA NP |
160 nm |
NA |
PLGA NP |
Roasted peanut extract (PE) |
NP help active principle cross mucus barrier |
PE allergy |
↓ Anaphylaxis symptoms ↑ Higher survival for vaccinated mice |
None |
Oral |
183
|
NS + ASTING |
100 nm |
Cancer |
Hollow polymeric nanoshell (NS) |
Agonist of stimulator of intereferon gene |
Adaptive immune response against tumor |
Colon cancer |
↑ Antigen presentation and anticancer adaptive response; ↓ tumor progression in cancer bearing mice |
None |
it |
184
|
ICG + imiquimod encapsulated in PLGA + checkpoint-blockade (CTLA4) |
100 nm |
Cancer |
PLGA |
ICG (PT agent) + imiquimod (TLR-7 agonist) + checkpoint-blockade (CTLA4) |
PTT combined with checkpoint-blockade immunotherapy |
Breast + colorectal cancer |
↑ T cells; ↓ Treg cells; ↑ INF-γ et TNF-α; ↑ Effector memory T cells (TEM) PLGA-ICG-R837 + laser + anti-CTLA4: tumor growth retardation in mice |
None |
iv |
185
|
Clay (heterocyte) NP + IB |
75 nm |
E. coli
|
Clay |
E. coli antigen: intimin β (IB). |
Humoral and cellular immune responses against EHEC antigen commercially used adjuvants – QuilA and Alum |
E. coli infection |
• Clay NP: maturation of RAW 264.7 macrophages + ↑ Cytokines; mice immunized with IB + clay NP: ↑ IgA, protects against E. coli infection |
None |
Sub-cutaneous |
186
|
Dendritic large pore silica NP + CuS NP inside pores + resiquimod + cancer cell membrane + anti-PD1 peptide (AUNP-12) |
CuS (5–10 nm) complex (150 nm) |
Cancer |
CuS NP in pores of silica |
Resiquimod + cancer cell membrane + anti-PD1 peptide (AUNP-12) |
Thermal ablation of primary tumor + vaccination effect to prevent tumor recurrence |
Triple negative BC |
↑ T cells to prevent TNBC recurrence and metastasis |
high TNBC-targeting |
iv |
187
|
First or second LP + RA |
200–7000 nm |
Bacteria |
First LP: 1,2-distearoyl-sn-glycero-3-phosphocholine stabilized with PEG or Second LP cationic liposomal adjuvant CAF01 |
Retinoic acid (RA) |
Vaccine formulation that upon parenteral administration induced intestinal IgA |
Enteric infection |
RA + vaccine antigens sent to local lymph nodes → mucosal immune response (antigen migration through antigen presenting cells); CAF23 → antigen specific intestinal IgA response |
NA |
SC |
73
|
Polysaccharide NP + PCS5 + poly (I:C) |
110–220 nm |
Peptide |
Poly-saccharide NP composed of chitosan or hyaluronic acid |
PCS5 (protease cleavage sites peptide) |
Generation of humoral and cellular responses |
HIV |
High anti-PCS5 antibodies; activation of antigen-presenting cells; T-cell activation |
None |
Intra-muscular |
188
|
Nano-11 + KAg |
30 nm (Nano-11) 500 nm (Nano-11 + Kag) |
Antigenic |
Positively charged corned-derived NP |
Kag inactivated/killed swine influenza virus antigen |
Immune reaction against virus |
Influenza |
↑ T-helper 1 and T-helper 2 transcription factors; ↑ T cells ↑ IgA in the nasal cavity; ↑ IFN-γ; ↑ observation in pigs |
None |
Intra-muscular/intra-nasal |
189
|
Lipid–hyaluronic acid multi-cross-linked hybrid NP + with protein antigen |
130–350 nm |
Antigenic |
lipids |
Protein antigen |
Cellular and immural response |
Ebola virus (EBOV) |
MVP accumulate in DC + ↑ Antigen processing; Mice immunized with MVP → CD8+ and CD4+; MVP delivering EBOV glycoprotein → 80% protection against EBOV |
None |
Sub-cutansous |
190
|
Psi@AcDex + CCM ± check point inhibitor |
NA |
Anti-cancer |
Porous silicon (Psi) encapsulated within a layer of acetylated dextran (AcDEX) |
Cancer cell membrane (CCM) surrounding Psi@AcDex |
Check point inhibitor |
Melanoma |
Mice bearing melanoma tumor treated twice with nanovaccine formulation → control on tumor progression (administration of nanovaccine + check point inhibitor more efficient than administration of checkpoint inhibitor alone) |
None |
Sub-cutaneous |
191
|
YC-NPs coated with Acr, Ag85B, and HBHA (Mtb antigens) |
320–350 nm |
Anti-bacteria |
Yellow carnauba wax NPs (YC-NPs) |
3 fusion proteins |
Cellular + humoral |
TB |
↑ Activation of CD4+/CD8+ T cell compared with BCG; generation of Ag85B-specific serum IgG and respiratory IgA; |
None |
Intra-nasal or intra-tracheal |
192
|
APNP + ovalbumin (OVA) and CpG |
85 nm |
Anti-cancer |
Nano-sized vaccine carriers AlO(OH) polymer nanoparticles (APNP) |
(OVA) and CpG |
Cellular + humoral |
Cancer |
Activation CD8+ T cell; aluminium in NP more efficient than aluminium in gel (old adjuvant); Vaccine ↑ survival of mice with melanoma |
None |
NA |
193
|
Nano silicon particle + TGEV |
70 nm |
Virus |
Nano silicon |
Inactivated transmissible gastroenteritis virus (TGEV) |
activate immunity through Toll-like receptor activation |
Veterinary vaccine |
Nano vaccine ↑ antibody titers, ↑ IL-6, ↑ TNF-α ↑ IFN-γ, ↑ CD3+ T cells, ↑ CD4+/CD8+ T lymphocyte |
None |
Sub-cutaneous |
194
|
Phospholipid bilayer and a phosphate calcium core + cancer membrane proteins (CMP) + α-helix HSP70 functional peptide (αHSP70p) + CPG |
20–30 nm |
Anti-cancer |
Phospholipid bilayer and a phosphate calcium core |
Cancer membrane proteins (CMP) + α-helix HSP70 functional peptide (αHSP70p) + CPG |
Cellular + humoral |
Cancer |
Vaccination → lymph node trafficking and multi-epitope-T cells response; Vaccine + anti-PD-1 antibody → tumor regression in mice with melanoma. |
None |
Sub-cutaneous |
195
|
RBD-Ferritin; RBD-mi3; RBD-I53-50 |
30–50 nm |
Virus |
Ferritin NP; Mi3 NP; I53-50 NP |
Spike protein receptor binding domain (RBD) |
Blockage of RBD binding to ACE2 |
COVID 19 |
RBD-NP + AddaVax or Sigma adjuvant injected to mice: neutralize virus better than RBD |
None |
Sub-cutaneous |
196
|
NE loaded with TLR7/8 agonists |
150 nm |
Anti-cancer |
Nano-emulsion |
TLR7/8 agonists |
Suppress immuno-suppressive TME |
Cancer |
Lymphocytes and innate immune cells recruited against tumor; polarization of M2 macrophages: → Tumor growth inhibition in mice |
None |
Sub-cutaneous |
197
|
pH-Responsive polymeric NP + protein antigen + nucleic acid adjuvant |
20–80 nm |
Virus |
polymeric NP |
Protein antigen |
Involvement of tissue-resident memory T cells (TRM) |
vaccinia + influenza |
CD8+ TRM cells in lungs; ↑ APC activation; ↑ cytokine production by TRM |
None |
Intra-nasal |
198
|
IQ/PN NP + anti-PLD1 |
50–100 nm |
Cancer |
Photoresponsive polydopamine NP (PN) |
Imiquimod + anti-PLD1 |
Targets cancer cells overexpressing PDL1; Immune check point inhibitor; |
Cancer |
Mice bearing CT26 injected with IQ/PN NP + anti-PLD1: tumor accumulation + tumor ablation following NIR irradiation + tumor growth prevention of secondary tumor → 100% mouse survival; ↑ T cells in tumor tissues |
PDL1 |
Intra-venous |
199
|
PapMV |
80 nm |
Cancer |
Papaya mosaic virus nanoparticle (PapMV) |
PapMV |
↑ Tumor-specific (CD8+ T) ↓ myeloid-derived suppressor cells (MDSC) |
Cancer |
Mice with melanoma injected it/iv with PapMV → tumor growth delay + increased survival + less lung metastases |
None |
Intra-tumor + intra-venous |
200
|
OMV with low endotoxins |
50–150 nm |
Virus |
Outer membrane vesicles (OMV) |
LPS |
Immune stimulation by attenuated LPS |
Influenza |
↑ antibody + T cell, IgA, lung-resident influenza-specific T cells |
None |
Intra-nasal |
90
|
MPLA + OVA encapsulated in Psomes |
100 nm |
Cancer |
Polymersomes (Psomes) |
MPLA + OVA |
Polymersomes promote the cellular uptake of OVA antigens by APCs |
Cancer |
OVA to APC; Psomes/OVA/MPLA injected to mice→ production of IL-6 + TNF-α cytokines + OVA-specific antibody |
None |
Intra-musculary |
201
|
Nano-11 + antigen |
70–80 nm |
NA |
Nano-11: cationic dendritic-like α-D-glucan NP |
Antigen (OVA) |
Targeting of APC (nano-11 enable progressive release of antigen at injection site for 3 weeks) |
NA |
↑ Monocytes; macrophages; ↑ macrophage Mc2+ phagocyte Nano-11; Nano-11 enable delivery of more antigens to APC than aluminium hydroxide adjuvant |
Dendritic cells |
Intra-musculary |
202
|
HAuNS + APP encapsulated in PLGA NP |
400–600 nm |
Cancer |
Gold nanoshells (HAuNS, a photothermal agent) |
An anti PD-1 peptide, APP |
Heat + PD1 blockade |
Cancer |
Sustained release of AAP (replace frequent administration of APP); PD1 blockade immunotherapy; PTA + NP eradicate primary tumors and inhibit metastatic growth in mice |
None |
Intra-tumoral |
203
|
GO-Car + OVA |
20–50 nm |
NA |
Go-Car: graphene oxide + carnosine |
OVA (antigen) |
Immune reaction |
NA |
GO-Car + OVA injected to mice: ↑ Antibody; C4+, CD8+ T cells |
None |
Intra-muscular |
204
|
ROP18 protein encapsulated in PLGA NP |
200 nm |
Pathogen |
PLGA NP |
ROP18 proteins |
Humoral |
Toxo-plasmose |
↑ IgA, IgG2a |
None |
Intra-nasal |
205
|
TMC + α-PGA + specific antigen |
200–1000 nm |
Bacteria |
PGA |
Peptide antigen |
NP improves antigen delivery |
Bacteria (strepto-coccus) |
↑ Systemic and mucosal antibody due to NP |
None |
Intra-nasal |
206
|
QS-21 + subunit protein + nano-patch |
Nano-patch (20 000 injection tips per cm2) |
Virus |
Nano-patch (admin) Saponin adjuvant (QS-21) |
Subunit protein antigen |
Humoral response |
Influenza |
With nano-patch: • use of 1/100th of the IM antigen dose necessary with syringe; • ↑ Immune response; • less adjuvant QS-21 necessary for immune stimulation compared with IM injection |
None |
Nano-patch |
207 and 208
|
Lipid NP encapsulating mRNA + LPS |
50–150 nm |
Cancer |
Lipid NP + LPS |
mRNA |
mRNA in cytosol of APC: coding for tumor-associated antigen gp100 and TRP2 |
Cancer |
CD8 T cell |
APC (intra-cellular) |
Intra-venous |
209
|
DNA + nano-aluminium |
65 nm (nano-aluminium) |
Toxoplasma gondii |
Nano-alumium |
DNA |
DNA encoding ROP13 and GRA14 |
Toxo-plasmose |
In vaccinated mice: • ↑ production of IgG2a, IFN-γ, IgG antibodies/cytokines against toxoplasmosis. • ↑ survival in presence of parasite |
None |
Intra-muscularly |
210
|
ssRNA |
|
NA |
ssRNA (single stranded RNA) |
None |
Stimulation of Th1 and Th2 responses |
NA |
Following mouse injection: • no tissue damage; • No increase in IgE and anti-nuclear antibodies; → No sign of autoimmune response; → ssRNA safe adjuvant |
None |
Intra-muscularly |
13
|
PDDA/OVA NP |
170 nm |
NA |
PDDA (poly(diallyldimethylammonium chloride)) |
Ovalbumin (OVA) |
↑ OVA-specific antibodies compared with Al(OH)3/OVA. |
|
Mice receiving vaccine: • ↑ OVA-specific IgG1; • ↓ OVA-specific IgG2a production; • → Th-2 response; Nano-metric size favors antigen presentation by APC at the lymph nodes; PDDA advantages: simple, low concentration needed, low cytotoxicity, yields high stability |
None |
Intra-nasal |
211
|
VP1 protein antigen + TNF-α or CPG encapsulated in PE |
90–130 nm |
Virus |
Poly-electrolyte (PE) (chitosan/heparin) |
VP1 protein antigen of enterovirus 71 |
Prolonged retention in lymph nodes; |
Hand–foot–mouth disease (HFMD) |
↑ Th1, Th2 immune response; ↑ IgA titers → mucosal protection |
Lymph node |
Sub-cutansous |
11
|
CPG (DNA) microcapsules (>4 million CPG copies) |
2.5 μm (capsule diameter) |
NA |
CaCO3 microcapsule |
CPG |
Microcapsules increases CPG stability |
NA |
In vitro study: • Cell internalization + endosome accumulation; • TLR-9 activated by CpG; • Microcapsule ↑ TNF-α + IL-6 by macrophages |
NA |
NA |
212
|
ROM4 + GRA14 + CaP NP |
90–120 nm |
Parasite |
CaP NP |
Cocktail DNA vaccines (ROM4 + GRA14) of Toxoplasma gondii |
Better immune reaction with CaP NP |
Toxo-plasmose |
Mice immunized with cocktail DNA + CaPN NP protected against toxoplasmose |
None |
Intra-muscular |
213
|
Antigen + poly(I:C) adsorbed on Nano-11 |
214 nm |
Virus |
cationic alpha-D-glucan NP (Nano-11) |
TLR3 agonist (poly(I:C)) |
Better immune response compared with commercial vaccine |
Influenza |
Pigs immunized with vaccine: ↑ of virus-specific SIgA antibodies in lungs compared with commercial influenza vaccine; ↑ cytokine mRNA expressions in lymph nodes compared to the commercial influenza vaccine; ↓ Lung lesions/virus load compared with commercial influenza vaccine |
None |
Intra-nasal |
177
|
PLGA + H56 TB vaccine |
150–500 nm |
Bacteria |
PLGA |
H56 TB vaccine |
No better immune response due to PLGA |
Tuberculosis |
Immunogenicity of antigen encapsulated in PLGA |
None |
Intra-nasal + sub-cutaneous |
214
|
Hexosome containing lipid phytantriol (Phy) + immunopotentiator monomycoloyl glycerol-1 (MMG-1) encapsulating MOMP |
100–150 nm |
NA |
Hexosomes |
Antigen: Chlamydia trachomatis major outer membrane protein (MOMP) |
Humoral + T-cell response; |
NA |
Exosomes yield stronger specific humoral responses than liposomes |
None |
Sub-cutaneous |
215
|
TTxd-Soluplus nano-aggregates |
68 nm |
Bacteria |
Soluplus polymeric micelles |
Tetanus toxoid (TTxd) |
Nano-vaccine increases antibody production |
Tetanus |
TTxd-Soluplus ↑ antibody response to TTxd |
None |
Trans-cutaneous |
216
|
Outer membrane vesicles + antigen +LPS |
NA |
NA |
Outer membrane vesicle (OMV) |
Antigen |
antigen program dendritic cells for cross-presentation to CD8+ T cells |
NA |
OMV → maturation of human monocyte-derived DC, murine bone marrow-derived DC, CD11c+ splenic DC |
DC/CD8+ T cells |
Intra-nasal |
217
|
Rabies vaccine with nano-sized aluminium adjuvant |
100 nm |
Virus |
Nano-sized aluminium |
Rabbies vaccine |
Enhanced antibody production with nano-sized aluminium |
Rabies virus |
Nano-sized aluminum → more neutralizing antibodies against rabies virus than other adjuvants (BLPs, AS02, AS03, MF59 and Poly I:C) |
None |
Intra-muscular, sub-cutaneous, intradermal |
218
|
NanoMn |
20–30 nm |
Virus |
Nano-sized manganese |
None |
Enhances interferon production |
COVID 19 |
Nano-Mn: • M1 macrophage polarization; • Monocytes recruited into inflammatory foci; • virus-specific memory T cells; → less coronavirus-induced tissue damage. Besides |
None |
Intra-peritoneal |
219
|
pH-Responsive biodegradable carbonate apatite (CA) encapsulating CpG ODN + antigen |
13–25 nm |
Virus/cancer |
Carbonate apatite NP + CPG (Cytosine-Phosphate-Guanine Oligodeoxynucleotides) |
Antigen (OVA) |
Enhance the production of type-I IFNs (such as IFN-α) |
Influenza |
Vaccination with CA-CpG + antigen: ↑ antigen-specific antibody responses; ↑ CD8+ cytotoxic T lymphocyte responses in vivo |
None |
Intra-venous |
45
|
AuSNP + FMD-VLP |
110 nm |
Virus |
Gold-star nanoparticles (AuSNP) |
Antigen: foot-and-mouth disease (FMD) virus like particle (VLP) |
↑ Macrophage activation |
Foot-and-mouth disease |
Better immune protection than with standard adjuvant (oil, ISA205) |
None |
Sub-cutaneous; Intra-dermal |
220
|
MWCNT non-covalently bound to CPG |
NA |
Bacteria |
Multi wall carbon nanotubes (MCNT) |
CPG |
↑ Cytokines |
Bacteria |
MWCNT + CpG: more efficient/less toxic than free CpG (lower dose necessary to protect animals against bacterial infection) |
None |
Intra-peritoneally |
221
|
Au clusters + HEVA |
5–30 nm |
Virus |
Au clusters |
Hepatitis E vaccine (HEVA) |
↑ Immune response due to gold clusters |
Hepatitis E |
↑ Antibody response → gold clusters aggregated vaccine; → gold clusters reduce toxicity of HEVA |
None |
Intra-peritoneally |
222
|
Cysteins + CPG + OVA (500 OVA per NP) |
50 nm |
Cancer |
Cysteins attached via disulfide bonds |
Antigen (OVA) |
↑ Immune response (more antigens) |
Cancer |
Nano-vaccine protects 70% of mice with B16-OVA melanoma; ↑ cytotoxic T lymphocytes |
None |
Sub-cutaneous |
223
|
GO@PEG/PEI + Ure B |
50–250 nm |
Cancer |
Graphene oxide (GO) coated with PEG and/or PEI |
Urease B (Ure B) |
GO serves as antigen carrier |
Cancer |
Nano-vaccine → maturation of DC + cytokine production via TLR activation |
None |
Intra-dermal |
26
|
DGBA + OVA + CPG |
60–80 nm |
Cancer |
DGBA: polyamidoamine dendrimer modified with guanidinobenzoic acid |
Antigen (OVA) |
Nano-vaccine favors antigen cross-presentation by DC |
Cancer |
Cellular immunity; Nano-vaccine combined with programmed cell death protein 1 (PD-1) checkpoint-blockade efficient against B16-OVA melanoma |
None |
Intra-dermal |
224
|
RGO@PEG + |
20–30 nm |
Cancer |
Reduced graphene oxide (RGO) coated with PEG |
NA |
Intra-cellular ROS in DC |
|
Nano-vaccine favors accumulation in LN + antigen processing/presentation to T cells |
None |
Sub-cutaneous |
32
|
LDH-A |
50–1000 nm |
NA |
Layered double hydroxide NP |
Antigen |
Activate macrophages + DC |
NA |
Macrophage cells exchange internalized LDH NP with external ones; Internalized LDH-antigen NP → facilitate DC maturation + antigen presentation to DC |
None |
Sub-cutaneous |
225
|
PLGA@CCM containing R837 + MM (mannose moiety) |
140–160 nm |
Cancer |
PLGA NP |
Antigen: cancer cell membrane + Imiquimod (R837): TLR agonist |
Enhanced DC activation |
Cancer |
Nano-vaccine: ↑ uptake by DC; DC maturation Efficacy increased with check point blockade therapy |
None |
Intra-dermal |
226
|
CCPS + DOX + HPPH |
50–120 nm |
Cancer |
Chimeric cross-linked polymersome (CCPS) |
DOX + HPPH (photosensitizer) |
Adjuvant reduces DOX/HPPH dose necessary against tumor |
Cancer |
Nano-vaccine: ↑ TAA and DC recruitment; In tumor bearing mice: ↑ mature DC in tumor lymph nodes and CD8+ T cells in tumor tissues; |
NA |
Intra-venous |
227
|
Liposomes containing P5 + HLA-DR + MPL |
120–140 nm |
Cancer |
Liposome |
P5 (peptide Derived from T cells) + HLA-Dr (peptide antigen of CD 4+) + MPL (agonist of TLR-4) |
↑ CD8+ + IFN-γ response |
Cancer |
Better anti-tumor activity/survival in tumor bearing mice compared with P5 |
NA |
Sub-cutaneous |
228
|
Microdisks containing antigens + TLRa |
683 nm (thickness) × 5000 nm (diameter) |
Cancer |
Microdisks |
Antigens + TLRa (Toll like receptor agonist) |
Co-delivery of antigens + TLRa |
Cancer |
Codelivery of antigens + TLRa DC activation depends on TLRa concentration; DC antigen presentation/processing depends on antigen concentration |
NA |
NA |
229
|
PSM + peptide/adjuvant |
NA |
Cancer |
PSM: porous silicon microparticle |
Peptide |
Effect of shape and admin route on localization/activation |
Cancer |
Effect of shape: spherical PSM accumulate in circulating DC Effect of admin. route: iv admin. PSM accumulate in spleen/inguinal lymph nodes; intra-dermally admin. PSM accumulate in popliteal lymph nodes |
NA |
Intra-venous/intra-dermal |
230
|
Lipid polymer NP + IMQ + MPLA + OVA |
220 nm |
Cancer |
Lipid polymer NP (MAN-IMNPS) |
Imiquimod (IMQ) + monophoryl A (MPLA) + Ovalbumin (OVA) |
Activation of DC |
Cancer |
Nano-vaccine in mice: • ↑ antigen-specific CD8+ T cells, ↑ lymphocyte activation, ↑ cross-presentation, ↑ memory T cells, ↑ antibody, ↑ IFN-γ, ↑ granzyme B; • → tumor growth delay and prolonged survival; • ↑ Anti-tumor effect with immune checkpoint blockade |
DC |
Sub-cutaneous |
231
|
Au NP + OVA |
15–100 nm |
NA |
Au NP |
OVA |
Prevents inactivation of vaccine by macrophages |
NA |
Subcapsular sinus macrophages (SSM) capture Nano-vaccine and prevent them from reaching lymph nodes and produce antibodies; by removing/inactivating SSM, ↑ production of antigen specific antibody by a factor of 60 |
SSM |
Intra-dermally |
232
|
Porous silica NP + proteins (VirB9-1 and VirB9-2) in pores |
50 nm |
Pathogen (Anaplasma marginale) |
Porous silica NP |
proteins (VirB9-1 and VirB9-2) |
Humor + cellular immune response |
Ana-plasmosis |
NP enable high antigen loading capacity; Antibody titres; T-cell response |
None |
Sub-cutaneous |
233
|
Fe3O4/T-MPs-CPG/Lipo (double vaccine/system) |
NA |
Cancer |
Fe3O4 NP + Liposome (Lipo) |
Tumor-derived antigenic microparticles (T-MPs) + CPG |
strong tumor antigen-specific host immune response |
Cancer |
Nano-vaccine: • change macrophage polarization in TME, ↑ T-cells; • ↑ anti-tumor efficacy with immune checkpoint blockade PD-L1 blockade (↑ survival of melanoma bearing mice) |
None |
Sub-cutaneous |
75
|
NanoZIF-8 containing Aluminium + antigen (OVA) + CPG |
80 nm |
Cancer |
NanoZIF-8 + alumium |
OVA + CPG (agonist of TLR-9) |
pH controlled release of antigen |
Cancer |
Nano-vaccine injected to tumor bearing mice: • Nano-vaccine targets lymph nodes and internalize in APC; • Antigen released in APC lyposome → ↑ cross-presentation; • ↑ antigen-specific antibody + T cells; • → Tumor growth delay |
None |
Sub-cutaneous |
179
|
Au NP (two size ranges: < 10 nm and > 10 nm) |
4.5 nm <10 nm (13, 30, 70 nm) >10 nm |
Cancer |
Au NP |
Au NP |
Type of immune activation depends on NP size |
Cancer |
• Au NP (<10 nm) activate NLRP3 inflammasome → Caspase-1 maturation + interleukin-1β production; • Au NP (>10 nm) activate NF-κB signaling pathway |
None |
Sub-cutaneous |
116
|
LZnP NP + TRP2180–188 + HPG10025–33 + MPLA |
30 nm |
Cancer |
Lipid coated zinc phosphate NP (LZnP NP) |
2 antigens (TRP2180-188 + HPG10025-33) |
Double antigenic system |
Cancer |
2 epitopes improve immune response; nano-vaccine: ↑ cytokines, ↑ T cells; nano-vaccine with 2 antigens more efficient than free antigens and nano-vaccine with 1 antigen |
None |
Sub-cutaneous |
12
|
To avoid any confusion, it may also be possible to define NM as an adjuvant by exclusion, i.e. consider that it represents any nanometric substance contained in a vaccine, which differs from the main/dominant active principle, i.e. essentially antigen or mRNA/DNA. Alternatively, a nano-adjuvant could designate a specific vaccine property, which would not be dominant, i.e. the vaccine might still work to the some extend in the absence of the nano-adjuvant but the latter helps the vaccine to reach its full efficacy. This presentation agrees with the separation, which is often made in the medical field between dominant and accessory effects, frequently for the sake of clarity to facilitate the understanding of the biological mechanisms involved. In any case, it is desirable to go beyond the simple definition of the adjuvant, which only conceives the adjuvant as a specific vaccine component and does not take into consideration some important properties, for example specific assemblies or interactions or transformations of the VAP, which can result from the presence of the nano-adjuvant or lead to the appearance of a property at nanometric scale and strongly enhance vaccine efficacy.
Considering the definition given above, NM contained in vaccines can generally be considered as adjuvants, except in the particular case where they act in a similar manner as the VAP, i.e. for example when they mimic an antigen or when an antigen or mRNA/DNA is not directly present in a nano-vaccine formulation.
II. Nano-dimensions in COVID 19 vaccines
Regarding the nanometric dimension in COVID-19 vaccines, it essentially appears in three different ways. First, the nanoscale structure can serve to protect and bring the vaccine active ingredient to the region where it can elicit an immune response against the virus, as it is the case with Pfizer/BioNTech vaccines, where mRNA is encapsulated in a liposome to allow mRNA cellular internalization followed by its processing, further yielding the production of the Spike protein. Second, it can consist of an elementary block of the vaccine, as it is the case for virus-based vaccines containing for example adenovirus or inactivated COVID-19 virus, where the nanometric elementary blocks serve to mimic the behavior of the COVID-19 virus, hence training the immune system to elicit an immune response against the real virus while avoiding its side effects. Third, it can result from the assembly at the nanometric scale of a certain quantity of active principles, e.g. DNA or proteins encoding or resembling parts of the COVID 19 virus, where such assembly could potentially allow a better activation of the immune system than active ingredients taken individually, which are isolated from each other.16 Following this presentation, the vaccine active principle appears to be either structurally distinct from the NM (first case), to correspond to the NM (second case), or to self-assemble in a NM (third case). In all three cases, the nanometric structure can be associated with an adjuvant function, either to allow the active principle to reach a target where it can act without being inactivated (first case), or to result in an activity, which results from its size, whether this size corresponds to that of individual blocks (second case) or assemblies (third case) of active principles, i.e. without such nanometric sizes, the active principles are no longer as effective.
III. Nano-adjuvants: at the crossroad between cancer and virus vaccines
As highlighted through a pubmed search, which enabled a field dependent article count, research on nano-vaccines appears to be rather limited and evenly distributed between cancer and viral applications before the COVID-19 crisis, while it has become more prominent during this crisis, resulting in a particularly large number of developments in the viral field. These trends are summarized in Fig. 1. By further comparing these two fields, NM appear at a crossroad of virology and oncology, since on the one hand NM sizes lie within the range of virus dimension and on the other hand NM can internalize in or interact with cancerous cells to destroy them. This schematic view helps to understand how similar NM could potentially be used in cancer and virus vaccines. Hence, the knowledge gained in the development of nano-vaccines against cancers could in part be used to offer a palette of efficient nano-vaccines against COVID-19. In a way, the COVID-19 crisis has shed some new light on nanotechnologies that appear as a meeting or rallying point between virologists and oncologists, who could unite their efforts together with nanotechnology specialists to fight the virus. This idea is further illustrated in the graphical abstract. In this context, I have chosen to present in this review NM, which have been tested in cancer vaccines, since they could potentially, after certain adjustments concerning the nature of the VAP, be added to COVID-19 vaccines.
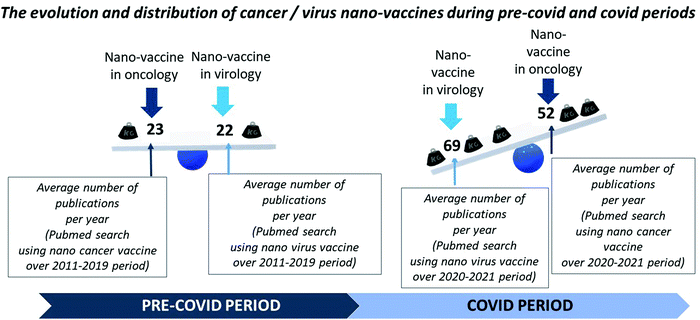 |
| Fig. 1 A schematic representation showing the distribution between the developments of nano-vaccines in oncology and in virology during the pre-covid and covid periods, where this diagram was obtained through a pubmed search that estimated the number of publications containing the terms nano, cancer, vaccine or nano, virus, vaccine and by deducing from this search the number of publications per year containing these terms over the periods 2011–2019 (pre-covid) and 2020–2021 (covid). | |
IV. How to improve the benefit/risk ratio and availability of Covid-19 vaccines by using nano-adjuvant
Through their mechanisms of action described in this article, e.g. protection of the antigen, increased stimulation of the immune system, facilitated presentation of antigens to APC, nano-adjuvants could improve the efficacy of vaccines against COVID 19 and reduce their toxicity, thus leading to a larger benefit/risk ratio. In addition, a challenge of a relatively new nature arose during this crisis, namely the ability to have access to enough vaccines to treat a sufficiently large percentage of the population to eradicate this virus. One way to achieve this objective is to produce vaccines which operate at a lower quantity of VAP, thus enabling a reduction of the therapeutic dose necessary for obtaining efficient vaccination, and thereby, for a given vaccine production, increase the number of efficient vaccine doses. Even if this link is not always fully demonstrated experimentally, it is conceivable that an improvement in the benefit/risk ratio such as that mentioned above would be accompanied by vaccine efficacy achieved for lower therapeutic dose.
The benefits of using nano-adjuvants, which are described throughout this review, should be balanced against the risks of using them. Concerning liposomes, their toxicity is minimal.17–19 One of the main risks associated with their use comes from their disintegration, e.g. if they are not stored under stabilizing conditions, hence resulting in the inactivation of the active principle, e.g. by yielding mRNA degradation by RNase.18 With regard to viruses or DNA active ingredients, the risks associated with their uses may arise from a loss of their nanometric structures through either partial/total degradation or disassembly, which can weaken their activity. Other risks may come from mistakes committed during mRNA and DNA fabrication, which prevent the production of the spike protein, or in the use of viral vaccines that don’t avoid side effects, e.g. because the inactivation method that was used did not yield a viral structure that is sufficiently different from COVID-19. When nano-adjuvants enable to reduce the dose of VAP in vaccines, they may prevent the presence of an unwanted immune reaction, such as an auto-immune.20 These risks can avoided through a proper design, production, and biosafety pre-clinical/clinical assessment of the nano-adjuvants, VAP and of their formulation.
V. The efficacy/toxicity of the different types of nanomaterials used as nano-adjuvants
The different types of NM used as vaccine adjuvants, whose list is provided in Fig. 2 and whose specific physico-chemical and anti-pathogenic properties are summarized in Table 1, are presented in the section below.
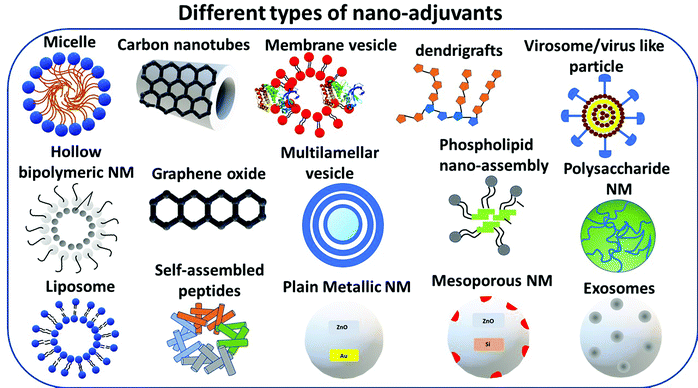 |
| Fig. 2 Nanomaterials of different compositions and assemblies that can be used as vaccine adjuvants, including micelles, carbon nanotubes, graphene oxide, dendrigrafts, virosomes, phospholipids nano-assemblies, polysaccharides, exosomes, mesoporous or plain nanomaterial (metallic or not), self-assembled peptides, liposomes, and hollow bi-polymeric NM. | |
V.1 Nanomaterials with specific carbon atom arrangements (graphene oxide and carbon nanotube).
Graphene oxide (GO) structure consists of a single two-dimensional crystalline layer containing sp2/sp3 hybridized carbon atoms organized within hexagonal networks, where the basal planes or edges of such layer are either largely covered by a series of oxygen functional groups such as hydroxyl, carbonyl, and epoxy groups, for non-reduced GO or not/partly covered by such groups for reduced GO (rGO).21 Whereas GO is usually obtained by oxidizing graphite, e.g. using Hummers’ methods,22 rGO results from the reduction of GO, often using a toxic chemical such as hydrazine hydrate.23 GO/rGO have further been coated or modified with various materials such as PEG to yield structures with improved hydrophilicity, solubility, stability, biocompatibility, and delivery efficacy compared with untreated structures.24 Two very interesting aspects are derived from GO/rGO geometry. First, the large surface of GO/rGO enables the non-covalent binding of a large quantity of antigens such as human amyloid peptide (Aβ) or glioma antigen (Ag).25 Second, such antigens could potentially be released in a controlled manner from GO/rGO surface through a transition from a folded to an un-folded state of the whole structure.26 A series of GO/rGO properties make this material particularly well suited for being used as nano-adjuvant. Firstly, GO/rGO apparently facilitates antigen cellular internalization through endocytosis via specific interactions between GO/rGO and cell membrane receptors involved in endocytosis,27 a behavior that can also be associated with the high aspect ratio of GO/rGO, which seems to facilitate GO/rGO insertion within the cell membrane. Secondly, the strength of immune stimulation can be tuned by varying the size of GO/rGO, i.e. it was shown that GO of 2 μm triggered a stronger immune response than GO of 350 nm.28 Thirdly, GO/rGO can protect the antigen from enzymatic digestion, by promoting intracellular antigen trafficking via the cytoplasm, hence avoiding lysosome degradation.29 Fourthly, it was demonstrated that GO/rGO could favor an immune response, by facilitating antigen cross-presentation to CD8+ T cells,28 by increasing the quantity of cytokines and chemokines produced by DC,26 by enhancing MHC-I expression, which is an essential step in the activation of CD8+ T cells,21 or by stimulating the release of proinflammatory factors by macrophages.30,31 In a very interesting study, mice suffering from B16F10 melanoma received a vaccine containing PEG/CPG functionalized rGO, where rGO apparently acted as the antigen. It led to the migration of rGO towards lymph nodes, the activation of DC, and resulted in tumor growth delay.32
Carbon nanotubes (CT) have also been suggested as cancer vaccine components.33 Carbon nanotubes (CNT) are made of cylinders consisting of rolled graphene layer(s) (Fig. 1),34 which are nanometer in width and nanometer to micrometer in length. They can be divided between single-walled CNT (SWNT) and multi-walled CNT (MWNT).35 As for GO, CNT properties can be tuned through various functionalization. While non-covalent functionalization, e.g. with carboxylic groups linked to amine functions,36 single stranded DNA (ssDNA),37 or bovine serum albumin,38 yielded CNT with improved hydrophilicity and aqueous dispersibility, covalent functionalization, e.g. with Tumor Proteins, resulted in an enhanced internalization of Tumor Proteins in DC.39 Under general terms, CNT functionalization appears to be a means for improving their biocompatibility and for tuning their biodistribution property in the organism. For example, by functionalizing MWNT with amine functions, these structures were individualized, thus facilitating their glomerular filtration and renal clearance.40 Cellular internalization of CNT was also reported to be more efficient than that of GO,41 potentially highlighting the role of specific carbon atom arrangement in the mechanisms of cellular internalization. Furthermore, internalization mechanisms were in some cases associated with endocytosis, as demonstrated for SWNT–streptavidin, which were observed by microscopy in endocytic vesicles and whose internalization was reduced at 4 °C.42 In some other cases, they were attributed to the passive diffusion of CNT through cell membrane, CNT playing the role of nano-needles inserted within the lipid bilayer, where they formed trans-membrane diffusing pores.42 Interestingly, this type of passive diffusion of MWNT was also observed in prokaryotic cells, which lack endocytosis mechanism, thus potentially paving the way towards the specific immune activation/inactivation of these micro-organisms by CNT.43 CNT immunogenicity can be harnessed to efficiently fight pathogenic cells, by taking advantage of the large surface area of CNT, and the various functionalization that they enable, which allow the attachment to CNT surface of many different types of immunogenic substances such as antigens, TLR agonists, CPG, αCD40, and anti-PD-1. Such multi-functional structure whose appeal is strengthened by its propensity for internalization has been shown to trigger certain specific immune mechanisms such as the cytolytic action of CD8+ T cells against pathogenic cells, which is favored by antigen cross presentation in DC.44 As an example of preclinical study, it was shown that mice were protected against bacterial infection at a lower therapeutic dose when they received MWCNT-CpG than free CPG, revealing the interest of using this type of NM for reducing the amount of active principle that needs to be administered to reach an effective treatment.45 As for GO, reassuring preclinical safety data of CNT are pending. They appear as a prerequisite to be able to start clinical assessment of these materials.
V.2 Vesicles (micelles, liposomes, membrane vesicles, exosomes).
Micelles.
Micelles have also been suggested as vaccine adjuvant.46 They are core/shell NP resulting from spontaneous self-assembly in water of individual amphiphilic molecules, i.e. molecules displaying a hydrophobic tail and a hydrophilic head. They are composed of various polymer/copolymer assemblies, e.g. PLA-b-P(NAS-co-NVP),47 γ-PGA associated with hydrophobic cholesterol,48 PEG-PLL-PLLeu,49,50 γ-PGA polymer modified with hydrophobic L-phenylalanine ethyl-ester,51 chitosan combined with hydrophobic phenyl alanine,52 PEI linked to stearic acid.53 Substances of therapeutic interest can be bound to the micelle external surface or be incorporated inside micelle core, e.g. HIV-1 Gag p24 antigens were attached to the micelle surface while TLR-l ligand (imiquimod) was encapsulated inside the micelle core.47 To maintain such substances inside the micelles, the stability of the whole structures needs to be maintained. For that, it can be taken advantage of electrostatic interactions, e.g. between anionic DNA or mRNA and cationic PEI external micelle external surface,51,54 Furthermore, in addition to antigens, a wide choice of other vaccine substances can be associated with micelles, such as siRNA, mRNA, or TLR agonist such as PIC,49–51,54 allowing such structures to be multi-functional. Other appealing micelle properties should facilitate their use as vaccine adjuvants such as tunable micelle surface properties (composition and charge) to achieve biocompatibility and hydrophilicity, a nanometric size that facilitates micelle migration to lymph nodes and once there, antigen delivery to DC.55 This results in better stimulation of the immune system when the antigens are associated with micelles than when they are free, i.e. in particular an enhanced stimulation/maturation of DC, followed by effective T cell activation and proliferation, a higher level of IgG/IgA antibody titers specific of the antigen associated with the micelles.47,49,50 The efficacy of these mechanisms seems to depend on certain micellar properties such as their size, i.e. DC maturation was observed to be stronger for smaller micelles,51 and their ability to release antigen in a controlled manner in lymph nodes under pH variation.56 Among the noticeable pre-clinical data, striking results were reported on mice bearing B16F10-OVA melanoma, which received a nano-emulsion encapsulating antigens with TLR-7/TLR-8 agonists. This treatment did not only lead to common lymphocyte activation against the tumor but also to the re-programming of immunosuppressive tumor micro-environment (TME), i.e. a change in macrophage polarization from M2 to M1, which was concomitant with tumor growth delay.57
Liposomes.
Liposomes are spheres comprising an aqueous core surrounded by phospholipid bilayers, made of different types of amphiphilic phospholipids, such as PC (phosphatidylcholine), PS (phosphatidylserine) and SM (sphingomyelin), which may also be combined with other lipids, such as CHO (cholesterol) or polymers (PEG), to stabilize the liposome membrane and adjust its permeabilization.58 A rather large range of different types of liposomes have been developed comprising deformable vesicles (Transfersome),59 inter-bilayer cross-linked multilamellar vesicles (ICMVs),60 solid core liposomes,61 small unilamellar vesicles (SUV) with sizes below 200 nm, large unilamellar vesicles (LUV) with sizes comprised between 200 and 500 nm, microsized multilamellar vesicles (MLV) consisting of numerous concentric phospholipid bilayers with sizes above 1 μm, virosomes containing an envelope made of viral phospholipids and viral proteins, e.g. spike glycoproteins, niosomes, e.g., see ref. 62 and bilosomes, consisting of bilayer vesicles incorporating bile salts.63 Under general terms, liposomes can either be in a liquid or solid state, depending on whether their transition temperature between these two states Tc is above or below ambient temperature, i.e. when Tc < 25 °C liposomes are in a liquid state while when Tc > 25 °C liposomes are in a solid state. The value of Tc itself depends on certain properties of the phospholipids such as their carbon chain length, their number of unsaturation, their nature, as well as the different types of interactions taking place within the phospholipid membrane. Membrane fluidity is an important aspect determining liposome adjuvant efficacy, i.e. liposomes with Tc of 55 °C encapsulating antigens (Leishmania donovani) yielded improved adjuvant activity in mice,64 while those with Tc of 23 °C or 41 °C loaded with antigens failed to act as adjuvants and to efficiently protect infected mice.64 Two schematic visions of the way in which liposomes work can be presented. First, it can be considered that liposomes disintegrate or fall apart, e.g. when their solid–liquid transition occurs or when they encounter certain types of biological material, and that this event results in liposomes becoming therapeutically active, e.g. through the release of their content. Second, one can believe that liposomes protect the active principle, which remains linked in one way or another to the liposomal structure until it reaches the location or condition where it becomes fully active. It is hard to determine experimentally in vivo which type of mechanism is really taking place. One of the main interest of liposomes comes from the various parameters, which can be adjusted to optimize their therapeutic properties. First, they can be linked at their surface to molecules with two different specific functions, i.e. compounds such as PEG that enable them to avoid being captured by the immune system (macrophages),65 and substances such as mannose, which can bind to certain cell type receptors, e.g. mannose receptor, hence improving liposome targeting efficacy.66 Second, it is possible to select among the different types of liposomes, a structure that will release the active principle slowly (MLV,67), which seems favorable to reach a sustained immune response and achieve efficient vaccination. Third, liposomal structures are under certain conditions able to carry together molecules with various functions, e.g. antigens, PRR, Aluminum NP, potentially enabling to tune their immunogenic activity through the selection of the right cocktail of immunogenic entities that they incorporate.61,68,69 Fourth, liposome surface charges can be adjusted by accurately selecting phospholipids, the charged phospholipids enabling to encapsulate in their core or to adsorb at their surface antigens of opposite charges. The resulting electrostatic interactions are believed to yield sustained/non immediate antigen release, a desired property when a long-lasting pharmaceutic effect is sought for.70 Comparing negatively with positively charged phospholipids, it can be concluded that cationic phospholipids are more likely to interact with the negatively charged cellular membrane and to trigger an immune response that differs from that of anionic phospholipids, i.e. anionic liposomes where shown to more efficiently yield a Th1 responses in mice than cationic liposomes.71 Fifth, liposome size can influence vaccine efficacy since some mechanisms of diffusion/transport and interaction with the immune system are size dependent. For example, liposomes smaller than 20 nm may enter systemic circulation, while those of sizes between 20 and 100 nm may preferentially diffuse towards draining lymph nodes, and those of sizes larger than 100 nm may remain at the injection site where they form a depot. This can further result in size dependent immune reactions, e.g. IL-12 cytokine production was observed for liposomes larger than 225 nm while such behavior was not reported for liposomes smaller than 155 nm.72 Sixth, liposomes offer different modes of associations of antigens, i.e. antigen adsorption or covalent binding on/at liposome surface, antigen encapsulation within its inner core, or antigen intercalation between adjacent bilayers. This enables associating liposomes to antigens in a way, which depends on antigen properties, i.e. water-soluble antigens can be inserted in the liposome aqueous inner core, lipophilic antigens can be intercalated into the lipid bilayer, and other antigen types can be attached to the liposome surface. Although the type of association chosen shall have an impact on the immune response, this aspect, which would require a comparative experimental study between the different types of antigen association, does not seem to have been thoroughly studied. A series of striking adjuvant-like behaviors were reported pre-clinically with different types of liposomes. They include the migration to lymph nodes and the triggering of antigen specific antibody (IgA) responses in mice immunized with 200–7000 nm PEG stabilized liposomes comprising retinoic acid and CAF23 antigens,73 multi-epitope T cells response and tumor growth delay following injection to melanoma bearing mice of 20–30 nm multi-functional liposomes consisting of a phosphate calcium core surrounded by cancer membrane proteins (CMP) and α-helix HSP70 functional peptide,74 and a change in TME macrophage polarization after administration to melanoma bearing mice of a complex structure consisting of liposomes associated with Fe3O4 NP, CPG, and antigenic microparticles.75 The properties of the various lipid nanoparticles used for delivering mRNA in mRNA vaccines are described in recent reviews.17–19
Exosome.
On the one hand, exosomes display several properties in common with liposomes such as a relatively similar round shape, a surrounding envelope made of phospholipids and a size of 30 to 120 nm. On the other hand, they are also characterized by a series of distinctive features. First, they are released from late endosomes of eukaryotic cells. Second, their lumen or surface content is made of various biomaterials such as specific tetraspanin proteins (CD9, CD63, CD81, CD82), nucleic acids, DNA, lipids, carbohydrate, and various types of RNA, e.g. mRNA, RNAs, siRNA, piRNA, microRNA.76–78 Third, they possess the specific function of facilitating the diffusion of their biomaterial between different cells,79,80 thereby providing a mean for cell function regulation. In this regard, exosomes appear in some cases to yield an immunosuppressive activity that can prevent tumors or pathogens from being destroyed by the immune system.81 In some other cases, they may contain the antigens released by antigen-presenting cells (APCs), dendritic cells (DCs), and then be used in vaccines.82 With regard to the vaccinal aspect, the case of exosomes is unique since without subsequent modification of their content in active principle following their expulsion from cells, their active compound (antigen, RNA) is incorporated in their envelope, possibly during the maturation phase of the exosomes in the endosomes.83 By contrast to other adjuvants, in the case of exosomes, the appearance of the medically active substance takes place intracellularly more or less concomitantly with that of the adjuvant. In exosomes, due to a cell-dependent genesis, the adjuvant and active principle appear to be intrinsically linked with each other, a situation that is not so common in other vaccine components.
Membrane vesicle.
A type of extracellular vesicle with a different origin from that of exosomes has been proposed as adjuvant.84–86 It consists of outer membrane vesicles (OMV). The latter are derived from Gram negative bacteria and are characterized by a diameter lying between 20 to 250 nm depending on bacterial species.87 They consist of a surrounding outer membrane composed of phospholipid such as LPS, proteins, which encapsulates periplasmic components.87 They usually require bio-engineering steps to have the proper antigens expressed or incorporated in OMV and to prevent a too high level of immune reaction that can be due to the presence of LPS or other immune components, and can result in sceptic shock.84,88 These steps can consist in modifying or adjusting the genome of the bacteria.89 Antigens can either be inserted in OMV lumen or displayed on OMV surface.84 Considering a simplified schematic view, both types of configurations present advantages and drawbacks. On the one hand surface exposed antigens may be more favorably accessible for antigen presenting cells (APC) but can also more easily be captured/destroyed by certain immune cells such as macrophages. The opposite holds for antigens in lumen, which could less freely interact with APC due to the phospholipidic layer separating them from APC, but this layer also protects them against immune degradation. For this reason, the choice between extra or intra vesicle antigen encapsulation or association must be made on a case-by-case basis, depending on certain vaccination parameters such as the vaccine mode of action or administration route. When OMV containing influenza antigen and a low endotoxin content were administered to mice via the intra-nasal route, it resulted in IgA antibody production, the activation of lung-resident influenza-specific T cells, and a certain level of protection against influenza among immunized mice.90
V.3 Polymer.
A large number of vaccine nano-adjuvants have been inserted in the category of polymers due to the broad definition of this type of material, i.e. structures composed of repetitive molecular or atomic sub-units.91–94 Since polymer structures are not necessarily characterized by the presence of a full symmetry or periodicity, it can in some cases be difficult to determine if a nanomaterial is polymeric or not. The polymeric nano-adjuvants described in the literature consist of DNA based structures, FDA approved poly(D,L-lactic-co-glycolic acid) (PLGA), poly(D,L-lactic-co-hydroxymethyl glycolic acid) (pLHMGA), poly(L-lactic acid) (PLA), poly(glycolic acid) (PGA), polystyrene (PS), poly(propylene sulfide) (PPS), polyethyleneimine (PEI), a series of different types of polysaccharides, such as chitosan, dextran, hyaluronic acid, starch, alginate, pullulan, and inulin.91–96 Polymeric structures are also characterized by a series of different types of arrangements such as: (i) co-polymers or the assembly of several different polymers within one structure, i.e. PEG-b-PPS amphiphile block copolymer,97 (ii) nanogels engulfing a polymer within a nanometric matrix structure,98 (iii) polymersomes that consist of self-assembled block copolymers,99 (iv) dendrimers or dendrigraft, which are polymers arranged in branches, sometimes symmetrically around a core.100–102 Antigens can be associated with polymers either via electrostatic interactions, e.g. positively charged polymers interact with negatively charged nucleotide, or through antigen encapsulation into polymeric nanoparticles.103 In general, it is possible to select polymers that are bio-degradable, bio-compatible, easily manufacturable, and easily soluble,104 and avoid some polymers such as PEI that can induce a certain level of toxicity.105 Furthermore, the natural origin of polymer can be a pledge for their biocompatibility.106 Polymer physico-chemical properties also impact their effect on the immune system, i.e. non-spherical nano-polymers display reduced uptake by APC compared with spherical ones,107,108 while positively charged nano-polymers are usually reported to be more efficiently internalized in APC than negatively charged particles due to the negatively charged membrane.109 In general, the association antigen/polymer is described as promoting antigen release as well as antigen association with other molecules of vaccinal interest such as CPG.110 Finally, several studies also reported the use of nano-polymers to make vaccination at mucosal level more efficient than with traditional vaccines. This region of the body is of particular interest for vaccination since a stimulation of the immune system at this location can potentially prevent viruses from entering the body.107,111
V.4 Metallic NM (plain or mesoporous).
NM with different metallic compositions, e.g. ZnO,112 or Au,113 have been suggested as vaccine adjuvant, and the use of Au NP in nano-vaccines has recently been reviewed.114,115 Their main interest lies in their adjustable physico-chemical properties, such as their sizes, shapes, charges, which can be tuned by varying their synthesis conditions such as the types and concentrations of the used reagents, as well as pH, temperature, and duration of the reactions. Concerning Au NM, it has notably been reported that a subcutaneous administration of such material with the OVA adjuvant resulted in immune mechanisms, which depended upon Au NP sizes, i.e. the smallest Au NP (<10 nm) activated NLRP3 inflammasome, caspase-1, and led to IL-1β production while the largest Au NP (>10 nm) triggered the activation of the NF-κB signaling pathway, demonstrating the possibility to tune the nature of the immune response by varying NM sizes, and hence possibly to choose the most efficient immune response against pathogens or pathogenic cells by selecting the optimal size of the Au NM contained in a vaccine as adjuvant.116 By contrast to the previously described NM, which are plain, mesoporous ZnO NM could also be synthesized and used as vaccine adjuvant. This type of structure is particularly interestingly since it enables inserting antigens (OVA) inside the mesoporous pores through a mechanism, which seems to rely on electrostatic interactions between the positively charged surface of the pores and the negatively charged OVA. It not only leads a high a loading efficacy, but also enables a slow release of the antigen between ∼5 h and ∼72 h following antigen insertion. Considering this type of structure from a visual and schematic point of view, it seems that it gathers together the faculty to protect the antigen against degradation through the partial opening of its pores and the ability to present the antigen to APC and its receptors (e.g. TLR) by allowing local interactions between antigens and APC receptors at NM openings. The pores should be finely designed so as not to block interactions with APC. Thus, the mouse administration of ZnO NM mentioned above loaded with the OVA antigen led to an immune reaction characterized by the activation of CD4+ and CD8+ T cells, the production of IgG2a, IgG2b, IFN-γ, and a Th1 immune response.117 Furthermore, the reaction of the immune system may be orientated in a specific direction by modifying the surface of this type of NM, e.g. by varying their charge through pH adjustment, with negative and positive charges obtained at high and low pH, respectively,112 or the nature of the coating material used to stabilize metallic NM, for example using black pepper extract.113
V.5 Mesoporous silica NM.
Mesoporous silica NM may possibly be used as nano-adjuvants, due to the various well-established methods that can be used to synthesize them, their relative biocompatibility, and their faculty to release an active principle from their pores in a controlled fashion under the application of a stimulus, e.g. a variation in pH or temperature, as reviewed elsewhere in more details.118
V.6 Cell mediated vaccines.
Although the cell size is micrometric, some cell-derived compounds (proteins, e.g. cytokines, exosomes…) or certain cell-triggered mechanisms that occur at nanometric scale, may either directly be inserted in COVID-19 vaccines or used to design a therapeutic substance such as an antibody acting against COVID-19.119 Under this hypothesis, cell-mediated vaccines may fall within the classification of nano-vaccines. First, exosomes, in particular those derived from mesenchymal stem cells, can act as nanosized lipid bilayer nano-systems, enabling the communication between infected and non-infected cells, and then possibly reduce the cytokine storm and/or increase/restore mitochondrial function in injured lungs.120 Second, certain proteins fabricated by dendritic cells such as DC-SIGN and DC-SIGNR, which are believed to promote COVID-19 infection, could be neutralized by nano-vaccines triggering an antibody activation against these proteins.121 Third, certain stem cells, in particular mesenchymal ones, could act against the cytokine storm resulting from COVID 19 infection, e.g. by yielding cytokines displaying regulatory instead of inflammatory immune properties.122
VI. Mechanisms of action of nano adjuvants
NM can be perceived as platforms serving as support to anchor not only VAP, but also other immune entities such as CPG, TLR agonist, enabling the concentration of these compounds, their protection against degradation, the controlled activation or release of the VAP from the platform, notably by breaking the bonds between the platform and the VAP, the mimicking of viruses through the platform appearance and composition, as illustrated in Fig. 3. These properties result in an efficient response of the immune system against pathogens, through the stimulation of APC, essentially dendritic and B cells, and involve different types of cells depending on the activated pathway, i.e. CD4+, CD8+, Th1, Th2, Th17, plasma B cells, and either the production of antibodies (IgA, IgE, IgG, IgM, IgD) or interleukines, e.g. IL-2 or IL-4, or interferons, e.g. IFN-γ, as summarized in Fig. 4.123–125 In some cases, these immune entities directly participate in the destruction of viruses as it is the case with cytotoxic T lymphocyte through the production of perforin or granzyme, or with antibodies. In some other cases, they indirectly trigger anti-pathogenic activity as it is the case for Th cells, certain cytokines, which would activate other immune cells to capture pathogens.125 It is also well-known that some of the activated immune cells such as Treg cells can weaken the response of the immune system against pathogens, necessitating that the immune system is activated selectively to stimulate immune cells or immune entities fulfilling the desired function.126Fig. 5 further illustrates how this idea can be implemented by protecting NM to avoid their capture by macrophages that would destroy them and their associated VAP (Fig. 5a) while enabling activation of CD4+ and CD8+ when the VAP is either mRNA or an antigen and the pathways involve either MHC-I or MHC-II (Fig. 5(b)).
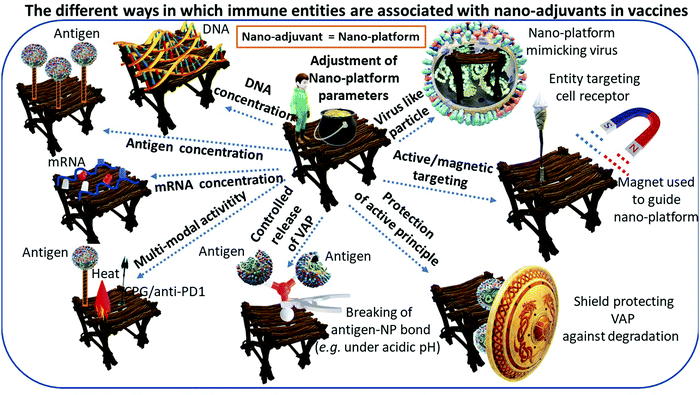 |
| Fig. 3 The different ways in which immune entities/VAP are associated with the nano-adjuvant. Nano-adjuvants can be represented as platforms, which can concentrate VAP, gather several different types of immune entities, control VAP activation in a controlled manner for example by breaking the bonds between the antigen and NM under certain specific conditions, protect the VAP, enable targeting of specific regions/cells by using an entity attached to the NM that specifically target a cell receptor or through magnetic targeting, or reproduce through NM shapes or compositions the behaviors of a virus. | |
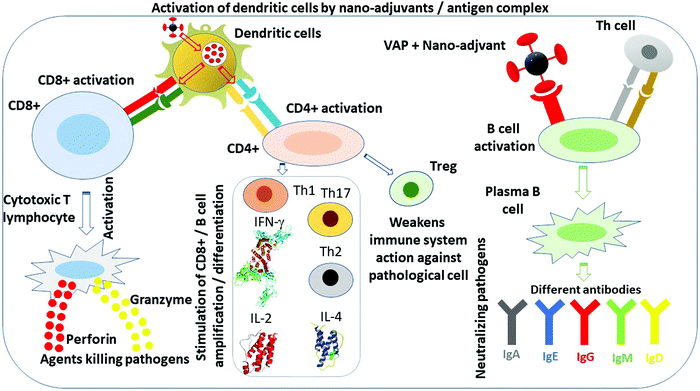 |
| Fig. 4 Different possible activations of antigen-presenting cells, i.e. essentially dendritic and B cells, using nano-adjuvants, involving various cells, e.g. CD4+, CD8+, Th1, Th17, Th2, plasma B cells, as well as other immune entities such as antibodies (IgA, IgE, IgG, IgM, IgD), interleukins (IL-2, IL-4) and interferons, e.g. INF-γ. | |
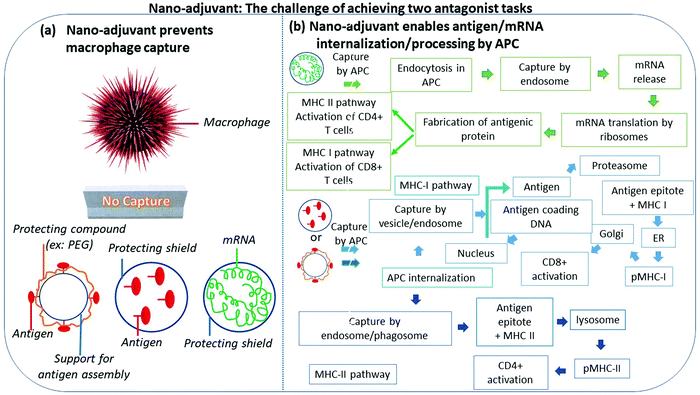 |
| Fig. 5 A figure highlighting the challenge of achieving two antagonist tasks with nano-adjuvants. On the one hand, (a) shows that NM should prevent the capture/degradation by some immune cells such as macrophages of the VAP using a protecting shield surrounding the NM or the association with the NM of a compound such as PEG that prevents such event from occurring. On the other hand, (b) presents the ways in which nano-adjuvants and associated VAP should be presented or internalized in/to APC and further processed through the MHC-I or MHC-II pathways, to yield CD4+ and CD8+ activations. | |
Nano-vaccines are designed to target essentially three regions. The first one consists of mucus, located in aerodigestive and urogenital tracts, eye conjunctiva, the inner ear and the ducts of allexocrine, where an immune response takes place in so called mucosa-associated lymphoid tissue (MALT), essentially through lymphocyte or IgA antibody production.100,111 Certain types of nanoparticles, such as positively charged ones, have been reported to enhance the immune response at mucosal site.127 Vaccination in mucous membranes using nanoparticles is essentially described to fight viral infection.128 In this case, it is designed to prevent pathogens from entering the organism through mucosal surfaces. Although more rarely employed, this route of vaccine administration has also been suggested against cancer, often using intranasal administration.129 For example, when emulsions of squalene-based NP of ∼200 nm containing OVA were introduced in mouse mucus, it led to the recruitment of antigen-specific T-cells, not only in the injection region but also at some distance of this location such as in the spleen, further resulting in an antitumor activity characterized by the survival of 50% of mice bearing lymphoma tumors 40 days after tumor inoculation.130 The second region of immune interest comprises lymph nodes, where APC migrate and lymphocyte priming occurs.131 Nanomaterials can favor the diffusion of antigens associated with them towards lymph nodes. Indeed, the size of the NM/antigen complex can enable the transport of this complex through the lymphatic capillary network,132 and then antigen processing by APC in lymph nodes. To achieve such mechanism, it seems that the immune response can be triggered against tumors or viruses, using various types of nanomaterials with the antigen depending by definition on the nature of the pathological agent. The third targeted region consists of a site, which is a well irrigated site such as the muscle,133 and/or a site of infection such as a tumor.134 In case where this type of targeting is planned, NM can allow an efficient diffusion of the antigens in the infected zone, i.e. by using a targeting molecule that drives the NM/antigen complex towards this region, or through NM passive diffusion, which enables NM, due to their sizes, to cross the holes of the angiogenetic blood vessels supplying the tumor with oxygen.135
The use of NM in vaccines can help protecting antigens against degradation or destruction.136 Whether the targeted disease is cancer or viral infection, the undertaking of such precaution is essential to achieve successful vaccination. It can be accomplished by encapsulating the antigen in a closed structure such as the inner aqueous core or the outer membrane of a liposome or a vesicle, or by attaching the antigen on the outer surface of a NM. The notion of non-degradation of the antigen shall be understood without forgetting that the antigen must also be internalized in APC to be presented to the MHC. In other words, NM are expected to allow selective antigen cellular interaction by allowing an absence of capture by certain cells such as macrophages, which could destroy antigens, while enabling internalization in other cells such as APC, which would allow antigen processing and further activate the immune system against pathogens. This selectivity, which could be achieved by a proper design of NM, e.g. using a coating material such as PEG that would prevent NM engulfment by macrophages,137,138 by attaching molecules such as glycospingolipid, which specially recognize APC cellular receptors,139 seems to be at the heart of a successful vaccination.
Under the general umbrella of vaccination principle, i.e. inducing the presence of a compound in the body which mimics certain pathological behaviors, hence training the immune system to fight against pathological cells and making it ready to prevent infection when it occurs, a large number of declinations can be considered, taking shape with nano-vaccines. The latter contain different types of antigenic proteins or other structures (mRNA and DNA) having the genetic code enabling antigen fabrication. Proteins, mRNA and DNA are either self-assembled at nanometer scale, are contained in particles mimicking a virus through their shape, i.e. VLP,140 or are associated with various types of NM (vesicles, polymers, polysaccharide, liposomes). mRNA and DNA present the advantage of potentially enabling antigen fabrication for all types of pathogens once their genetic codes have been identified. mRNA can be preferred to DNA since it may be more immunogenic and in the process of antigen fabrication, mRNA seems to act at less upstream steps than DNA, i.e. mRNA is issued through transcription from DNA. This comparison does not however take into consideration the fragility of mRNA and the whole set of cellular mechanisms involved in antigen fabrication, which are still largely unelucidated.141 Whether nano-vaccines contain the antigens or their genetic code, APC involvement appears necessary to yield antigen processing, which then triggers the reaction of the immune system against pathogens. Therefore, NM properties should be tuned or chosen to reach an optimal interaction with the APC.
Another interest of NM resides in their faculty to be associated with other kinds of immunogenic substances than antigens. A first type of such substances is designed to recognize pathogen recognition receptors (PRR) such as toll like receptors (TLR) located at the surface of APC. In this case, they either consist of pathogen associated molecular patterns (PAMP) originating from viruses or danger associated molecular patterns (DAMP) coming from tumors depending on the type of targeted disease. Examples of such substances include imiquimod, detoxified LPS such as MPLA, or CPG, which are agonists of TLR7, TLR4, and TLR9, respectively.142 It has been reported that the association of such substance with NM can favor the interaction between PRR and PRR agonist, hence preventing an uncontrolled immune reaction. This is achieved first by enabling such interaction to take place locally at PRR site, second by controlling the type of association between the antigen and the NM, i.e. the antigen is either adsorbed at or covalently linked to NM surface or the antigen is encapsulated within the NM, or third by triggering antigen release or dissociation from NM at a specific location of interest under physico-chemical disturbance, e.g. inside a cellular compartment characterized by an acidic environment. Furthermore, by using NM it may also be possible to associate a specific PRR agonists with an antigen within the same unit, hence potentially orientating the response of the immune system in a desired direction.143 For example, by choosing TLR4 as PRR, a T cell response may be triggered, while by selecting TLR2 as PRR a Th2 response may be expected.144 A second type of such substances is used to prevent the weakening of the immune response, which can occur at the site of infection, for example when T-cell activation by APC is blocked by so-called PD-1 receptors. To prevent the latter situation from occurring, anti-PD1 molecules have been associated with NM, leading to the successful neutralization of PD-1.145
Furthermore, by introducing NM in vaccines, it is feasible to tune vaccine immunogenicity by skillfully choosing the physicochemical properties of NM such as their sizes, shapes, charges, and hydrophobicity. It should be borne in mind that the immune response usually depends on the effects on several of these parameters as well as on other factors such as NM composition, concentration, route of administration, and the nature of the antigen associated with them. Thus, while the analysis of each of these parameters taken individually helps to better understand how nano-vaccines operate, the observed behaviors remain linked to specific experimental situations, and their generalization to other types of circumstances is hypothetical. Regarding sizes, it has been suggested that the type of region containing dendritic cells, which is targeted depends on NM size, with NM of 10–100 nm targeting Lymph node-resident dendritic cells, and NM larger than 500 nm targeting peripheral tissue-resident dendritic cells,146 where they can induce adaptive T and B (humoral) cell immunity.146 The type of cells by which NM are captured also seems to depend on NM size, i.e. for example smaller NM were reported to internalize in DC while larger NM were engulfed in macrophages.147 Efficacy of cross-presentation can also be influenced by NM size and in some cases increase with decreasing NM sizes,148,149 due for example to the smaller NM more easily escaping from endosomes to cytosol than larger NM.148 Concerning NM shape, it can also determine the efficacy of NM cellular internalization, i.e. anisotropic NM such as rods were more efficiently captured by macrophages and DC than spherical or cubic NM.150 With regard to NM charge, it is well-established that the positively charged NM interact electrostatically with the negatively charged cell membrane, a behavior that can potentially enhance the immune response, e.g. by resulting in a more efficient activation of B/T cells using positively than negatively charged NM.151,152 Interestingly, NM hydrophobicity has been reported to trigger a certain type of danger signal that activates the innate immune system,153,154 and therefore improves the strength of the immune response.155,156 Such observation has led to the development and use of polyanydride NM, which are very hydrophobic, and therefore prone to this type of behaviors.157
Different types of vaccines containing essentially non-immunogenic nano-adjuvant such as SPIO, polysaccharide, polymer, CaP, liposome, associated with various types of agents activating/targeting immune cells such as folate, IgG, anti-inflammatory drugs, TLR ligands or agonists, antibodies, antigens, were developed. The immune responses that they triggered depended on the types of nano-adjuvant and targeting/active principles, as well as on the way in which these two entities were associated with each other and interacting with the external environment, e.g. by being sensitive or responsive to a specific stimulus such as pH or temperature variation. It was characterized by the production of cytokines, a T-cell or humoral driven immune response, the activation of APC (dendritic cells and/or macrophages), and the involvement of certain co-stimulatory molecules, as summarized in Table 2 and associated references.
Table 2 Illustrative examples of immune responses triggered by various vaccines containing nano-adjuvants, where Table 2 indicates for each chosen nano-vaccine the treatment purpose, the active/targeting compounds and non-immunogenic nano-adjuvant contained in the vaccine, the nano-adjuvant size, various immune mechanisms triggered by the vaccine involving cytokines, lymphocytes, macrophages, dendritic cells, and co-stimulatory molecules. Here, a non-immunogenic adjuvant is an adjuvant that does not contain a compound that is usually considered as belonging to the immune system
Treatment (purpose) |
Active/targeting compound |
Non-immunogenic nanoadjuvant |
Nano-adjuvant size |
Immune mechanism involved |
Cytokine (TNF-α, IFN-γ, IL-12…) |
Lymphocyte (CD4+; CD8+) antibodies |
Dendritic cells |
Macrophages/monocytes |
Costimulatory molecules (CD80+; CD86+…) |
Ref. |
iv admin. to mice |
IgG coating |
SPIO |
175–200 nm |
NA |
NA |
NA |
NP accumulation in monocyte/macrophage derived monocyte → enhanced by IgG |
NA |
234
|
Anti-inflammatory activity in rats with arthritis; |
Folate |
Dendrimer |
NA |
NA |
NA |
NA |
Receptor-specific internalization in macrophages; such as ankle swelling, paw volume, cartilage damage, bone resorption, and body weight decrease. |
NA |
235
|
Treatment of obese mice |
Anti-inflammatory drugs |
Nano-scale polysaccharide |
4–30 nm |
Reduction in pro-inflammatory cytokines |
NA |
NA |
Association with M1 macrophages: ↓ pro-inflammatory cytokine |
NA |
236
|
Delivery of NP-vaccines to DC in mice |
mAb targeting CD40, CD11c, DEC-205; + TLR3/TLR7 ligands |
PEG-PLGA NP |
190–250 nm |
↑ IL-12 production; ↑ IFN-y |
CD8+ activation |
activation of DC |
NA |
CD40, CD11c, DEC-205 |
237
|
Melanoma treatment in mice |
TLR ligands (Poly:IC + CPG) + Mannose + melanoma Ag |
PLGA NP |
150 nm |
High IgG2c/IgG1 ratios and high levels of IFN-γ and IL-2. |
Th1 response; ↑ IgG2c/IgG1 |
Targeted |
Targeted |
Mannone: target MR/CD206 Expressed by DCs and macrophages |
238
|
Immunization of mice with nano-vaccine: ↑ CD8+ |
Ovalbumin |
pH-Sensitive polyacrylic micelles |
25–30 nm |
NA |
CD8+ T cell activation |
Membrane disruption of dendritic cells; conjugation of ovalbumin to the micelles significantly enhanced antigen cross-presentation |
Macrophage uptake of nano-system |
NA |
239
|
Reduction of E.G7-OVA tumor burden in mice |
Ovalbumin |
EPC/DOPE/polymer liposomes |
100 nm |
NA |
Nano-vaccine: cytotoxic T cells |
Fusion with the dendritic cell membrane; destabilization endosomes (pH variation) |
NA |
NA |
240
|
Immunization of mice bearing melanoma tumors: tumor growth delay |
Ag + TLR agonist |
Liposomes |
NA |
Cytokine produced by CD8+ |
CD4+ and CD8+ T cell responses |
Activation of TLR3 or TLR9 in dendritic cells |
Macrophages/monocytes: ⇒ transport of nano-system to lymph nodes |
NA |
241
|
Applications in preventive and therapeutic vaccines |
TLR7/8 a (resiquimod) + TLR9a (CpG) |
Polymer |
800 nm |
Nano-system favors lymph node production of cytokines (IL-12, IP-10 and IFNs) |
TH1 CD4+ and CD8+ T cell immunity |
Activation of endosomal TLR7/8 in dendritic cells |
Nano-system sent to lymph nodes by migratory/monocyte derived dendritic cells |
↑ Agonist density: higher expression of co-stimulatory molecules by macrophages/monocytes |
242
|
Nano-system for humoral immunity |
Ag: Hen Egg Lysozyme |
CaP NP |
250 nm |
NA |
NS interact with HEL-specific B-cell receptor |
NA |
NA |
NA |
243
|
Mice administered with nano-system |
Antigen bovine serum albumin |
DMPC/Chol/DPPE liposomes |
100–600 nm |
NA |
Covalent linkage of Ag: ↑ IgG and IgM; Encapsulation of Ag: ↑ IgG; |
NA |
NA |
NA |
244
|
Mice injected with nanosystems |
MUC-1 (inside or at the surface of liposome) |
DSPC/Chol/DMPG/MPLA liposomes |
NA |
Cytokine produced by T cells |
Strong antigen-specific T cell responses; Only surface conjugation: strong MUC-1 specific antibody; |
IL-12 secretion by macrophages |
Antigen associated with liposomes processed by APC (dendritic cells and macrophages) |
NA |
245
|
VII. Tuning nm properties to adjust the anti-viral immune response
One of the main interests of introducing nano-adjuvants in vaccines comes from the fact that their properties can be adjusted to modulate their interactions with the immune system. Assuming that the vaccine active principle VAP and NM behave similarly, which implies among other things that the VAP remains attached to the NM during the various steps of NM interaction with the immune system, this aspect could be used to optimize the properties of COVID-19 vaccines. Below, the impact of NM properties on immune reactions is presented through behaviors deduced from specific examples taken outside of the COVID-19 literature that lacks to the author knowledge an experimentally-based description of these aspects. Individual NM properties have been isolated from each other to be able to draw some specific trends. Experimental proofs would be needed to confirm that these behaviors apply to COVID 19 vaccines.
First, cellular internalization of the nano-adjuvant and associated active ingredient (mRNA, DNA, spike protein, etc.), designated as NM-VAP, could potentially be improved by reducing the NM-VAP size, as has been shown for PLGA NM whose internalization was larger at 0.3 μm than at 1–17 μm,158 or by choosing a NM-VAP with a negative charge that could promote electrostatic interactions between the cationic NM-VAP and anionic cell membranes,159 where the enhanced internalization was associated with a more efficient immune response.158
Second, the passage of NM-VAP through lysosomes, which is essential for antigen processing, could be achieved by varying the shape ratio of NM, e.g. nanosheet were retained in cytoplasm and lysosomes,160 while nano rods ended up to the nucleus.161
Third, the use of NM-VAP could enable targeting a large portion of the whole DC population by using NM of various sizes.162,163
Fourth, to enhance cytokine/chemokine/co-stimulatory molecule production as well as DC activation, hydrophobic or cationic NM-VAP could be chosen since they were shown to generate such immune reaction in a more pronounced manner than hydrophilic or anionic system nano-systems.164–166
Fifth, the immune response can potentially be tuned by attaching to the surface of NM molecules that yields a desired immune effect such as: (i) CD47 molecules to reduce NP internalization by phagocytic cells,167 TLR-7, TLR-8, and TLR-9 agonists o modulate TLR-mediated immune responses,168 TLR agonist to activate the complement pathway.169
VIII. Added values of nano-components in vaccines
In most cases, NM are introduced in vaccines as adjuvant. It therefore appears necessary to wonder about the added value provided by nano-adjuvants compared to standard adjuvants.170 Adjuvants are introduced into vaccines to improve the immune response against a pathogen,171 to yield a more sustained immune response, e.g. by increasing the duration of the antibody response,170 to potentially reduce the dose of antigens needed to stimulate the immune system,172 and to ease antigen penetration/diffusion in specific regions of immune interest such as the mucus.173 The adjuvants which are currently used to achieve these effects suffer from certain weaknesses. First, they partially stimulate the immune system, e.g. saponin was reported not to trigger T Cell response whereas Alum and MF59 were described as yielding a Th2 response leading to antibody production without leading to a strong Th1 response. Second, they can display a certain level of toxicity, e.g. saponin can activates inflammasomes leading to APC apoptosis while Complete Freud's Adjuvant (CFA) can create a local inflammation at the site of injection.174 Third, the administration at the site of infection, which is in some cases requested to prevent systemic toxicity as it is the case with ADU-S100 and MK-1454, can be complex to achieve. Fourth, antigen release mechanisms, which determine in large part vaccine efficacy, are not always optimal, e.g. adjuvants in the form of emulsions such as Montanide operate by allowing a depot of the antigen within the injection area followed by a slow diffusion of the antigen from this region without however always a full control over such behavior.175 Nano-adjuvants allow to overcome these weaknesses in large part. Indeed, they can enable to tune the strength of the immune response by adjusting NM physicochemical properties or by associating NM with several types of different immune entities, e.g. anionic liposomes were associated with TLR4 and TLR7 agonists.176 NM can also yield a reduction in vaccine toxicity. This can be due to the nature of NM, which are often less toxic than standard adjuvants. This can also come from the lower doses of VAP necessary when VAP are combined with NM than when they are associated with standard adjuvants.177 NM also enable various routes of vaccine administration, better targeting properties than standard adjuvant, and a controlled release of the VAP by adjusting the type of association/binding between the VAP and NM, which allows breaking this binding under well-defined conditions, i.e. acidic pH for example. In several cases, standard adjuvants are also not incompatible with nano-adjuvants. Indeed, standard adjuvants could be prepared at the nanometric scale, either with the same composition as for CaP NM,178 or with a slightly different one as for NM containing Zinc and Aluminum (ZA).179 In both cases, vaccines led to the triggering of the immune system, which increased survival in mice infected with pathogens,178 or produced tumor growth retardation in mice bearing lymphoma tumors.179 Considering the latter approach, it presents the advantage of allowing a comparison between the efficacy of standard adjuvants and equivalent ones fabricated at nanometer scale, thus facilitating clinical trial design by offering control groups of patients with pre-established behaviors. Fig. 6 summarizes the advantages provided by nano-adjuvants.
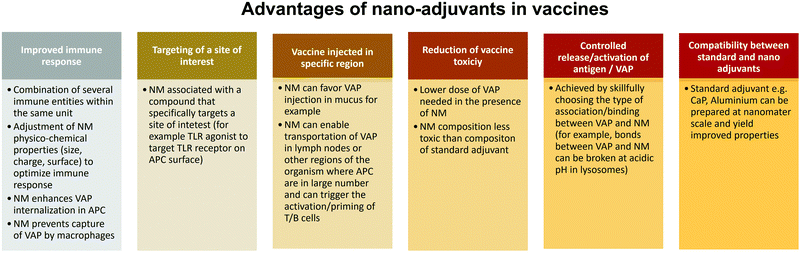 |
| Fig. 6 The different advantages provided using NM as vaccine adjuvants, including an improved immune response, the targeting of certain sites of immune interest, the facilitated injection of the vaccine in a desired region of the organism, the reduction of vaccine toxicity, the controlled release/activation of the antigen, the compatibility or complementarity between standard and nano adjuvants. | |
Conclusion
In our battle against COVID 19, NM have been shown to be useful for different applications, e.g. in vaccines, in masks or diagnostic tools such as PCR to improve their efficacy.180 NM incorporated in vaccines could most often be classified as adjuvants. Nano-adjuvants can often be used in vaccines against both cancer and viruses. When one examines which nano-adjuvant is best suited for use in a COVID-19 vaccine, one might therefore consider those tested against cancer to broaden its choice. Nano-adjuvants consist of NM with various compositions and structures such as graphene oxide, carbon nanotubes, various vesicles, such as micelles, liposomes, exosomes, membrane vesicles, polymers including polysaccharides, and metallic NM. The diversity of these NM makes it possible to choose the type of NM depending on the desired effect, e.g. vesicles to protect VAP against degradation. The different mechanisms of action of NM used as vaccine adjuvants include: (i) targeting by NM and associated VAP of a region of immune interest such as the mucus or certain specific cells such as APC, (ii) protection by NM of the antigen or mRNA/DNA against degradation in the organism, (iii) enhanced cellular internalization of VAP, (iv) improved presentation to MHC or processing by APC of antigen or mRNA/DNA, (v) synergistic effects between different immunogenic substances gathered within the same unit resulting in an improved immune response, (vi) optimization of vaccine properties through an adjustment of NM physicochemical properties such as NM sizes, surface, composition. As a whole, these behaviors result in nano-adjuvants displaying improved properties compared with standard adjuvants.
Abbreviations
ACE2 | Cellular receptor necessary for entry of COVID 19 in cells |
APC | Antigen presenting cell |
BC | Breast cancer |
CD | Cluster of differentiation |
CHO | Cholesterol |
CNT | Carbon nanotube |
COV | Coronavirus |
COVID-19 | Coronavirus disease 19 |
DAMP | Danger associated molecular patterns |
DC | Dendritic cell |
DNA | Deoxyribonucleic Acid |
GO | Graphene Oxide |
rGO | Reduced graphene oxide |
Ig | Immunoglobulin |
IL | Interleukin |
Im | Intramuscular |
It | Intratumoral |
Iv | Intravenous |
IFN | Interferon |
LP | Liposome |
LPS | Lipopolysaccharide |
NM | Nanomaterial |
NP | Nanoparticle |
MHC | Major histocompatibility complex |
MWCNT | Multi wall carbon nanotube |
OMV | Outer membrane vesicle |
OVA | Ovalbumin |
PAMP | Pathogen associated molecular patterns |
PC | Phosphatidylcholine |
PEG | Polyethylene glycol |
PEI | Polyethylenimine |
PGA | Polyglycolic acid |
PLA | Polylactic acid |
PLL | Poly-L-lysine |
PRR | Pathogen recognition receptors |
PS | Phosphatidylserine |
mRNA | Messenger ribonucleic acid |
ssRNA | Single stranded RNA |
RNase | Ribonuclease |
siRNA | Small interfering RNA |
piRNA | PIWI-interacting RNA |
SWCNT | Single wall carbon nanotube |
SM | Sphingomyelin |
Th | T helper cell |
TLR | Toll like receptor |
TME | Tumor microenvironment |
VAP | Vaccine active principle |
SUV | Small unilamellar vesicles |
LUV | Large unilamellar vesicles |
MLV | Multilamellar vesicles |
VLP | Virus like particle |
Conflicts of interest
Edouard Alphandéry has been working in the company Nanobacterie.
Acknowledgements
I would like to thank the BPI (“banque publique d’investissement, France”), the region of Paris (“Paris Région Entreprise, France”), the French Research Tax Credit program (“crédit d’impôt recherche”), the incubator Paris Biotech Santé, the ANRT (CIFRE 2014/0359, CIFRE 2016/0747, CIFRE 2013/0364, CIFRE 2015/976), the Eurostars programs (Nanoneck-2 E9309 and Nanoglioma E11778), the AIR program (“aide à l’innovation responsable”) from the region of Paris (A1401025Q), the ANR (“Agence Nationale de la Recherche”) Méfisto, as well as the Universities Paris 6 and Paris 11. I also would like to thank the Nomis Foundation and Markus Reinhard for their support.
References
- J. Y. Chung, M. N. Thone and Y. J. Kwon, COVID-19 vaccines: The status and perspectives in delivery points of view, Adv. Drug Delivery Rev., 2021, 170, 1–25 CrossRef CAS PubMed.
- G. Chauhan, M. J. Madou, S. Kalra, V. Chopra, D. Ghosh and S. O. Martinez-Chapa, Nanotechnology for COVID-19: Therapeutics and vaccine research, ACS Nano, 2020, 14, 7760–7782 CrossRef CAS PubMed.
- M. D. Shin, S. Shukla, Y. H. Chung, V. Beiss, S. K. Chan, O. A. Ortega-Rivera, D. M. Wirth, A. Chen, M. Sack, J. K. Pokorski and N. F. Steinmetz, COVID-19 vaccine development and a potential nanomaterial path forward, Nat. Nanotechnol., 2020, 15, 646–655 CrossRef CAS PubMed.
- Y. H. Chung, V. Beiss, S. N. Fiering and N. F. Steinmetz, COVID-19 vaccine frontrunners and their nanotechnology design, ACS Nano, 2020, 14(10), 12522–12537 CrossRef CAS PubMed.
- M. A. Heinrich, B. Martina and J. Prakash, Nanomedicine strategies to target coronavirus, Nano Today, 2020, 35, 100961 CrossRef CAS PubMed.
- C. Weiss, M. Carriere, L. Fusco, I. Capua, J. A. Regla-Nava, M. Pasquali, J. A. Scott, F. Vitale, M. A. Unal, C. Mattevi, D. Bedognetti, A. Merkoç, E. Tasciotti, A. Yilmazer, Y. Gogotsi, F. Stellacci and L. G. Delogu, Toward nanotechnology-enabled approaches against the COVID-19 pandemic, ACS Nano, 2020, 14, 6383–6406 CrossRef CAS PubMed.
- S. R. Bonam, N. G. Kotla, R. A. Bohara, Y. Rochev, T. J. Webster and J. Bayry, Potential immuno-nanomedicine strategies to fight COVID-19 like pulmonary infections, Nano Today, 2021, 36, 101051 CrossRef CAS PubMed.
- B. Temizoz, E. Kuroda and K. J. Ishii, Vaccine adjuvants as potential cancer immunotherapeutics, Int. Immunol., 2016, 28, 329–338 CrossRef CAS PubMed.
- H. Qian, C. Y. Tay, M. I. Setyawati, S. L. Chia, D. S. Lee and D. T. Leong, Protecting microRNAs from RNase degradation with steric DNA nanostructures, Chem. Sci., 2017, 8, 1062 RSC.
- S. Hamdy, A. Haddadi, R. W. Hung and A. Lavasanifar, Targeting dendritic cells with nano-particulate PLGA cancer vaccine formulations, Adv. Drug Delivery Rev., 2011, 63, 943–955 CrossRef CAS PubMed.
- D. Qiao, L. Liu, Y. Chen, C. Xue, Q. Gao, H.-Q. Mao, K. M. Leong and Y. Chen, Potency of a scalable nanoparticulate subunit vaccine, Nano Lett., 2018, 18, 3007–3016 CrossRef CAS PubMed.
- X. Zhuang, T. Wu, Y. Zhao, X. Hu, Y. Bao, Y. Guo, Q. Song, G. Li, S. Tan and Z. Zhang, Lipid-enveloped zinc phosphate hybrid nanoparticles for codelivery of H-2Kb and H-2Db-restricted antigenic peptides and monophosphoryl lipid A to induce antitumor immunity against melanoma, J. Controlled Release, 2016, 228, 26–37 CrossRef CAS PubMed.
- H. J. Park, H. L. Ko, D. H. Won, D. B. Hwang, Y. S. Shin, H. W. Kwak, H. J. Kim, J. W. Yun and J. H. Nam, Comprehensive analysis of the safety profile of a single-stranded RNA nano-structure adjuvant, Pharmaceutics, 2019, 11, 464 CrossRef CAS PubMed.
- A. Cruz-Reséndiz, J. Zepeda-Cervantes, A. Sampieri, C. Bastián-Eugenio, G. Acero, J. I. Sánchez-Betancourt, G. Gevorkian and L. Vaca, A self-aggregating peptide: Implications for the development of thermostable vaccine candidates, BMC Biotechnol., 2020, 20, 1 CrossRef PubMed.
- G. Mattheolabakis and C. M. Mikelis, Nanoparticle delivery and tumor vascular normalization: The chicken or the egg?, Front. Oncol., 2019, 9, 1227 CrossRef PubMed.
- T. Kisby, A. Yilmazer and K. Kostarelos, Reasons for success and lessons learnt from nanoscale vaccines against COVID-19, Nat. Nanotechnol., 2021, 16, 843–852 CrossRef CAS PubMed.
- B. Z. Igyarto, S. Jacobsen and S. Ndeupen, Future considerations for the mRNA-lipid nanoparticle vaccine platform, Curr. Opin. Virol., 2021, 48, 65–72 CrossRef CAS PubMed.
- J. Kim, Y. Eygeris, M. Gupta and G. Sahay, Self-assembled mRNA vaccines, Adv. Drug Delivery Rev., 2021, 170, 83–112 CrossRef CAS PubMed.
- E. Kon, U. Elia and D. Peer, Principles for designing an optimal mRNA lipid nanoparticle vaccine, Curr. Opin. Biotechnol, 2022, 73, 1–8 CrossRef PubMed.
- Y. Ishay, A. Kenig, T. Tsemach-Toren, R. Amer, L. Rubin, Y. Hershkovitz and F. Kharouf, Autoimmune phenomena following SARS-CoV-2 vaccination, Int. Immunopharmacol., 2021, 99, 107970 CrossRef CAS PubMed.
- W. Cao, L. He, W. Cao, X. Huang, K. Jia and J. Dai, Recent progress of graphene oxide as a potential vaccine carrier and adjuvant, Acta Biomater., 2020, 112, 14–28 CrossRef CAS PubMed.
- H. Yu, B. Zhang, C. Bulin, R. Li and R. Xing, High-efficient synthesis of graphene oxide based on improved hummers method, Sci. Rep., 2016, 6, 1–7 CrossRef CAS PubMed.
- M. Mahmudzadeh, H. Yari, B. Ramezanzadeh and M. Mahdavian, Highly potent radical scavenging-anti-oxidant activity of biologically reduced graphene oxide using Nettle extract as a green bio-genic amines-based reductants source instead of hazardous hydrazine hydrate, J. Hazard. Mater., 2019, 371, 609–624 CrossRef CAS PubMed.
- L. Wang, D. Yu, R. Dai, D. Fu, W. Li, Z. Guo, C. Cui, J. Xu, S. Shen and K. Ma, PEGylated doxorubicin cloaked nano-graphene oxide for dual-responsive photo-chemical therapy, Int. J. Pharm., 2019, 557, 66–73 CrossRef CAS PubMed.
- D. Stauffer, N. Dragneva, W. B. Floriano, R. C. Mawhinney, G. Fanchini, S. French, O. Rubel, D. Stauffer, N. Dragneva, W. B. Floriano, R. C. Mawhinney and G. Fanchini, An atomic charge model for graphene oxide for exploring its bioadhesive properties in explicit water: An atomic charge model for graphene oxide for exploring its bioadhesive, J. Chem. Phys., 2014, 141, 044705 CrossRef CAS PubMed.
- L. Xu, J. Xiang, Y. Liu, J. Xu, Y. Luo, L. Feng, Z. Liu and R. Peng, Functionalized graphene oxide serves as a novel vaccine nano-adjuvant for robust stimulation of cellular immunity, Nanoscale, 2016, 8, 3785–3795 RSC.
- J. Russier, E. Treossi, A. Scarsi, F. Perrozzi, H. Dumortier, L. Ottaviano, M. Meneghetti, V. Palermo and A. Bianco, Evidencing the mask effect of graphene oxide: A comparative study on primary human and murine phagocytic cells, Nanoscale, 2013, 5, 11234–11247 RSC.
- H. Yue, W. Wei, Z. G. Yue, B. Wang, N. N. Luo, Y. J. Gao, D. Ma, G. H. Ma and Z. G. Su, The role of the lateral dimension of graphene oxide in the regulation of cellular responses, Biomaterials, 2012, 33, 4013–4021 CrossRef CAS PubMed.
- F. Cao, X. Zhu, X. Wang, Y. Liu, G. Ma, M. Yan and H. Sun, Alum-functionalized graphene oxide nanocomplexes for effective anticancer vaccination, Acta Biomater., 2018, 83, 390–399 Search PubMed.
- J. Ma, R. Liu, X. Wang, Q. Liu, Y. N. Chen, R. P. Valle, Y. Y. Zuo, T. Xia and S. J. Liu, A crucial role of lateral size for graphene oxide in activating macrophages and stimulating pro-inflammatory responses in cells and animals, ACS Nano, 2015, 9, 10498–10515 CrossRef CAS PubMed.
- G. Chen, H. Yang, C. Lu, Y. Chao, S. Hwang, C. Chen, K. Lo, L. Sung, W. Luo, H. Tuan and Y. Hu, Simultaneous induction of autophagy and toll-like receptor signaling pathways by graphene oxide, Biomaterials, 2012, 33, 6559–6569 CrossRef CAS PubMed.
- C. Xu, H. Hong, Y. Lee, K. S. Park, M. Sun, T. Wang, M. E. Aikins, Y. Xu and J. J. Moon, Efficient lymph node-targeted delivery of personalized cancer vaccines with reactive oxygen species-inducing reduced graphene oxide nanosheets, ACS Nano, 2020, 14, 13268–13278 CrossRef CAS PubMed.
- H. A. F. M. Hassana, S. S. Diebold, L. A. Smyth, A. A. Walters, G. Lombardi and K. T. Al-Jamal, Application of carbon nanotubes in cancer vaccines: Achievements, challenges and chances, J. Controlled Release, 2019, 297, 79–90 CrossRef PubMed.
- S. Iijima, Helical microtubules of graphitic carbon, Nature, 1991, 354, 56–58 CrossRef CAS.
- E. T. Thostenson, Z. Ren and T. W. Chou, Advances in the science and technology of carbon nanotubes and their composites: A review, Compos. Sci. Technol., 2001, 61, 1899–1912 CrossRef CAS.
- D. Tasis, N. Tagmatarchis, A. Bianco and M. Prato, Chemistry of carbon nanotubes, Chem. Rev., 2006, 106, 1105–1136 CrossRef CAS PubMed.
- M. Zheng, A. Jagota, E. D. Semke, B. A. Diner, R. S. McLean, S. R. Lustig, R. E. Richardson and N. G. Tassi, DNA-assisted dispersion and separation of carbon nanotubes, Nat. Mater., 2003, 2, 338–342 CrossRef CAS PubMed.
- X. Wang, T. Xia, S. A. Ntim, Z. Ji, S. George, H. Meng, H. Zhang, V. Castranova, S. Mitra and A. E. Nel, Quantitative techniques for assessing and controlling the dispersion and biological effects of multiwalled carbon nanotubes in mammalian tissue culture cells, ACS Nano, 2010, 4, 7241–7252 CrossRef CAS PubMed.
- C. H. Villa, T. Dao, I. Ahearn, N. Fehrenbacher, E. Casey, D. A. Rey, T. Korontsvit, V. Zakhaleva, C. A. Batt, M. R. Philips and D. A. Scheinberg, Single-walled carbon nanotubes deliver peptide antigen into dendritic cells and enhance igg responses to tumor-associated antigens, ACS Nano, 2011, 5, 5300–5311 CrossRef CAS PubMed.
- K. T. Al-Jamal, A. Nunes, L. Methven, H. Ali-Boucetta, S. Li, F. M. Toma, M. A. Herrero, W. T. Al-Jamal, H. M. ten Eikelder, J. Foster, S. Mather, M. Prato, A. Bianco and K. Kostarelos, Degree of chemical functionalization of carbon nanotubes determines tissue distribution and excretion profile, Angew. Chem., Int. Ed., 2012, 51, 6389–6393 CrossRef CAS PubMed.
- X. Zhang, W. Hu, J. Li, L. Tao and Y. Wei, A comparative study of cellular uptake and cytotoxicity of multi-walled carbon nanotubes, graphene oxide, and nanodiamond, Toxicol. Res., 2012, 1, 62–68 CrossRef CAS.
- C. F. Lopez, S. O. Nielsen, P. B. Moore and M. L. Klein, Understanding nature's design for a nanosyringe, Proc. Natl. Acad. Sci. U. S. A., 2004, 101, 4431–4434 CrossRef CAS PubMed.
- K. Kostarelos, L. Lacerda, G. Pastorin, W. Wu, S. Wieckowski, J. Luangsivilay, S. Godefroy, D. Pantarotto, J. P. Briand, S. Muller, M. Prato and A. Bianco, Cellular uptake of functionalized carbon nanotubes is independent of functional group and cell type, Nat. Nanotechnol., 2007, 2, 108–113 CrossRef CAS PubMed.
- H. A. F. M. Hassan, L. Smyth, J. T. W. Wang, P. M. Costa, K. Ratnasothy, S. S. Diebold, G. Lombardi and K. T. Al-Jamal, Dual stimulation of antigen presenting cells using carbon nanotube-based vaccine delivery system for cancer immunotherapy, Biomaterials, 2016, 104, 310–322 CrossRef CAS PubMed.
- H. Takahashi, K. Misato, T. Aoshi, Y. Yamamoto, Y. Kubota, X. Wu, E. Kuroda, K. J. Ishii, H. Yamamoto and Y. Yoshioka, Carbonate apatite nanoparticles act as potent vaccine adjuvant delivery vehicles by enhancing cytokine production induced by encapsulated cytosine-phosphate-guanine oligodeoxynucleotides, Front. Immunol., 2018, 9, 783 CrossRef PubMed.
- T. Trimaille and B. Verrier, Micelle-based adjuvants for subunit vaccine delivery, Vaccines, 2015, 3, 803–813 CrossRef CAS PubMed.
- G. Jiménez-Sánchez, V. Pavot, C. Chane-Haong, N. Handké, C. Terrat, D. Gigmes, T. Trimaille and B. Verrier, Preparation and in vitro evaluation of Imiquimod loaded polylactide-based micelles as potential vaccine adjuvants, Pharm. Res., 2015, 32, 311–320 CrossRef PubMed.
- Y. W. Noh, J. H. Hong, S. M. Shim, H. S. Park, H. H. Bae, E. K. Ryu, J. H. Hwang, C. H. Lee, S. H. Cho and M. H. Sung, Polymer nanomicelles for efficient mucus delivery and antigen-specific high mucosal immunity, Angew. Chem., Int. Ed., 2013, 52, 7684–7689 CrossRef CAS PubMed.
- Z. Luo, P. Li, J. Deng, N. Gao, Y. Zhang, H. Pan, L. Liu, C. Wang, L. Cai and Y. Ma, Cationic polypeptide micelle-based antigen delivery system: A simple and robust adjuvant to improve vaccine efficacy, J. Controlled Release, 2013, 170, 259–267 CrossRef CAS PubMed.
- Z. Luo, C. Wang, H. Yi, P. Li, H. Pan, L. Liu, L. Cai and Y. Ma, Nanovaccine loaded with poly I:C and STAT3 siRNA robustly elicits anti-tumor immune responses through modulating tumor-associated dendritic cells in vivo, Biomaterials, 2015, 38, 50–60 CrossRef CAS PubMed.
- K. Hyungjin, U. Tomofumi, A. Takami, B. Masanori and A. Mitsuru, Amphiphilic poly(amino acid) nanoparticles induce size-dependent dendritic cell maturation, Adv. Funct. Mater., 2010, 20, 3925–3931 CrossRef.
- B. Layek, L. Lipp and J. Singh, APC targeted micelle for enhanced intradermal delivery of hepatitis B DNA vaccine, J. Controlled Release, 2015, 207, 143–153 CrossRef CAS PubMed.
- Q. Zeng, H. Jiang, T. Wang, Z. Zhang, T. Gong and X. Sun, Cationic micelle delivery of Trp2 peptide for efficient lymphatic draining and enhanced cytotoxic T-lymphocyte responses, J. Controlled Release, 2015, 200, 1–12 CrossRef CAS PubMed.
- M. Zhao, M. Li, Z. Zhang, T. Gong and X. Sun, Induction of HIV-1 gag specific immune responses by cationic micelles mediated delivery of gag mRNA, Drug Delivery, 2015, 23(7), 2596–2607 CrossRef PubMed.
- Z. Luo, S. Shi, L. Jin, L. Xu, J. Yu, H. Chen and X. Li, Cationic micelle based vaccine induced potent humoral immuneresponse through enhancing antigen uptake and formation of germinal center, Colloids Surf., B, 2015, 135, 556–564 CrossRef CAS PubMed.
- S. Keller, J. T. Wilson, G. I. Patilea, H. B. Kern, A. J. Convertine and P. S. Stayton, Neutral polymer micelle carriers with pH-responsive, endosome-releasing activity modulate antigen trafficking to enhance CD8+ T cell responses, J. Controlled Release, 2014, 191, 24–33 CrossRef CAS PubMed.
- S. Y. Kim, S. Kim, J. E. Kim, S. N. Lee, I. W. Shin, H. S. Shin, S. M. Jin, Y. W. Noh, Y. J. Kang, Y. S. Kim, T. H. Kang, Y. M. Park and Y. T. Lim, Lyophilizable and multifaceted toll-like receptor 7/8 agonist-loaded nanoemulsion for the reprogramming of tumor microenvironments and enhanced cancer immunotherapy, ACS Nano, 2019, 13, 12671–12686 CrossRef CAS PubMed.
- N. Wang, M. Chen and T. Wang, Liposomes used as a vaccine adjuvant-delivery system: From basics to clinical immunization, J. Controlled Release, 2019, 303, 130–150 CrossRef CAS PubMed.
- A. Chopra and G. Cevc, Non-invasive, epicutaneous immunisation with toxoid in deformable vesicles protects mice against tetanus, chiefly owing to a Th2 response, Eur, J. Pharm. Sci., 2014, 56, 55–64 CAS.
- J. J. Moon, H. Suh, A. Bershteyn, M. T. Stephan, H. Liu, B. Huang, M. Sohail, S. Luo, S. Ho Um, H. Khant, J. T. Goodwin, J. Ramos, W. Chiu and D. J. Irvine, Inter bilayer crosslinked multilamellar vesicles as synthetic vaccines for potent humoral and cellular immune responses, Nat. Mater., 2011, 10, 243–251 CrossRef CAS PubMed.
- T. Wang, Y. Y. Zhen, X. Y. Ma, B. Wei and N. Wang, Phospholipid bilayer-coated aluminum nanoparticles as an effective vaccine adjuvant-delivery system, ACS Appl. Mater. Interfaces, 2015, 7, 6391–6396 CrossRef CAS PubMed.
- R. N. Germain, Vaccines and the future of human immunology, Immunity, 2010, 33, 441–450 CrossRef CAS PubMed.
- A. E. Gregory, R. Titball and D. Williamson, Vaccine delivery using nanoparticles, Front. Cell. Infect. Microbiol., 2013, 3(13) DOI:10.3389/fcimb.2013.00013.
- T. Mazumdar, K. Anam and N. Ali, Influence of phospholipid composition on the adjuvanticity and protective efficacy of liposome-encapsulated Leishmania donovani antigens, J. Parasitol., 2005, 91, 269–274 CrossRef CAS PubMed.
- R. Kaur, V. W. Bramwell, D. J. Kirby and Y. Perrie, Pegylation of DDA:TDB liposomal adjuvants reduces the vaccine depot effect and alters the Th1/Th2 immune responses, J. Controlled Release, 2012, 158, 72–77 CrossRef CAS PubMed.
- T. Wang and N. Wang, Preparation of the multifunctional liposome-containing microneedle arrays as an Oral cavity mucosal vaccine adjuvant-delivery system, Methods Mol. Biol., 2016, 1404, 651–667 CrossRef PubMed.
- B. S. Pattni, V. V. Chupin and V. P. Torchilin, New developments in liposomal drug delivery, Chem. Rev., 2015, 115, 10938–10966 CrossRef CAS PubMed.
- N. Wang, Y. Zhen, Y. Jin, X. Wang, N. Li, S. Jiang and T. Wang, Combining different types of multifunctional liposomes loaded with ammonium bicarbonate to fabricate microneedle arrays as a vaginal mucosal vaccine adjuvant-dual delivery system (VADDS), J. Controlled Release, 2017, 246, 12–29 CrossRef CAS PubMed.
- F. S. Hassane, A. Phalipon, M. Tanguy, C. Guerreiro, F. Belot, B. Frisch, L. A. Mulard and F. Schuber, Rational design and immunogenicity of liposome-based diepitope constructs: application to synthetic oligosaccharides mimicking the Shigella flexneri 2a O-antigen, Vaccine, 2009, 27, 5419–5426 CrossRef CAS PubMed.
- A. Vangala, V. W. Bramwell, S. McNeil, D. Christensen, E. M. Agger and Y. Perrie, Comparison of vesicle based antigen delivery systems for delivery of hepatitis B surface antigen, J. Controlled Release, 2007, 119, 102–110 CrossRef CAS PubMed.
- M. T. Orr, C. B. Fox, S. L. Baldwin, S. J. Sivananthana, E. Lucas, S. Lin, T. Phan, J. J. Moon, T. S. Vedvick, S. G. Reeda and R. N. Coler, Adjuvant formulation structure and composition are critical for the development of an effective vaccine against tuberculosis, J. Controlled Release, 2013, 172, 190–200 CrossRef CAS PubMed.
- C. R. Alving, M. Rao, N. J. Steers, G. R. Matyas and A. V. Mayorov, Liposomes containing lipid A: An effective, safe, generic adjuvant system for synthetic vaccines, Expert Rev. Vaccines, 2012, 11, 733–744 CrossRef CAS PubMed.
- D. Christensen, L. B. Hansen, R. Leboux, W. Jiskoot, J. P. Christensen, P. Andersen, J. Dietrich and A. Liposome-Based, Adjuvant containing two delivery systems with the ability to induce mucosal immunoglobulin A following a parenteral immunization, ACS Nano, 2019, 13, 1116–1126 CAS.
- T. Kang, Y. Huang, Q. Zhu, H. Cheng, Y. Pei, J. Feng, M. Xu, G. Jiang, Q. Song, T. Jiang, H. Chen, X. Gao and J. Chen, Necroptotic cancer cells-mimicry nanovaccine boosts anti-tumor immunity with tailored immune-stimulatory modality, Biomaterials, 2018, 164, 80–97 CrossRef CAS PubMed.
- H. Zhao, B. Zhao, L. Wu, H. Xiao, K. Ding, C. Zheng, Q. Song, L. Sun, L. Wang and Z. Zhang, Amplified cancer immunotherapy of a surface-engineered antigenic microparticle vaccine by synergistically modulating tumor microenvironment, ACS Nano, 2019, 13, 12553–12566 CrossRef CAS PubMed.
- X. Huang, T. Yuan, M. Tschannen, Z. Sun, H. Jacob, M. Du, M. Liang, R. L. Dittmar, Y. Liu and M. Kohli,
et al., Characterization of human plasma-derived exosomal RNAs by deep sequencing, BMC Genom., 2013, 14, 319 CrossRef CAS PubMed.
- T. Kogure, I. K. Yan, W. L. Lin and T. Patel, Extracellular vesicle-mediated transfer of a novel long noncoding RNA TUC339: A mechanism of intercellular signaling in human hepatocellular cancer, Genes Cancer, 2013, 4, 261–272 CrossRef CAS PubMed.
- Y. Sato-Kuwara, S. A. Melo, F. A. Soares and G. A. Calin, The fusion of two worlds: Non-coding RNAs and extracellular vesicles—Diagnostic and therapeutic implications, Int. J. Oncol., 2014, 46, 17–27 CrossRef PubMed.
- A. K. Ludwig and B. Giebel, Exosomes: Small vesicles participating in intercellular communication, Int. J. Biochem. Cell Biol., 2012, 44, 11–15 CrossRef CAS PubMed.
- M. Simons and G. Raposo, Exosomes – vesicular carriers for intercellular communication, Curr. Opin. Cell Biol., 2009, 21, 575–581 CrossRef CAS PubMed.
- F. Chalmin, S. Ladoire, G. Mignot, J. Vincent, M. Bruchard and J.-P. Remy-Martin,
et al., Membrane-associated Hsp72 from tumor-derived exosomes mediates STAT3-dependent immunosuppressive function of mouse and human myeloid-derived suppressor cells, J. Clin. Invest., 2010, 120, 457 CAS.
- S. Montaner-Tarbes, F. E. Borras, M. Montoya, L. Fraile and H. A. Del Portillo, Serum-derived exosomes from non-viremic animals previously exposed to the porcine respiratory and reproductive virus contain antigenic viral proteins, Vet. Res., 2016, 47, 59 CrossRef PubMed.
- K. Cheng, Q. Kang and X. Zhao, Biogenic nanoparticles as immunomodulator for tumor treatment, Wiley Interdiscip. Rev.: Nanomed. Nanobiotechnol., 2020, 12, e1646 Search PubMed.
- M. J. H. Gerritzen, D. E. Martens, R. H. Wijffels, L. Van der Pold and M. Storka, Bioengineering bacterial outer membrane vesicles as vaccine platform, Biotechnol. Adv., 2017, 35, 565–574 CrossRef CAS PubMed.
- S. Stremersch, S. C. De Smedt and K. Raemdonck, Therapeutic and diagnostic applications of extracellular vesicles, J. Controlled Release, 2016, 244, 167–183 CrossRef CAS PubMed.
- K. Tan, R. Li, X. Huang and Q. Liu, Outer membrane vesicles: Current status and future direction of these novel vaccine adjuvants, Front. Microbiol., 2018, 9, 783 CrossRef PubMed.
- A. Kulp and M. J. Kuehn, Biological functions and biogenesis of secreted bacterial outer membrane vesicles, Annu. Rev. Microbiol., 2010, 64, 163–184 CrossRef CAS PubMed.
- R. S. Munford, Endotoxemia—Menace, marker, or mistake?, J. Leukoc. Biol., 2016, 100, 687–698 CrossRef CAS PubMed.
- A. Zariri, E. Pupo, E. Van Riet, J. P. Van Putten and P. Van der Ley, Modulating endotoxin activity by combinatorial bioengineering of meningococcal lipopolysaccharide, Sci. Rep., 2016, 6, 36575 CrossRef CAS PubMed.
- T. Y. Lee, C. U. Kim, E. H. Bae, S. H. Seo, D. G. Jeong, S. W. Yoon, K. T. Chang, Y. S. Kim, S. H. Kim and D. J. Kim, Outer membrane vesicles harboring modified lipid A moiety augment the efficacy of an influenza vaccine exhibiting reduced endotoxicity in a mouse model, Vaccine, 2017, 35, 586–595 CrossRef CAS PubMed.
- A. Comberlato, K. Paloja and M. M. C. Bastings, Nucleic acids presenting polymer nanomaterials as vaccine adjuvants, J. Mater. Chem. B, 2019, 7, 6321 RSC.
- S. Guo, D. Fu, A. Utupova, D. Sun, M. Zhou, Z. Jin and K. Zhao, Applications of polymer-based nanoparticles in vaccine field, Nanotechnol. Rev., 2019, 8, 143–155 CAS.
- A. Gutjahr, C. Phelip, A.-L. Coolen, C. Monge, A.-S. Boisgard, S. Paul and B. Verrier, Biodegradable polymeric nanoparticles-based vaccine adjuvants for lymph nodes targeting, Vaccines, 2016, 4, 34 CrossRef PubMed.
- J. Han, D. Zhao, D. Li, X. Wang, Z. Jin and K. Zhao, Polymer-based nanomaterials and applications for vaccines and drugs, Polymers, 2018, 10, 31 CrossRef PubMed.
- B. Sun, S. Yu, D. Zhao, S. Guo, X. Wang and K. Zhao, Polysaccharides as vaccine adjuvants, Vaccine, 2018, 36, 5226–5234 CrossRef CAS PubMed.
- X. Wei, J. Liao, Z. Davoudi, H. Zheng, J. Chen, D. Li, X. Xiong, Y. Yin, X. Yu, J. Xiong and Q. Wang, Folate receptor-targeted and GSH-responsive carboxymethyl chitosan nanoparticles containing covalently entrapped 6-mercaptopurine for enhanced intracellular drug delivery in leukemia, Mar. Drugs, 2018, 16, 439, DOI:10.3390/md16110439.
- E. A. Scott, A. Stano, M. Gillard, A. C. Maio-Liu, M. A. Swartz and J. A. Hubbell, Dendritic cell activation and T cell priming with adjuvant- and antigen-loaded oxidation-sensitive polymersomes, Biomaterials, 2012, 33, 6211–6219 CrossRef CAS PubMed.
- P. Li, Z. Luo, P. Liu, N. Gao, Y. Zhang, H. Pan, L. Liu, C. Wang, L. Cai and Y. Ma, Bioreducible alginate-poly(ethylenimine) nanogels as an antigen-delivery system robustly enhance vaccine-elicited humoral and cellular immune responses, J. Controlled Release, 2013, 168, 271–279 CrossRef CAS PubMed.
- B. M. Discher, Y. Y. Won, D. S. Ege, J. C. M. Lee, F. S. Bates, D. E. Discher and D. A. Hammer, Polymersomes: tough vesicles made from diblock copolymers, Science, 1999, 284, 1143–1146 CrossRef CAS PubMed.
- R. Sharma, U. Agrawal, N. Mody and S. P. Vyas, Polymer nanotechnology based approaches in mucosal vaccine delivery: Challenges and opportunities, Biotechnol. Adv., 2015, 33, 64–79 CrossRef CAS PubMed.
- K. Zhao, R. Guangyu, Q. Teng, X. Li, H. Lan, L. Yu, S. Yu, Z. Jin, G. Chen and Z. Li, Dendrigraft poly-L-lysines delivery of DNA vaccine effectively enhances the immunogenic responses against H9N2 avian influenza virus infection in chickens, Nanomedicine, 2020, 27, 102209 CrossRef CAS PubMed.
- J. S. Chahal, O. F. Khan, C. L. Cooper, J. S. McPartlan, J. K. Tsosie, L. D. Tilley, S. M. Sidik, S. Lourido, R. Langer, S. Bavari, H. L. Ploegh and D. G. Anderson, Dendrimer-RNA nanoparticles generate protective immunity against lethal Ebola, H1N1 influenza, and Toxoplasma gondii challenges with a single dose, Proc. Natl. Acad. Sci. U. S. A., 2016, 113, E4133–E4142 CrossRef CAS PubMed.
- A. M. Carmona-Ribeiro and Y. Pérez-Betancourt, Cationic nanostructures for vaccines design, Biomimetics, 2020, 5, 32 CrossRef CAS PubMed.
- R. J. C. Bose, M. Kim, J. H. Chang, R. Paulmurugan, J. J. Moon, W. G. Koh, S. H. Lee and H. Park, Biodegradable polymers for modern vaccine development, J. Ind. Eng. Chem., 2019, 77, 12–24 CrossRef CAS PubMed.
- M. Khansarizadeh, A. Mokhtarzadeh, M. Rashedinia, S. M. Taghdisi, P. Lari, K. H. Abnous and M. Ramezani, Identification of possible cytotoxicity mechanism of polyethylenimine by proteomics analysis, Hum. Exp. Toxicol., 2016, 35, 377–387 CrossRef CAS PubMed.
- F. G. Torres, O. P. Troncoso, A. Pisani, F. Gatto and G. Bardi, Natural polysaccharide nanomaterials: An overview of their immunological properties, Int. J. Mol. Sci., 2019, 20, 5092 CrossRef CAS PubMed.
- G. Sharma, D. T. Valenta, Y. Altman, S. Harvey, H. Xie, S. Mitragotri and J. W. Smith, Polymer particle shape independently influences binding and internalization by macrophages, J. Controlled Release, 2010, 147, 408–412 CrossRef CAS PubMed.
- J. A. Champion and S. Mitragotri, Role of target geometry in phagocytosis, Proc. Natl. Acad. Sci. U. S. A., 2006, 103, 4930–4934 CrossRef CAS PubMed.
- Z. G. Yue, W. Wei, P. P. Lv, H. Yue, L. Y. Wang, Z. G. Su and G. H. Ma, Surface charge affects cellular uptake and intracellular trafficking of chitosan-based nanoparticles, Biomacromolecules, 2011, 12, 2440–2446 CrossRef CAS PubMed.
- A. L. Siefert, M. J. Caplan and T. M. Fahmy, Artificial bacterial biomimetic nanoparticles synergize pathogen associated molecular patterns for vaccine efficacy, Biomaterials, 2016, 97, 85–96 CrossRef CAS PubMed.
- Z. Jin, S. Gao, X. Cui, D. Sun and K. Zhao, Adjuvants and delivery systems based on polymeric nanoparticles for mucosal vaccines, Int. J. Pharm., 2019, 572, 118731 CrossRef CAS PubMed.
- P. Sharma, N. Y. Jang, J. W. Lee, B. C. Park, Y. K. Kim and N. H. Cho, Application of ZnO-based nanocomposites for vaccines and cancer immunotherapy, Pharmaceutics, 2019, 11, 493 CrossRef CAS PubMed.
- S. A. C. Carabineiro, Applications of gold nanoparticles in nanomedicine: Recent advances in vaccines, Molecules, 2017, 22, 857 CrossRef PubMed.
- L. A. Dykman, Gold nanoparticles for preparation of antibodies and vaccines against infectious diseases, Expert Rev. Vaccines, 2020, 19, 465–477 CrossRef CAS PubMed.
- S. A. C. Carbineiro, Applications of gold nanoparticles in nanomedicine: Recent advances in vaccines, Molecules, 2017, 22, 857, DOI:10.3390/molecules22050857.
- M. Zhu, L. Du, R. Zhao, H. Y. Wang, Y. Zhao, G. Nie and R. F. Wang, Cell-penetrating nanoparticles activate the inflammasome to enhance antibody production by targeting microtubule-associated protein 1-light chain 3 for degradation, ACS Nano, 2020, 14, 3703–3717 CrossRef CAS PubMed.
- S. Afroz, H. Medhi, S. Maity, G. Minhas, S. Battu, J. Giddaluru, K. Kumar, P. Paik and N. Khan, Mesoporous ZnO nanocapsules for the induction of enhanced antigen-specific immunological responses, Nanoscale, 2017, 9, 14641 RSC.
- G. C. Carvalho, R. M. Sábio, T. D. C. Ribeiro, A. S. Monteiro, D. V. Pereira, S. J. L. Ribeiro and M. Chorilli, Highlights in mesoporous silica nanoparticles as a multifunctional controlled drug delivery nanoplatform for infectious diseases treatment, Pharm. Res., 2020, 37, 191, DOI:10.1007/s11095-020-02917-6.
- Q. Wang, H. Cheng, H. Peng, H. Zhou, P. Y. Li and R. Langer, Non-genetic engineering of cells for drug delivery and cell-based therapy, Adv. Drug Delivery Rev., 2015, 91, 125–140, DOI:10.1016/j.addr.2014.12.003.
- S. Gurunathan, M. H. Kang and J. H. Kim, Diverse Effects of Exosomes on COVID-19: A Perspective of Progress From Transmission to Therapeutic Developments, Front. immunol., 2021, 12, 716407 CrossRef CAS PubMed.
- M. K. Saadeldin, A. K. Abdel-Aziz and A. Abdellatif, Dendritic cell vaccine immunotherapy; the beginning of the end of cancer and COVID-19. A hypothesis, Med. Hypotheses, 2021, 146, 110365 CrossRef CAS PubMed.
- M. S. Choudhery and D. T. Harris, Stem cell therapy for COVID-19: Possibilities and challenges, Cell Biol. Int., 2020, 44, 2182–2191 CrossRef CAS PubMed.
- K. T. Gause, A. K. Wheatley, J. Cui, Y. Yan, D. J. Kent and F. Caruso, Immunological principles guiding the rational design of particles for vaccine delivery, ACS Nano, 2017, 11, 54–68 CrossRef CAS PubMed.
- A. C. Gomes, M. Mohsen and M. F. Bachmann, Harnessing nanoparticles for immunomodulation and vaccines, Vaccines, 2017, 5, 6 CrossRef PubMed.
- S. Singha, K. Shao, K. K. Ellestad, Y. Yang and P. Santamaria, Nanoparticles for immune stimulation against infection, cancer, and autoimmunity, ACS Nano, 2018, 12, 10621–10635 CrossRef CAS PubMed.
- A. Tanaka and S. Sakaguchi, Targeting Treg cells in cancer immunotherapy, Eur. J. Immunol., 2019, 49, 1140–1146 CrossRef CAS PubMed.
- C. Thomas, V. Gupta and F. Ahsan, Influence of surface charge of PLGA particles of recombinant hepatitis B surface antigen in enhancing systemic and mucosal immune responses, Int. J. Pharm., 2009, 379, 41–50 CrossRef CAS PubMed.
- H. K. Dewangan, Rational application of nanoadjuvant for mucosal vaccine delivery system, J. Immunol. Methods, 2020, 481–482, 112791 CrossRef CAS PubMed.
- N. Csaba, M. Garcia-Fuentes and M. J. Alonso, Nanoparticles for nasal vaccination, Adv. Drug Delivery Rev., 2009, 61, 140–157 CrossRef CAS PubMed.
- C. H. Huang, C. Y. Huang, H. M. Ho, C. H. Lee, P. T. Lai, S. C. Wu, S. J. Liu and M. H. Huang, Nanoemulsion adjuvantation strategy of tumor-associated antigen therapy rephrases mucosal and immunotherapeutic signatures following intranasal vaccination, J. Immunother. Cancer, 2020, 8, e001022 CrossRef PubMed.
- S. Hirosue and J. Dubrot, Modes of antigen presentation by lymph node stromal cells and their immunological implications, Front. Immunol., 2015, 6, 446 Search PubMed.
- S. N. Mueller, S. Tian and J. M. DeSimone, Rapid and persistent delivery of antigen by lymph node targeting PRINT nanoparticle vaccine carrier to promote humoral immunity, Mol. Pharmaceutics, 2015, 12, 1356–1365 CrossRef CAS PubMed.
- S. H. Wang, J. Chen, D. Smith, Z. Cao, H. Acosta, Y. Fan, S. Ciotti, A. Fattom and J. Baker, A novel combination of intramuscular vaccine adjuvants, nanoemulsion and CpG produces an effective immune response against influenza A virus, Vaccine, 2020, 38, 3537–3544 CrossRef CAS PubMed.
- A. Marabelle, H. Kohrt, C. Caux and R. Levy, Intratumoral immunization: A new paradigm for cancer therapy, Clin. Cancer Res., 2014, 20, 1747–1756 CrossRef CAS PubMed.
- Q. Zhoua, C. Dong, W. Fan, H. Jiang, J. Xiang, N. Qiu, Y. Piao, T. Xie, Y. Luo, Z. Li, F. Liu and Y. Shen, Tumor extravasation and infiltration as barriers of nanomedicine for high efficacy: The current status and transcytosis strategy, Biomaterials, 2020, 240, 119902 CrossRef PubMed.
- B. Slütter, L. Plapied, V. Fievez, M. A. Sande, A. des Rieux, Y. J. Schneider, E. Van Riet, W. Jiskoot and V. Préat, Mechanistic study of the adjuvant effect of biodegradable nanoparticles in mucosal vaccination, J. Controlled Release, 2009, 138, 113–121 CrossRef PubMed.
- V. Shalgunov, D. Zaytseva-Zotova, A. Zintchenko, T. Levada, Y. Shilov, D. Andreyev, D. Dzhumashev, E. Metelkin, A. Urusova, O. Demin, K. McDonnell, G. Troiano, S. Zale and E. Safarova, Comprehensive study of the drug delivery properties of poly(L-lactide)-poly(ethylene glycol) nanoparticles in rats and tumor-bearing mice, J. Controlled Release, 2017, 261, 31–42 CrossRef CAS PubMed.
-
Shi2021.
- S. Ahmad, A. A. Zamry, H. T. T. Tana, K. K. Wong, J. Lim and R. Mohamud, Targeting dendritic cells through gold nanoparticles: A review on the cellular uptake and subsequent immunological properties, Mol. Immunol., 2017, 91, 123–133 CrossRef CAS PubMed.
- T. Tong, Y. Qi, L. B. Bussiere, M. Wannemuehler, C. L. Miller, Q. Wang and C. Yu, Transport of artificial virus-like nanocarriers through intestinal monolayers via microfold cells, Nanoscale, 2020, 12, 16339–16347 RSC.
- M. A. Liu, A comparison of plasmid DNA and mRNA as vaccine technologies, Vaccines, 2019, 7, 37 CrossRef CAS PubMed.
- R. Madan-Lala, P. Pradhan and K. Roy, Combinatorial delivery of dual and triple TLR agonists via polymeric pathogen-like particles synergistically enhances innate and adaptive immune responses, Sci. Rep., 2017, 7, 2530 CrossRef PubMed.
- A. Vasou, N. Sultanoglu, S. Goodbourn, R. E. Randall and L. G. Kostrikis, Targeting pattern recognition receptors (PRR) for vaccine adjuvantation: From synthetic PRR agonists to the potential of defective interfering particles of viruses, Viruses, 2017, 9, 186 CrossRef PubMed.
- H. Fang, B. Ang, X. Xu, X. Huang, Y. Wu, Y. Sun, W. Wang, N. Li, X. Cao and T. Wan, TLR4 is essential for dendritic cell activation and anti-tumor T-cell response enhancement by DAMPs released from chemically stressed cancer cells, Cell. Mol. Immunol., 2014, 11, 150–159 CrossRef CAS PubMed.
- Y. Zhang, K. R. Hughes, R. M. Raghani, J. Ma, S. Orbach, J. S. Jeruss and L. D. Shea, Cargo-free immunomodulatory nanoparticles combined with anti-PD-1 antibody for treating metastatic breast cancer, Biomaterials, 2021, 269, 120666 CrossRef CAS PubMed.
- A. Schudel, D. M. Francis and S. N. Thomas, Material design for lymph node drug delivery, Nat. Rev. Mater., 2019, 4, 415–428 CrossRef PubMed.
- T. Fifis, A. Gamvrellis, B. Crimeen-Irwin, G. A. Pietersz, J. Li, P. L. Mottram, I. F. C. McKenzie and M. Plebanski, Size-dependent immunogenicity: Therapeutic and protective properties of nano-vaccines against tumors, J. Immunol., 2004, 173, 3148–3154 CrossRef CAS PubMed.
- T. Hirai, Y. Yoshioka, H. Takahashi, K. Ichihashi, T. Yoshida, S. Tochigi, K. Nagano, Y. Abe, H. Kamada, S. Tsunoda, H. Nabeshi, T. Yoshikawa and Y. Tsutsumi, Amorphous silica nanoparticles enhance cross-presentation in murine dendritic cells, Biochem. Biophys. Res. Commun., 2012, 427, 553–556 CrossRef CAS PubMed.
- A. Mant, F. Chinnery, T. Elliott and A. P. Williams, The pathway of cross-presentation is influenced by the particle size of phagocytosed antigen, Immunology, 2012, 136, 163–175 CrossRef CAS PubMed.
- K. Niikura, T. Matsunaga, T. Suzuki, S. Kobayashi, H. Yamaguchi, Y. Orba, A. Kawaguchi, H. Hasegawa, K. Kajino, T. Ninomiya, K. Ijiro and H. Sawa, Gold nanoparticles as a vaccine platform: Influence of size and shape on immunological responses in vitro and in vivo, ACS Nano, 2013, 7, 3926–3938 CrossRef CAS PubMed.
- C. Foged, C. Arigita, A. Sundblad, W. Jiskoot, G. Storm and S. Frokjaer, Interaction of Dendritic Cells with Antigen-Containing Liposomes: Effect of Bilayer Composition, Vaccine, 2004, 22, 1903–1913 CrossRef CAS PubMed.
- C. Foged, B. Brodin, S. Frokjaer and A. Sundblad, Particle size and surface charge affect particle uptake by human dendritic cells in an in vitro model, Int. J. Pharm., 2005, 298, 315–322 CrossRef CAS PubMed.
- S. Y. Seong and P. Matzinger, Hydrophobicity: An ancient damage-associated molecular pattern that initiates innate immune responses, Nat. Rev. Immunol., 2004, 4, 469–478 CrossRef CAS PubMed.
- P. Matzinger, The danger model: A renewed sense of self, Science, 2002, 296, 301–305 CrossRef CAS PubMed.
-
(a) D. F. Moyano, M. Goldsmith, D. J. Solfiell, D. Landesman-Milo, O. R. Miranda, D. Peer and V. M. Rotello, Nanoparticle hydrophobicity dictates immune response, J. Am. Chem. Soc., 2012, 134, 3965–3967 CrossRef CAS PubMed;
(b) B. Pulendran, P. S. Arunachalam and D. T. O. Hagan, Emerging concepts in the science of vaccine adjuvants, Nat. Rev. Drug Discovery, 2021, 20, 454–475 CrossRef CAS PubMed.
- Y. Liu, Y. Yin, L. Y. Wang, W. F. Zhang, X. M. Chen, X. X. Yang, J. J. Xu and G. H. Ma, Surface hydrophobicity of microparticles modulates adjuvanticity, J. Mater. Chem. B, 2013, 1, 3888–3896 RSC.
- L. K. Petersen, A. E. Ramer-Tait, S. R. Broderick, C. S. Kong, B. D. Ulery, K. Rajan, M. J. Wannemuehler and B. Narasimhan, Activation of innate immune responses in a pathogen-mimicking manner by amphiphilic polyanhydride nanoparticle adjuvants, Biomaterials, 2011, 32, 6815–6822 CrossRef CAS PubMed.
- V. B. Joshi, S. M. Geary and A. K. Salem, Biodegradable particles as vaccine delivery systems: size matters, AAPS J., 2013, 15, 85–94 CrossRef CAS PubMed.
- C. Foged, B. Brodin, S. Frokjaer and A. Sundblad, Particle size and surface charge affect particle uptake by human dendritic cells in an in vitro model, Int. J. Pharm., 2005, 298, 315–322 CrossRef CAS PubMed.
- M. Pei, J. Liang, C. Zhang, X. Wang, C. Zhang, G. Ma and H. Sun, Chitosan/calcium phosphates nanosheet as a vaccine carrier for effective cross-presentation of exogenous antigens, Carbohydr. Polym., 2019, 224, 115172 CrossRef CAS PubMed.
- J. Yue, L. Liang, Y. Shen, X. Guan, J. Zhang, Z. Li, R. Deng, S. Xu, C. Liang, W. Shi and W. Xu, Investigating dynamic molecular events in melanoma cell nucleus during photodynamic therapy by SERS, Front. Chem., 2019, 6, 665 CrossRef PubMed.
- Y. Nishioka and H. Yoshino, Lymphatic targeting with nanoparticulate system, Adv. Drug Delivery Rev., 2001, 47, 55–64, DOI:10.1016/S0169-409X(00)00121-6148.
- V. Manolova, A. Flace, M. Bauer, K. Schwarz, P. Saudan and M. F. Bachmann, Nanoparticles target distinct dendritic cell populations according to their size, Eur. J. Immunol., 2008, 38, 1404–1413, DOI:10.1002/eji.200737984.
- R. S. Raghuvanshi, Y. K. Katare, K. Lalwani, M. M. Ali, O. Singh and A. K. Panda, Improved immune response from biodegradable polymer particles entrapping tetanus toxoid by use of different immunization protocol and adjuvants, Int. J. Pharm., 2002, 245, 109–121, DOI:10.1016/S0378-5173(02)00342-3.
- F. Shima, T. Akagi and M. Akashi, Effect of hydrophobic side chains in the induction of immune responses by nanoparticle adjuvants consisting of amphiphilic poly(γ-glutamic acid), Bionconjugate Chem., 2015, 26, 890–898 CrossRef CAS PubMed.
- H. W. Despres, A. Sabra, P. Anderson, U. D. Hemraz, Y. Boluk, R. Sunasee and K. Ckless, Mechanisms of the immune response cause by cationic and anionic surface functionalized cellulose nanocrystals using cell-based assays, Toxicol. In Vitro, 2019, 55, 124–133 CrossRef CAS PubMed.
- M. P. Chao, C. H. Takimoto, D. D. Feng, K. McKenna, P. Gip, J. Liu, J. P. Volkmer, I. L. Weissman and R. Majeti, Therapeutic targeting of the macrophage immune checkpoint CD47 in myeloid malignancies, Front. Oncol., 2020, 9, 1380 CrossRef PubMed.
- S. Agrawal and E. R. Kandimalla, Synthetic agonists of Toll-like receptors 7, 8 and 9, Biochem. Soc. Trans., 2007, 35, 1461–1467 CrossRef CAS PubMed.
- G. Hajishengallis and J. D. Lambris, Crosstalk pathways between Toll-like receptors and the complement system, Trends Immunol., 2010, 31, 154–163 CrossRef CAS PubMed.
- B. Pulendran, P. S. Arunachalam and D. T. O. Hagan, Emerging concepts in the science of vaccine adjuvants, Nat. Rev. Drug Discovery, 2021, 20(6), 454–475 CrossRef CAS PubMed.
- Z. B. Wang and J. Xu, Better adjuvants for better vaccines: Progress in adjuvant delivery systems, modifications, and adjuvant–antigen codelivery, Vaccines, 2020, 8, 128 CrossRef CAS PubMed.
- R. Billeskov, Y. Wang, S. Solaymani-Mohammadi, B. Frey, S. Kulkarni, P. Andersen, E. M. Agger, Y. Sui and J. A. Berzofsky, Low antigen dose in adjuvant-based vaccination selectively induces CD4 T cells with enhanced functional avidity and protective efficacy, J. Immunol., 2017, 198, 3494–3506 CrossRef CAS PubMed.
- Y. W. Noh, J. Hyun Hong, S. M. Shim, H. S. Park, H. H. Bae, E. Kyoung, R. Jung, H. Hwang, C. H. Lee, S. H. Cho, M. H. Sung, H. Poo and Y. T. Lim, Polymer nanomicelles for efficient mucus delivery and antigen-specific high mucosal immunity, Angew. Chem., Int. Ed., 2013, 52, 7684–7689 CrossRef CAS PubMed.
- N. Petrovsky, Comparative safety of vaccine adjuvants: A summary of current evidence and future needs, Drug Saf., 2015, 38, 1059–1074 CrossRef CAS PubMed.
- K. D. Brewer, G. M. Weir, I. Dude, C. Davis, C. Parsons, A. Penwell, R. Rajagopalan, L. Sammatur, C. V. Bowen and M. M. Stanford, Unique depot formed by an oil based vaccine facilitates active antigen uptake and provides effective tumour control, J. Biomed. Sci., 2018, 25, 7 CrossRef PubMed.
- C. B. Fox, S. J. Sivananthan, M. S. Duthie, J. Vergara, J. A. Guderian, E. Moon, D. Coblentz, S. G. Reed and D. Carter, A nanoliposome delivery system to synergistically trigger TLR4 AND TLR7, J. Nanobiotechnol., 2014, 12, 17 CrossRef PubMed.
- S. Renu, N. Feliciano-Ruiz, F. Lu, S. Ghimire, Y. Han, J. Schrock, S. Dhakal, V. Patil, S. Krakowka, H. HogenEsch and G. J. Renukaradhya, A nanoparticle-poly(I:C) combination adjuvant enhances the breadth of the immune response to inactivated influenza virus vaccine in pigs, Vaccines, 2020, 8, 229 CrossRef CAS PubMed.
- E. Ahmadpour, S. Sarvi, M. B. H. Soteh, M. Sharif, M. T. Rahimid, R. Valadan, M. Tehranic, A. Khalilian, M. Montazeri, M. Fasihi-Ramandi and A. Daryani, Enhancing immune responses to a DNA vaccine encoding Toxoplasmagondii GRA14 by calcium phosphate nanoparticles as an adjuvant, Immunol. Lett., 2017, 185, 40–47 CrossRef CAS PubMed.
- X. Zhong, Y. Zhang, L. Tan, T. Zheng, Y. Hou, X. Hong, G. Du, X. Chen, Y. Zhang and X. Sun, An aluminum adjuvant-integrated nano-MOF as antigen delivery system to induce strong humoral and cellular immune responses, J. Controlled Release, 2019, 300, 81–92 CrossRef CAS PubMed.
- E. Alphandéry, The potential of various nanotechnologies for coronavirus diagnosis/treatment highlighted through a literature analysis, Bioconjugate Chem., 2020, 31, 1873–1882 CrossRef PubMed.
- M. Bai, H. Dong, X. Su, Y. Jin, S. Sun, Y. Zhang, Y. Yang and H. Guo, Hollow mesoporous silica nanoparticles as delivery vehicle of foot-and-mouth disease virus-like particles induce persistent immune responses in guinea pigs, J. Med. Virol., 2019, 91, 941–948 CrossRef CAS PubMed.
- A. Bansal, W. Gamal, I. J. Menon, V. Olson, X. Wu and M. J. D’Souzaa, Laser-assisted skin delivery of immunocontraceptive rabies nanoparticulate vaccine in poloxamer gel, Eur. J. Pharm. Sci., 2020, 155, 105560 CrossRef CAS PubMed.
- A. Brotons-Canto, C. Gamazo, N. Martín-Arbella, M. Abdulkarim, M. Gumbleton, G. Quincoces, I. Peñuelas and J. M. Irache, J. Pharm. Sci., 2019, 108, 2421–2429 CrossRef CAS PubMed.
- S. Chattopadhyay, Y. H. Liu, Z. S. Fang, C. L. Lin, J. C. Lin, B. Y. Yao and C. M. J. Hu, Synthetic immunogenic cell death mediated by intracellular delivery of STING agonist nanoshells enhances anticancer chemoimmunotherapy, Nano Lett., 2020, 20, 2246–2256 CrossRef CAS PubMed.
- Q. Chen, L. Xu, C. Liang, C. Wang, R. Peng and Z. Liu, Photothermal therapy with immune-adjuvant nanoparticles together with checkpoint blockade for effective cancer immunotherapy, Nat. Commun., 2016, 7, 13193 CrossRef CAS PubMed.
- W. Chen, H. Zuo, T. J. Mahony, B. Zhang, B. Rolfe and Z. P. Xu, Efficient induction of comprehensive immune responses to control pathogenic E. coli by clay nano-adjuvant with the moderate size and surface charge, Sci. Rep., 2017, 7, 13367 CrossRef PubMed.
- Y. Cheng, Q. Chen, Z. Guo, M. Li, X. Yang, G. Wan, H. Chen, Q. Zhang and Y. Wang, An intelligent biomimetic nanoplatform for holistic treatment of metastatic triple-negative breast cancer via photothermal ablation and immune remodeling, ACS Nano, 2020, 14, 15161–15181 CrossRef PubMed.
- T. G. Dacoba, R. W. Omange, H. Li, J. Crecente-Campo, M. Luo and M. J. Alonso, Polysaccharide nanoparticles can efficiently modulate the immune response against an HIV peptide antigen, ACS Nano, 2019, 13, 4947–4959 CrossRef CAS PubMed.
- S. Dhakal, F. Lu, S. Ghimire, S. Renu, Y. S. Lakshmanappa, B. T. Hogshead, D. Ragland, H. HogenEsch and G. J. Renukaradhya, Corn-derived alpha-D-glucan nanoparticles as adjuvant for intramuscular and intranasal immunization in pigs, Nanomedicine, 2019, 16, 226–235 CrossRef CAS PubMed.
- Y. Fan, S. M. Stronsky, Y. Xu, J. T. Steffens, S. A. Van Tongeren, A. Erwin, C. L. Cooper and J. J. Moon, Multilamellar vaccine particle elicits potent immune activation with protein antigens and protects mice against ebola virus infection, ACS Nano, 2019, 13, 11087–11096 CrossRef CAS PubMed.
- F. Fontana, M. Fusciello, C. Groeneveldt, C. Capasso, J. Chiaro, S. Feola, Z. Liu, E. E. Mäkilä, J. J. Salonen, J. T. Hirvonen, V. Cerullo and H. A. Santos, Biohybrid Vaccines for Improved Treatment of Aggressive Melanoma with Checkpoint Inhibitor, ACS Nano, 2019, 13, 6477–6490 CrossRef CAS PubMed.
- P. Hart, A. Copland, G. R. Diogo, S. Harris, R. Spallek, W. Oehlmann, M. Singh, J. Basile, M. Rottenberg, M. J. Paul and R. Reljic, Nanoparticle-fusion protein complexes protect against Mycobacterium tuberculosis infection, Mol. Ther., 2018, 26, 822–833 CrossRef CAS PubMed.
- H. Jiang, Q. Wang, L. Li, Q. Zeng, H. Li, T. Gong, Z. Zhang and X. Sun, Turning the old adjuvant from gel to nanoparticles to amplify CD8+ T cell responses, Adv. Sci., 2018, 5, 1700426 CrossRef PubMed.
- X. H. Jin, L. L. Zheng, M. R. Song, W. S. Xu, Y. N. Kou, Y. Zhou, L. W. Zhang, Y. N. Zhu, B. Wan, Z. Y. Wei and G. P. Zhang, Nano silicon adjuvant enhances inactivated transmissible gastroenteritis vaccine through activation the Toll-like receptors and promotes humoral and cellular immune responses, Nanomedicine, 2018, 14, 1201–1212 CrossRef CAS PubMed.
- T. Kang, Y. Huang, Q. Zhu, H. Cheng, Y. Pei, J. Feng, M. Xu, G. Jiang, Q. Song, T. Jiang, H. Chen, X. Gao and J. Chen, Necroptotic cancer cells-mimicry nanovaccine boosts anti-tumor immunity with tailored immune-stimulatory modality, Biomaterials, 2018, 164, 80e97 CrossRef PubMed.
- Y. F. Kang, C. Sun, Z. Zhuang, R. Y. Yuan, Q. Zheng, J. P. Li, P. P. Zhou, X. C. Chen, Z. Liu, X. Zhang, X.-H. Yu, X. W. Kong, Q. Y. Zhu, Q. Zhong, M. Xu, N. S. Zhong, Y. X. Zeng, G. K. Feng, C. Ke, J. C. Zhao and M. S. Zeng, Rapid development of SARS-CoV-2 spike protein receptor-binding domain self-assembled nanoparticle vaccine candidates, ACS Nano, 2021, 15, 2738–2752 CrossRef CAS PubMed.
- S. Y. Kim, S. Kim, J. E. Kim, S. N. Lee, I. W. Shin, H. S. Shin, S. M. Jin, Y. W. Noh, Y. J. Kang, Y. S. Kim, T. H. Kang, Y. M. Park and Y. T. Lim, Lyophilizable and multifaceted toll-like receptor 7/8 agonist-loaded nanoemulsion for the reprogramming of tumor microenvironments and enhanced cancer immunotherapy, ACS Nano, 2019, 13, 12671–12686 CrossRef CAS PubMed.
- F. C. Knight, P. Gilchuk, A. Kumar, K. W. Becker, S. Sevimli, M. E. Jacobson, N. Suryadevara, L. Wang-Bishop, K. L. Boyd, J. E. Crowe, S. Joyce and J. T. Wilson, Mucosal immunization with a pH-responsive nanoparticle vaccine induces protective CD8+ lung-resident memory T cells, ACS Nano, 2019, 13, 10939–10960 CrossRef CAS PubMed.
- Q. V. Le, J. Suh, J. J. Choi, G. T. Park, J. W. Lee, G. Shim and Y. K. Oh, In situ nanoadjuvant-assembled tumor vaccine for preventing long-term recurrence, ACS Nano, 2019, 13, 7442–7462 CrossRef CAS PubMed.
- M. E. Lebel, K. Chartrand, E. Tarrab, P. Savard, D. Leclerc and A. Lamarre, Potentiating cancer immunotherapy using papaya mosaic virus-derived nanoparticles, Nano Lett., 2016, 16, 1826–1832 CrossRef CAS PubMed.
- J. W. Lim, W. Na, H. O. Kim, M. Yeom, A. Kang, G. Park, C. Park, J. Ki, S. Lee, B. Jung, H. H. Jeong, D. Park, D. Song and S. Haam, Co-delivery of antigens and immunostimulants via a polymersome for improvement of antigen-specific immune response, J. Mater. Chem. B, 2020, 8, 5620 RSC.
- F. Lu, Y. Y. C. Mosley, R. J. R. Rosales, B. E. Carmichael, S. Elesela, Y. Yao and H. HogenEsch, Alpha-D-glucan nanoparticulate adjuvant induces a transient inflammatory response at the injection site and targets antigen to migratory dendritic cells, npj Vaccines, 2017, 2, 4 CrossRef PubMed.
- L. Luo, C. Zhu, H. Yin, M. Jiang, J. Zhang, B. Qin, Z. Luo, X. Yuan, J. Yang, W. Li, Y. Du and J. You, Laser immunotherapy in combination with perdurable PD-1 blocking for the treatment of metastatic tumors, ACS Nano, 2018, 12, 7647–7662 CrossRef CAS PubMed.
- C. Meng, X. Zhi, C. Li, C. Li, Z. Chen, X. Qiu, C. Ding, L. Ma, H. Lu, D. Chen, G. Liu and D. Cui, Graphene oxides decorated with carnosine as an adjuvant to modulate innate immune and improve adaptive immunity in vivo, ACS Nano, 2016, 10, 2203–2213 CrossRef CAS PubMed.
- H. Nabi, I. Rashid, N. Ahmad, A. Durrani, H. Akbar, S. Islam, A. A. Bajwa, W. Shehzad, K. Ashraf and N. Imran, Induction of specific humoral immune response in mice immunized with ROP18 nanospheres from Toxoplasma gondii, Parasitology, 2017, 116, 359–370 CrossRef PubMed.
- R. J. Nevagi, Z. G. Khalil, W. M. Hussein, J. Powell, M. R. Batzloff, R. J. Capon, M. F. Good, M. Skwarczynski and I. Toth, Polyglutamic acid-trimethyl chitosan-based intranasal peptide nanovaccine induces potent immune responses against group A streptococcus, Acta Biomater., 2018, 80, 278–287 CrossRef CAS PubMed.
- H. I. Ng, G. J. P. Fernando, A. C. I. Depelsenaire and M. A. F. Kendall, Potent response of QS-21 as a vaccine adjuvant in the skin when delivered with the Nanopatch, resulted in adjuvant dose sparing, Sci. Rep., 2016, 6, 29368 CrossRef PubMed.
- D. Jenkins, S. Corrie, C. Flaima and M. Kendallac, High density and high aspect ratio solid micro-nanoprojection arrays for targeted skin vaccine delivery and specific antibody extraction, RSC Adv., 2012, 2, 3490–3495 RSC.
- M. A. Oberli, A. M. Reichmuth, J. R. Dorkin, M. J. Mitchell, O. S. Fenton, A. Jaklenec, D. G. Anderson, R. Langer and D. Blankschtein, Lipid nanoparticle assisted mRNA delivery for potent cancer immunotherapy, Nano Lett., 2017, 17, 1326–1335 CrossRef CAS PubMed.
- A. S. Pagheh, A. Daryani, P. Alizadeh, H. Hassannia, S. M. R. Oliveira, T. Kazemi, F. Rezaei, M. D. L. Pereira and E. Ahmadpour, Protective effect of a DNA vaccine cocktail encoding ROP13 and GRA14 with Alum nano-adjuvant against Toxoplasma gondii infection in mice, Int. J. Biochem. Cell Biol., 2021, 132, 105920 CrossRef CAS PubMed.
- Y. Pérez-Betancourt, B. C. L. F. Távora, M. Colombini, E. L. Faquim-Mauro and A. M. Carmona-Ribeiro, Simple nanoparticles from the assembly of cationic polymer and antigen as immunoadjuvants, Vaccines, 2020, 8, 105 CrossRef PubMed.
- Y. Qu, Y. Ju, C. Cortez-Jugo, Z. Lin, S. Li, J. Zhou, Y. Ma, A. Glab, S. J. Kent, F. Cavalieri and F. Caruso, Template-mediated assembly of DNA into microcapsules for immunological modulation, Small, 2020, 16, 2002750 CrossRef CAS PubMed.
- M. T. Rahimi, S. Sarvi, M. Sharif, S. Abediankenari, E. Ahmadpour, R. Valadan, R. Fasihi- Ramandie, S. A. Hosseini and A. Daryani, Immunological evaluation of a DNA cocktail vaccine with co-delivery of calcium phosphate nanoparticles (CaPNs) against the Toxoplasma gondii RH strain in BALB/c mice, Parasitol. Res., 2017, 116, 609–616 CrossRef PubMed.
- C. B. Roces, M. T. Hussain, S. T. Schmidt, D. Christensen and Y. Perrie, Investigating prime-pull vaccination through a combination of parenteral vaccination and intranasal boosting, Vaccines, 2020, 8, 10 CrossRef CAS PubMed.
- L. Rodrigues, K. N. Raftopoulos, S. T. Schmidt, F. Schneidere, H. Dietze, T. Radesc, H. Franzyk, A. E. Pederseng, C. M. Papadakis, D. Christensen, G. Winter, C. Foged and M. Hubert, Immune responses induced by nano-self-assembled lipid adjuvants based on a monomycoloyl glycerol analogue after vaccination with the Chlamydia trachomatis major outer membrane protein, J. Controlled Release, 2018, 285, 12–22 CrossRef CAS PubMed.
- M. Saydam, W. P. Cheng, N. Palmer, R. Tierney, R. Francis, K. MacLellan-Gibson, A. Khan and F. Mawas, Nano-sized Soluplus polymeric micelles enhance the induction of tetanus toxin neutralising antibody response following transcutaneous immunisation with tetanus toxoid, Vaccine, 2017, 35, 2489–2495 CrossRef CAS PubMed.
- S. T. T. Schetters, W. S. P. Jong, S. K. Horrevorts, L. J. W. Kruijssen, S. Engels, D. Stolk, M. H. Daleke-Schermerhorn, J. Garcia-Vallejo, D. Houben, W. W. J. Unger, J. M. M. den Haan, J. Luirink and Y. Van Kooyk, Outer membrane vesicles engineered to express membrane-bound antigen program dendritic cells for cross-presentation to CD8+ T cells, Acta Biomater., 2019, 91, 248–257 CrossRef CAS PubMed.
- W. Shi, Y. Kou, J. Xiao, L. Zhang, F. Gao, W. Kong, W. Su, C. Jiang and Y. Zhang, Comparison of immunogenicity, efficacy and transcriptome changes of inactivated rabies virus vaccine with different adjuvants, Vaccine, 2018, 36, 5020–5029 CrossRef CAS PubMed.
- Y. Sun, Y. Yin, L. Gong, Z. Liang, C. Zhu, C. Ren, N. Zheng, Q. Zhang, H. Liu, W. Liu, F. You, D. Lu and Z. Lin, Manganese nanodepot augments host immune response against coronavirus, Nano Res., 2020, 1–13 Search PubMed.
- Z. Teng, S. Sun, H. Chen, J. Huang, P. Du, H. Dong, X. Xu, S. Mua, Z. Zhang and H. Guo, Golden-star nanoparticles as adjuvant effectively promotes immune response to foot-and-mouth disease virus-like particles vaccine, Vaccine, 2018, 36, 6752–6760 CrossRef CAS PubMed.
- J. Tomporowski, J. M. Heer, B. Allan, S. Gomis and P. Aich, Carbon nanotubes significantly enhance the biological activity of CpG ODN in chickens, Int. J. Pharm., 2019, 561, 135–147 CrossRef CAS PubMed.
- H. Wang, Y. Ding, S. Su, D. Meng, A. Mujeeb, Y. Wu and G. Nie, Assembly of hepatitis E vaccine by ‘in situ’ growth of gold clusters as nano-adjuvants: An efficient way to enhance the immune responses of vaccination, Nanoscale Horiz., 2016, 1, 394 RSC.
- K. Wang, S. Wen, L. He, A. Li, Y. Li, H. Dong, W. Li, T. Ren, D. Shi and Y. Li, “Minimalist” nanovaccine constituted from near whole antigen for cancer immunotherapy, ACS Nano, 2018, 12, 6398–6409 CrossRef CAS PubMed.
- J. Xu, H. Wang, L. Xu, Y. Chao, C. Wang, X. Han, Z. Dong, H. Chang, R. Peng, Y. Cheng and Z. Liu, Nanovaccine based on a protein-delivering dendrimer for effective antigen cross-presentation and cancer immunotherapy, Biomaterials, 2019, 207, 1–9 CrossRef CAS PubMed.
- S. Yan, K. Xu, L. Li, W. Gu, B. E. Rolfe and Z. P. Xu, The pathways for layered double hydroxide nanoparticles to enhance antigen (cross)-presentation on immune cells as adjuvants for protein vaccines, Front. Pharmacol., 2018, 9, 1060 CrossRef PubMed.
- R. Yang, J. Xu, L. Xu, X. Sun, Q. Chen, Y. Zhao, R. Peng and Z. Liu, Cancer cell membrane-coated adjuvant nanoparticles with mannose modification for effective anticancer vaccination, ACS Nano, 2018, 12, 5121–5129 CrossRef CAS PubMed.
- W. Yang, G. Zhu, S. Wang, G. Yu, Z. Yang, L. Lin, Z. Zhou, Y. Liu, Y. Dai, F. Zhang, Z. Shen, Y. Liu, Z. He, J. Lau, G. Niu, D. O. Kiesewetter, S. Hu and X. Chen, In situ dendritic cell vaccine for effective cancer immunotherapy, ACS Nano, 2019, 13, 3083–3094 CrossRef CAS PubMed.
- P. Zamani, J. G. Navashen, A. R. Nikpoor, M. Hatamipour, R. K. Oskueee, A. Badiee and M. R. Jaafari, MPL nano-liposomal vaccine containing P5 HER2/neu-derived peptide pulsed PADRE as an effective vaccine in a mice TUBO model of breast cancer, J. Controlled Release, 2019, 303, 223–236 CrossRef CAS PubMed.
- Q. Zeng, P. Zhang, X. Zeng, L. H. Tostanoski and C. M. Jewell, Advanced manufacturing of microdisk vaccines for uniform control of material properties and immune cell function, Biomater. Sci., 2018, 6, 115 RSC.
- J. Zhang, J. Mai, F. Li, J. Shen, G. Zhang, J. Li, L. E. Hinkle, D. Lin, X. Liu, Z. Li, R. F. Wang, E. A. Mittendorf, M. Ferrari and H. Shen, Investigation of parameters that determine Nano-DC vaccine transport, Biomed. Microdevices, 2019, 21, 39 CrossRef PubMed.
- Y. N. Zhang, W. Poon, E. Sefton and W. C. W. Chan, Suppressing subcapsular sinus macrophages enhances transport of nanovaccines to lymph node follicles for robust humoral immunity, ACS Nano, 2020, 14, 9478–9490 CrossRef CAS PubMed.
- Y. N. Zhang, W. Poon, E. Sefton and W. C. W. Chan, Suppressing subcapsular sinus macrophages enhances transport of nanovaccines to lymph node follicles for robust humoral immunity, ACS Nano, 2020, 14, 9478–9490 CrossRef CAS PubMed.
- L. Zhao, D. Mahony, A. S. Cavallaro, B. Zhang, J. Zhang and J. R. Deringer,
et al., Immunogenicity of outer membrane proteins VirB9-1 and VirB9-2, a novel nanovaccine against anaplasma marginale, PLoS One, 2016, 11, e0154295 CrossRef PubMed.
- A. Beduneau, Z. Ma, C. B. Grotepas, A. Kabanov, B. E. Rabinow, N. Gong, R. L. Mosley, H. Dou, M. D. Boska and H. E. Gendelman, Facilitated monocyte-macrophage uptake and tissue distribution of superparmagnetic iron-oxide nanoparticles, PLoS One, 2009, 4, e4343 CrossRef PubMed.
- T. P. Thomas, S. N. Goonewarden, I. J. Majoros, A. Kotlyar, Z. Cao, P. R. Leroueil and J. R. Baker, Folate-targeted nanoparticles show efficacy in the treatment of inflammatory arthritis, Arthritis Rheumatism, 2011, 63, 2671–2680 CrossRef CAS PubMed.
- L. Ma, T. W. Liu, M. A. Wallig, I. T. Dobrucki, L. W. Dobrucki, E. R. Nelson, K. S. Swanson and A. M. Smith, Efficient targeting of adipose tissue macrophages in obesity with polysaccharide nano-carriers, ACS Nano, 2016, 10, 6952–6962, DOI:10.1021/acsnano.6b02878.
- L. J. Cruz, R. A. Rosalia, J. W. Kleinovink, F. Rueda, C. W. G. M. Löwik and F. Ossendorp, Targeting nanoparticles to CD40, DEC-205 or CD11c molecules on dendritic cells for efficient CD8+ T cell response: A comparative study, J. Controlled Release, 2014, 192, 209–218 CrossRef CAS PubMed.
- M. Silva, E. Zupancic, G. Vandermeulen, V. G. Oliveira, A. Salgado, M. Videira, M. Gaspar, L. Graca, V. Préat and H. F. Florindo, In vivo delivery of peptides and Toll-like receptor ligands by mannose-functionalized polymeric nanoparticles induces prophylactic and therapeutic anti-tumor immune responses in a melanoma, J. Controlled Release, 2015, 198, 91–103, DOI:10.1016/j.jconrel.2014.11.033.model.
- S. Keller, J. T. Wilson, G. I. Patilea, H. B. Kern, A. J. Convertine and P. S. Stayton, Neutral polymer micelle carriers with pH-responsive, endosome-releasing activity modulate antigen trafficking to enhance CD8+ T cell responses, J. Control. Release, 2014, 191, 24–33, DOI:10.1016/j.jconrel.2014.03.041.
- E. Yuba, A. Harada, Y. Sakanishi, S. Watarai and K. Kono, A liposome-based antigen delivery system using pH-sensitive fusogenic polymers for cancer immunotherapy, Biomaterials, 2013, 34, 3042–3052 CrossRef CAS PubMed.
- K. Zaks, M. Jordan, A. Guth, K. Sellins, R. Kedl, A. Izzo, C. Bosio and S. Dow, Efficient immunization and cross-priming by vaccine adjuvants containing TLR3 or TLR9 agonists complexed to cationic liposomes, J. Immunol., 2006, 176, 7335–7345 CrossRef CAS PubMed.
- G. Lynn, R. Laga, P. A. Darrah, A. S. Ishizuka, A. J. Balaci, A. E. Dulcey, M. Pechar, R. Pola, M. Y. Gerner, A. Yamamoto, C. R. Buechler, K. M. Quinn, M. G. Smelkinson, O. Vanek, R. Cawood, T. Hills, O. Vasalatiy, K. Kastenmüller, J. R. Francica, L. Stutts, J. K. Tom, K. A. Ryu, A. P. Esser-Kahn, T. Etrych, K. D. Fisher, L. W. Seymour and R. A. Seder, In vivo characterization of the physicochemical properties of polymer-linked TLR agonists that enhance vaccine immunogenicity, Nat. Biotechnol., 2015, 33, 1201–1210, DOI:10.1038/nbt.3371.
- V. V. Temchura, D. Kozlova, V. Sokolova, K. Überla and M. Epple, Targeting and activation of antigen-specific B-cells by calcium phosphate nanoparticles loaded with protein antigen, Biomaterials, 2014, 35(23), 6098–6105, DOI:10.1016/j.biomaterials.2014.04.010.
- E. Shahum and H. M. Thérien, Immunopotentiation of the humoral response by liposomes: Encapsulation versus covalent linkage, Immunology, 1988, 2, 315–317 Search PubMed.
- H. H. Guan, W. Budzynski, R. R. Koganty, M. J. Krantz, M. A. Reddish, J. A. Rogers, B. M. Longenecker and J. Samuel, Liposomal formulations of synthetic MUC1 peptides: Effects of encapsulation versus surface display of peptides on immune responses, Bioconjugate Chem., 1998, 9, 451–458 CrossRef CAS PubMed.
|
This journal is © The Royal Society of Chemistry 2022 |
Click here to see how this site uses Cookies. View our privacy policy here.