DOI:
10.1039/D2QM00307D
(Research Article)
Mater. Chem. Front., 2022,
6, 2826-2834
MOF-derived bimetallic core–shell catalyst HZSM-5@ZrO2–In2O3: high CO2 conversion in reverse water gas shift reaction†
Received
6th April 2022
, Accepted 5th August 2022
First published on 6th August 2022
Abstract
The bicomponent core–shell catalyst HZSM-5@ZrO2–In2O3 was synthesized via the decomposition of In(NO3)3/HZSM-5@UIO-66, which was obtained by impregnating HZSM-5@UIO-66 with In(NO3)3 solution. The bimetallic oxide particles of ZrO2–In2O3 were formed through the simultaneous decomposition of UIO-66 and In(NO3)3, and were anchored to the surface of the HZSM-5 core. The HZSM-5@ZrO2–In2O3 catalyst exhibited 31.1% CO2 conversion with 96.3% CO selectivity at 400 °C for the reverse water gas shift reaction.
Introduction
With the rapid development of industry and increasing CO2 emissions, the CO2 concentration in the atmosphere has increased by 25% over the past 200 years, leading to severe environmental and ecological problems, such as the melting of glaciers, rising sea levels and climate change.1 Thus, it is vital to utilize CO2 to produce high-value-added fuels and chemicals for environmental improvement and industrial development. In recent years, green hydrogen has gradually become an economically and environmentally benign renewable energy,2 and using it to reduce CO2 is of great industrial value.3 CO, CH4 and CH3OH are three major products from the direct utilization of CO2. Among them, CO is widely used as a raw material for the further synthesis of alcohols,4 olefins,5 aromatics,6 and other value-added chemicals. Therefore, the hydrogenation of CO2 to CO is a promising way to reduce CO2.7
Catalysts for the reverse water gas shift (RWGS) reaction are roughly divided into two categories, which are described as follows. Noble metal catalysts (such as Au,8 Pt,9 Pd,10etc.) have a high catalytic activity and stability, but they are very expensive and generate CH4 as a non-negligible by-product; and non-noble metal catalysts (such as Fe,11 Zn,12 Ce,13etc.) are inexpensive but have a poor low-temperature activity. Hence many studies have focused on designing new catalysts to improve the catalytic performance of metals and oxides.14 Indium oxide (In2O3) has attracted extensive attention due to its surface redox properties and excellent activity for CO2 hydrogenation.15–19 Abundant oxygen vacancies on the surface of In2O3 play an essential role in the activation of CO2.20,21 Since In2O3 is easily loaded and modified, other precious metals22,23 or metal oxides24,25 have often been introduced to form more oxygen vacancies and active sites, promoting the activation of CO2 and H2. However, most studies involving In2O3 have focused on the hydrogenation of CO2 to methanol, and few studies have included the RWGS reaction.26
Metal–organic frameworks (MOFs) have wide applications in the field of catalysis27–31 owing to their tunable composition and structure. Furthermore, MOF derivatives not only avoid the disadvantage of weak coordination bonds between metal clusters and organic linkers but also effectively inhibit metal oxide agglomeration in the pyrolysis process.32 These factors are beneficial for fabricating stable catalysts with high dispersion, high specific surface area and high activity.28
Fig. 1 shows the typical assembly methods for catalysts. This work assembled the Zr-based MOF (UIO-66) and HZSM-5 via a solvothermal method to form a new composite HZSM-5@UIO-66. The HZSM-5@UIO-66 was impregnated with indium nitrate (In(NO3)3), followed by decomposition of the UIO-66 membrane and the adsorbed In(NO3)3 during calcination, resulting in the formation of the bicomponent core–shell catalyst HZSM-5@ZrO2–In2O3. Due to the highly synergistic effect of the bimetal ZrO2–In2O3 and the special core–shell structure of HZSM-5@ZrO2–In2O3, the catalyst exhibited high CO2 conversion (31.1%) and excellent CO selectivity (96.3%) at 400 °C, 3 MPa, and 4500 mL g−1 h−1.
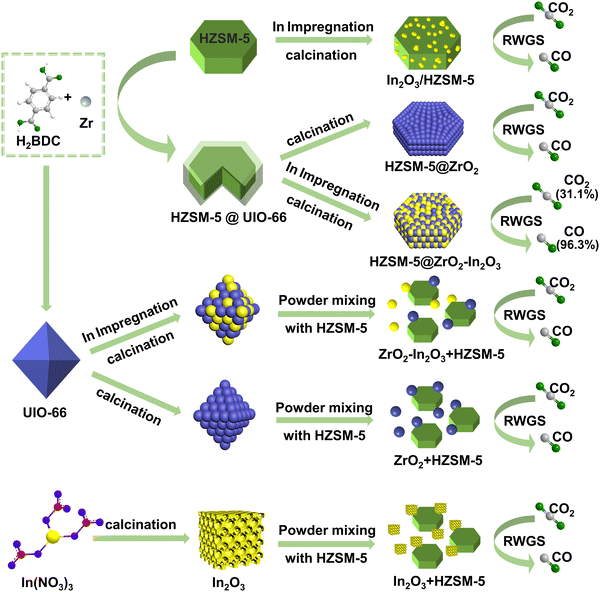 |
| Fig. 1 Assembly diagram of composites (from top to bottom) In2O3/HZSM-5, HZSM-5@ZrO2, HZSM-5@ZrO2–In2O3, ZrO2–In2O3 + HZSM-5, ZrO2 + HZSM-5, In2O3 + HZSM-5 and their effects on CO2 hydrogenation. | |
Results and discussion
Syntheses and characterizations of fresh catalysts
HZSM-5 has been used widely in various catalytic reactions due to its regular pore channels, large surface area and thermal stability.33,34 In Fig. 2, monodispersed HZSM-535 appears as smooth regular hexagonal prisms with a uniform size, while UIO-6636 appears as octahedra. The HZSM-5 was placed in the UIO-66 precursor solution as a shape-directed carrier, and the UIO-66 was epitaxially grown onto the HZSM-5 core to obtain HZSM-5@UIO-66 via a solvothermal method. The morphology of the HZSM-5 core was well retained, while the UIO-66 was neither a regular octahedra nor small crystalline grains;37 it was completely coated on HZSM-5 as a dense membrane layer (Fig. 2). The formation of the UIO-66 membrane can be attributed to the following factors. It has been confirmed that the solvent dipole moment can change the interfacial tension between the host nanoparticle and guest solvent, affecting the particle growth rate and ultimately changing the material morphology.38 Thus, ethanol (EtOH, μ = 1.68 D) with a different dipole moment than N,N-dimethylformamide (DMF, μ = 3.86 D) was added to the preparation solution of the UIO-66 membrane. The optimal volume ratio of the two above-mentioned solvents was confirmed to be 1
:
1.9,39 At this ratio, UIO-66 can grow uniformly along the epitaxial direction of the HZSM-5 core. In addition, the molar amounts of the metal sources (zirconium chloride (ZrCl4)) and organic linkers (terephthalic acid (H2BDC)) that constitute the UIO-66 had the most significant influence on the formation of the core–shell structure. The effect of n(ZrCl4) and n(H2BDC) on the growth of UIO-66 onto HZSM-5, under VEtOH/VDMF = 1 was investigated in this study. Fig. S2 (ESI†) shows the morphology of the UIO-66 grown on the HZSM-5 surface at different molar amounts. When n(ZrCl4) = n(H2BDC) = 0.035 mM, the UIO-66 membrane had a thickness of ∼10 nm, and there were many standalone spherical UIO-66 crystals of about 40 nm in diameter. This result was ascribed to the high concentrations of the precursors in the solution, which promoted the growth of UIO-66 on the HZSM-5 surface, and led to the independent nucleation of UIO-66 crystals. To obtain a homogeneous material, n(ZrCl4) and n(H2BDC) were further reduced, from 0.035 mM to 0.025 mM, upon which both the UIO-66 membrane thickness and the standalone UIO-66 crystal size gradually decreased. Finally, when n(ZrCl4) and n(H2BDC) were reduced to 0.020 mM, there were almost no standalone UIO-66 particles, and the HZSM-5 surface was evenly covered by a 4 nm membrane. The enlarged view of HZSM-5@UIO-66 (Fig. 2C and Fig. S3D, ESI†) showed a continuous UIO-66 membrane around the periphery of HZSM-5. Therefore, 0.020 mM could be regarded as the optimal molar amount for the UIO-66 precursor. Furthermore, these results demonstrated that the UIO-66 membrane thickness in HZSM-5@UIO-66 could be effectively controlled by adjusting the precursor molar amounts.
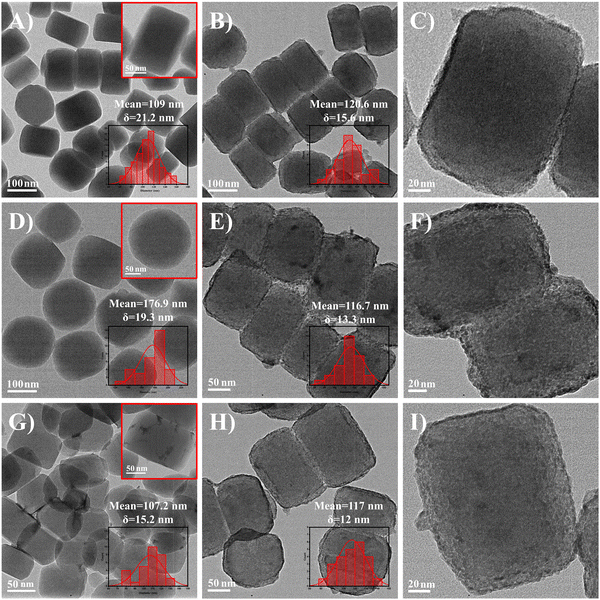 |
| Fig. 2 TEM images of different materials: (A) HZSM-5, (B and C) HZSM-5@UIO-66, (D) UIO-66, (E and F) HZSM-5@ZrO2–In2O3, (G) In2O3/HZSM-5, and (H and I) HZSM-5@ZrO2. | |
HZSM-5@ZrO2 and HZSM-5@ZrO2–In2O3 (Fig. 2) originated from the calcination of HZSM-5@UIO-66 and In(NO3)3/HZSM-5@UIO-66, respectively. During calcination, the organic linkers (H2BDC) in the UIO-66 membrane underwent oxidative decomposition with oxygen and, simultaneously, the UIO-66 membrane was converted to ZrO2.40 The disappearance of the organic linkers resulted in a certain volume contraction;41,42 therefore, HZSM-5@ZrO2 and HZSM-5@ZrO2–In2O3 were slightly smaller in size than HZSM-5@UIO-66. The presence of organic linkers constructed a temporary physical barrier for the ZrO2 nanoparticles, hindering their aggregation during calcination.40 The metal oxides ZrO2 and ZrO2–In2O3 that were obtained after calcination were anchored on the HZSM-5 surface in the form of particles (Fig. 2F and I) about 5 nm in diameter. In the enlarged view (Fig. 2F and I and Fig. S3F, ESI†), the oxide shell on the surface of HZSM-5 can clearly be seen. Furthermore, compared with HZSM-5@ZrO2–In2O3, only a small amount of In2O3 was loaded on the HZSM-5 (Fig. 2G) at the same In2O3 loading due to its worse adsorption than UIO-66. Clearly, coating UIO-66 on HZSM-5 could obtain ZrO2–In2O3 with a small size and the catalyst with a higher In2O3 loading. Hollow cubes of pure In2O3, granular ZrO2 and ZrO2–In2O3 were also prepared (Fig. S4, ESI†).
The HAADF-STEM image of HZSM-5@UIO-66 (Fig. 3A) showed a large difference in brightness between the middle and edge regions of the material, indicating a typical core–shell structure. Energy-dispersive X-ray (EDX) elemental mapping and line-scan EDX (Fig. 3B–I) demonstrated the structural composition and elemental distribution of HZSM-5@UIO-66. Si and Al were the main components of the HZSM-5 core, and O existed in the whole material. Zr and C, which formed UIO-66, appeared as a shell, indicating that UIO-66 was successfully coated on the surface of the HZSM-5 core. Moreover, EDX elemental mapping (Fig. S5, ESI†) of HZSM-5@ZrO2–In2O3 verified that In2O3 was successfully loaded on the surface of the material after impregnation.
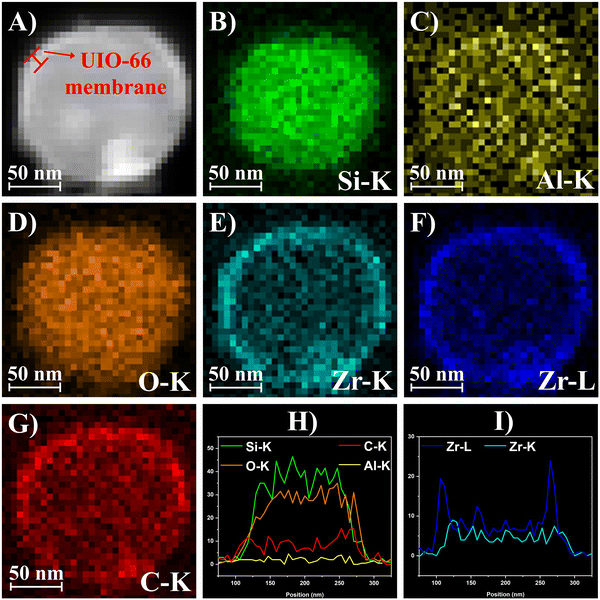 |
| Fig. 3 (A) HAADF-STEM image of HZSM-5@UIO-66, (B–G) EDX elemental mapping of the selected region, and (H and I) line-scan EDX of HZSM-5@UIO-66. | |
The N2 adsorption–desorption isotherms of UIO-66, HZSM-5, HZSM-5@UIO-66, and HZSM-5@ZrO2–In2O3 were obtained (Fig. S6A, ESI†). All isotherms were the typical type-I, meaning that all the samples were microporous. However, the pore size distribution image (Fig. S6B, ESI†) of HZSM-5@ZrO2–In2O3 showed the formation of mesopores. The disappearance of organic linkers in the UIO-66 membrane during calcination generated some extra spaces in the material, leading to mesopores.40,43 The Brunauer–Emmett–Teller (BET) surface area of HZSM-5@UIO-66 was 296 m2 g−1, which is lower than that of HZSM-5 (456 m2 g−1), and significantly lower than that of UIO-66 (1307 m2 g−1) (Table S1, ESI†). This is because HZSM-5 dominated the HZSM-5@UIO-66 composite, while the UIO-66 content was low. Furthermore, the UIO-66 membrane blocked part of the pores of HZSM-5 due to the mismatching aperture during preparation, which caused the BET surface area of HZSM-5@UIO-66 to be smaller than HZSM-5. HZSM-5@ZrO2–In2O3 still maintained a high BET specific surface area (309 m2 g−1).
Both UIO-66 and HZSM-5 showed diffraction peaks that matched their corresponding simulated powder X-ray diffraction (PXRD) patterns (Fig. S7, ESI†). However, for HZSM-5@UIO-66, only the characteristic peaks of HZSM-5 were observed. The absence of the characteristic peaks of UIO-66 might be attributed to its indistinguishability from HZSM-5 in the PXRD pattern and its low content in the composite. Although no UIO-66 diffraction peaks were observed in the PXRD pattern, Fourier transform infrared (FT-IR) spectroscopy (Fig. S9, ESI†) showed that both UIO-66 and HZSM-5@UIO-66 had the characteristic bands of UIO-66 at 1591, 1395 and 747 cm−1, confirming the existence of the UIO-66 membrane in HZSM-5@UIO-66. HZSM-5@UIO-66 was calcined in air to obtain HZSM-5@ZrO2, and its XRD pattern clearly showed the characteristic peaks of HZSM-5 and the highly crystalline tetragonal ZrO2 (t-ZrO2)44 derived from the UIO-66 membrane. The peaks of HZSM-5 and pure cubic In2O3 (c-In2O3, formed via the pyrolysis of In(NO3)3)45 were observed in In2O3/HZSM-5. By comparison with the precursors UIO-66 and HZSM-5@UIO-66, four new characteristic peaks (2θ = 25–70°) appeared in ZrO2–In2O3 and HZSM-5@ZrO2–In2O3. For ZrO2–In2O3, the characteristic peaks of UIO-66 disappeared completely due to the phase transition. The local spectra were magnified at 25–70° to verify the phase of the new peaks. For HZSM-5@ZrO2–In2O3 (with an In2O3 loading of 20 wt%), only the diffraction peaks of t-ZrO2 were observed because of impregnation loss and uniform distribution. When the In2O3 loading was increased to 70 wt%, the ZrO2 peaks grew accordingly and shifted towards a higher diffraction angle (the characteristic peaks of ZrO2–In2O3 (70 wt%) were the same as this), indicating that the loading was increased and some of the In2O3 was doped into ZrO2.46
The peaks of In and Zr in HZSM-5@ZrO2–In2O3 appeared at the corresponding positions of In3+ and Zr4+ (Fig. S10, ESI†), indicating the existence of oxides.47 Compared with pure ZrO2 and pure In2O3, new peaks appeared at higher binding energies. This phenomenon was ascribed to the change in the coordination states of In and Zr, which was caused by In doping in the ZrO2 lattice to form the In–O–Zr structure (solid solution). This result was consistent with the XRD, and the In doping could force O ions away to balance the charge, forming more oxygen vacancies.48 The O1s spectrum of HZSM-5@ZrO2–In2O3 also exhibited a higher concentration of oxygen vacancies than HZSM-5@ZrO2 (Fig. S10, ESI†).
Catalytic performance
For the prepared catalysts, three main products (CO, CH4 and CH3OH) were generated via the following three reactions. Since the appropriate reaction temperature for converting CO2 to methanol was below 300 °C, only trace amounts of methanol were detected at 400 °C. | CO2 + H2 ⇌ CO + H2O ΔH = 41.2 kJ mol−1 | (1) |
| CO2 + 4H2 ⇌ CH4 + H2O ΔH = −164.9 kJ mol−1 | (2) |
| CO2 + 3H2 ⇌ CH3OH + H2O ΔH = −49.4 kJ mol−1 | (3) |
In Fig. 4A, In2O3/HZSM-5 (where HZSM-5 was directly impregnated without coating with UIO-66) showed an inferior catalytic performance with 12.7% CO2 conversion, and HZSM-5@ZrO2 (where HZSM-5 was coated with UIO-66 but not impregnated) showed the lowest activity with 7.1% CO2 conversion. However, HZSM-5@ZrO2–In2O3 exhibited a superior CO2 conversion (27.8%). On the one hand, the excellent adsorption and special pore structure of UIO-66 enabled HZSM-5@ZrO2–In2O3 to have more active sites (Table S2, ESI†). On the other hand, the synergy of the ZrO2–In2O3 bimetal played a crucial role in activating CO2. To verify the improved catalytic performance of the bimetallic ZrO2–In2O3 shell compared with the monometallic oxide, In2O3 + HZSM-5 and ZrO2 + HZSM-5 were prepared (monometallic In2O3 and monometallic ZrO2 were mixed with HZSM-5, respectively). The CO2 conversion of HZSM-5@ZrO2–In2O3 was about 2.5 and 3.4 times that of In2O3 + HZSM-5 and ZrO2 + HZSM-5, respectively, suggesting a strong synergy between ZrO2 and In2O3. The introduction of ZrO2 can form the Zr–In interface and more oxygen vacancies,49 significantly improving the adsorption and hydrogenation of CO2.50,51 In addition, materials with a core–shell structure have been shown to be superior catalysts in many reactions.52 Hence for comparison, a mixed ZrO2–In2O3 + HZSM-5 catalyst containing the same active oxide content as HZSM-5@ZrO2–In2O3 was also prepared according to the ICP results (Table S2, ESI†). However, ZrO2–In2O3 + HZSM-5 afforded a lower CO2 conversion (22.6%) and CO yield (22.2%) than HZSM-5@ZrO2–In2O3 (27.8%, 26.9%), suggesting that besides the same active sites, the core–shell structure of the HZSM-5@ZrO2–In2O3 catalyst also plays an important role in this reaction. The physical mixing of ZrO2–In2O3 and HZSM-5 was disordered, and the core–shell structure of ZrO2–In2O3 and HZSM-5 improved the distribution of the ZrO2–In2O3 components on the HZSM-5 surface. Although ZrO2–In2O3 + HZSM-5 was not as active as HZSM-5@ZrO2–In2O3, it still exhibited a higher CO2 conversion and CO yield compared with the monometallic oxides (In2O3 + HZSM-5 and ZrO2 + HZSM-5), which further proved the excellent catalytic performance of the MOF-derived bimetallic oxide in the RWGS reaction.
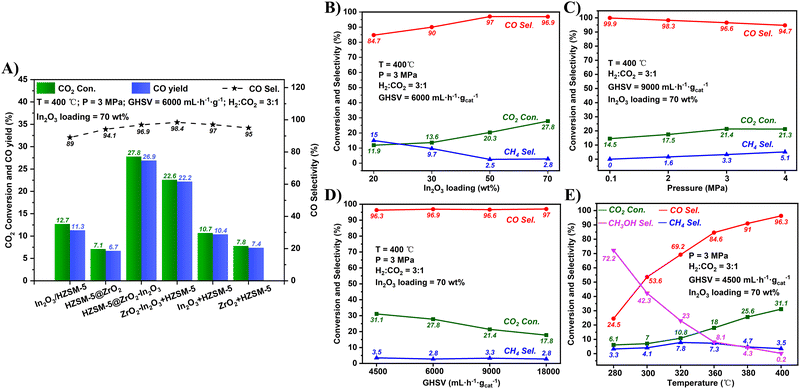 |
| Fig. 4 Catalytic performance of (A) the various catalysts, (B) In2O3 loadings, (C) reaction pressures, (D) GHSV, and (E) reaction temperatures. | |
As shown in Fig. 4B, with the increase in In2O3 loading from 20 wt% to 70 wt%, the CO2 conversion increased from 11.9% to 27.8%. The higher the In2O3 amount, the more the number of ZrO2–In2O3 active sites. In addition, the operation conditions also have a significant influence on this reaction. Fig. 4C–E shows the influence of the pressure, space velocity (GHSV), and reaction temperature on the catalyst performance. When the pressure was 0.1 MPa, the CO2 conversion was 14.5% with 99.9% CO selectivity. When the pressure was increased from 2 to 3 MPa, the CO2 conversion increased from 17.5% to 21.4%. When the pressure was raised to 4 MPa, the CO2 conversion hardly changed, but the CO selectivity decreased to 94.7%. The increase in pressure typically leads to increased collisions between the reactant gas molecules, activating more molecules, and promoting the adsorption of activated molecules on the catalyst surface, thereby increasing the CO2 conversion. Moreover, the CO2 methanation side-reaction has a reduced amount of gas molecules, and the high pressure tends to promote the side-reaction, thus decreasing the CO selectivity. With the GHSV increasing from 4500 to 18
000 mL h−1 gcat−1, the CO2 conversion decreased from 31.1% to 17.8%, and the CO/CH4 selectivity remained at around 97%/3%. The reduction in GHSV increased the residence time of the reactant gas in the catalyst bed, thus enhancing the CO2 conversion. When the temperature was gradually increased from 280 to 400 °C, the CO2 conversion increased from 6.1% to 31.1%. This is because CO2 is inert and a high temperature is beneficial for activating CO2. The CH3OH selectivity decreased from 72% to 0.2% within this temperature range, while the CO selectivity increased from 24.5% to 96.3%. The RWGS reaction is a typical endothermic reaction, whereas the conversion of CO2 to methanol is typically exothermic; hence increasing the temperature transforms the product from CH3OH to CO. Based on the above discussions, the optimal reaction conditions for the HZSM-5@ZrO2–In2O3 catalyst (In2O3 loading of 70 wt%) were as follows: 400 °C, 3 MPa, and 4500 mL h−1 gcat−1. Under these conditions, the CO2 conversion and CO selectivity were 31.1% and 96.3%, respectively. Furthermore, only a small amount of methanol intermediate was generated due to the high reaction temperature, and the UIO-66 membrane might occupy the acidic sites of HZSM-5. Therefore, despite the presence of HZSM-5 in the catalysts, only very small amounts of hydrocarbons were detected in the product, while the main product was CO. Table S3 (ESI†) compares HZSM-5@ZrO2–In2O3 with other catalysts, including metal oxides and noble metal catalysts, in the RWGS reaction. Both the CO2 conversion and CO selectivity of HZSM-5@ZrO2–In2O3 are outstanding. The special core–shell structure and the full synergistic effect of bimetallic ZrO2–In2O3 play a critical role in enhancing the performance. In addition, compared with noble metal catalysts, In-based catalysts have lower costs and, thus, better practical application prospects in industries.
Catalytic mechanism
There are roughly two reaction mechanisms for the RWGS reaction, one of which is the redox mechanism.53 In this process, H2 does not directly participate in forming intermediates but acts only as a reducing agent to reduce the catalyst (CO2 + M0 → MOx + CO; H2 + MOx → M0 + H2O). Since the main product with the HZSM-5@ZrO2–In2O3 catalyst was CH3OH at low temperature, the redox reaction was first excluded. The second most common mechanism is the degradation mechanism of the formate intermediate (CO2 → *HCOO → CO* → CO).54,55 In this pathway, H2 is involved in the formation of the intermediate *HCOO. CO2 is hydrogenated to the intermediate *HCOO on the catalyst surface, and the intermediate further decomposes to form CO. The combination of ZrO2 and In2O3 increases the oxygen vacancies on the oxide surface, and the most significant role of the oxygen vacancies is in the adsorption and activation of CO2.21,56 Adsorbed CO2 is further hydrogenated to the formate intermediate. This mechanism can explain the product transformation due to the change in temperature: formate is further hydrogenated to methanol at low temperature whereas it is directly pyrolyzed to CO at high temperature.
Conclusions
In conclusion, the bicomponent core–shell catalyst HZSM-5@ZrO2–In2O3 was synthesized via the decomposition of In(NO3)3/HZSM-5@UIO-66, which was obtained by impregnating HZSM-5@UIO-66 with the In(NO3)3 solution. Under the synergistic effect of bimetallic ZrO2–In2O3 derived from the UIO-66 membrane and In(NO3)3, the HZSM-5@ZrO2–In2O3 catalyst with a special core–shell structure afforded excellent CO2 conversion (31.1%) and CO selectivity (96.3%). The traditional concept is that the bimetallic oxide ZrO2–In2O3 is suitable for CO2 hydrogenation to methanol. This work reveals the superior catalytic performance of bimetallic ZrO2–In2O3 derived from MOF towards the RWGS reaction, and provides a novel fabrication strategy for future catalysts for effectively catalysing CO2 hydrogenation to CO.
Author contributions
Under Haitao Xu's guidance, Huimin Fang performed the experiments, collected and analyzed the data, then wrote the draft of the manuscript. Qi Jiang completed the XRD characterization, and the STEM characterization was completed by Dengpeng Lan. Denghui Cheng and Xuqiang Liu assisted in the experimental process. Haitao Xu and Guofeng Zhao revised the article. The manuscript was reviewed by Jichang Liu, Jianping Ge, and Zhenliang Xu. All the authors endorsed the final version of the manuscript.
Conflicts of interest
There are no conflicts to declare.
Acknowledgements
This work was supported by financial support from the Joint Fund by the National Natural Science Foundation of China and PetroChina (Project U1862204), the National Natural Science Foundation of China (No. 21371058 and No. 22179038) and the Special Project for Peak Carbon Dioxide Emissions-Carbon Neutrality (21DZ1206700) from the Shanghai Municipal Science and Technology Commission. The authors are grateful to the Research Center of Analysis and Testing, East China University of Science and Technology, and Prof. Jianping Ge from the Shanghai Key Laboratory of Green Chemistry of Chemical Processes, East China Normal University, for help in the transmission electron microscopy and high angle annular dark-field scanning transmission electron microscopy analysis.
Notes and references
- J. Hansen, M. Sato, R. Ruedy, K. Lo, W. Lea David and M. Medina-Elizade, Global temperature change, Proc. Natl. Acad. Sci. U. S. A., 2006, 103, 14288–14293 CrossRef CAS PubMed.
- P. Lianos, Review of recent trends in photoelectrocatalytic conversion of solar energy to electricity and hydrogen, Appl. Catal., B, 2017, 210, 235–254 CrossRef CAS.
- G. Centi, E. A. Quadrelli and S. Perathoner, Catalysis for CO2 conversion: a key technology for rapid introduction of renewable energy in the value chain of chemical industries, Energy Environ. Sci., 2013, 6, 1711–1731 RSC.
- B. Liu, Y. Li, Y. Duan, T. Ding, Y. Tang and C. Zheng, Effect of supports on performance of Cu–Fe based catalysts for higher alcohols synthesis from syngas, React. Kinet., Mech. Catal., 2019, 128, 695–706 CrossRef CAS.
- F. Jiao, J. Li, X. Pan, J. Xiao, H. Li, H. Ma, M. Wei, Y. Pan, Z. Zhou, M. Li, S. Miao, J. Li, Y. Zhu, D. Xiao, T. He, J. Yang, F. Qi, Q. Fu and X. Bao, Selective conversion of syngas to light olefins, Science, 2016, 351, 1065–1068 CrossRef CAS PubMed.
- K. Cheng, W. Zhou, J. Kang, S. He, S. Shi, Q. Zhang, Y. Pan, W. Wen and Y. Wang, Bifunctional Catalysts for One-Step Conversion of Syngas into Aromatics with Excellent Selectivity and Stability, Chem, 2017, 3, 334–347 CAS.
- X. Zhang, X. Zhu, L. Lin, S. Yao, M. Zhang, X. Liu, X. Wang, Y.-W. Li, C. Shi and D. Ma, Highly Dispersed Copper over β-Mo2C as an Efficient and Stable Catalyst for the Reverse Water Gas Shift (RWGS) Reaction, ACS Catal., 2017, 7, 912–918 CrossRef CAS.
- L. C. Wang, M. Tahvildar Khazaneh, D. Widmann and R. J. Behm, TAP reactor studies of the oxidizing capability of CO2 on a Au/CeO2 catalyst – A first step toward identifying a redox mechanism in the Reverse Water–Gas Shift reaction, J. Catal., 2013, 302, 20–30 CrossRef CAS.
- Y. Wu, D. Lan, J. Liu, J. Ge, H. Xu, Y. Han, H. Zhang, X. Pan, Z. Xu and J. Liu, UIO66-membranized SAPO-34 Pt catalyst for enhanced carbon dioxide conversion efficiency, Mater. Today, Energy, 2021, 21, 100781 CAS.
- N. C. Nelson, L. Chen, D. Meira, L. Kovarik and J. Szanyi, In Situ Dispersion of Palladium on TiO2 During Reverse Water–Gas Shift Reaction: Formation of Atomically Dispersed Palladium, Angew. Chem., Int. Ed., 2020, 59, 17657–17663 CrossRef CAS PubMed.
- D. H. Kim, S. W. Han, H. S. Yoon and Y. D. Kim, Reverse water gas shift reaction catalyzed by Fe nanoparticles with high catalytic activity and stability, J. Ind. Eng. Chem., 2015, 23, 67–71 CrossRef CAS.
- J. Wen, C. Huang, Y. Sun, L. Liang, Y. Zhang, Y. Zhang, M. Fu, J. Wu, L. Chen and D. Ye, The Study of Reverse Water Gas Shift Reaction Activity over Different Interfaces: The Design of Cu-Plate ZnO Model Catalysts, Catalysts, 2020, 10(5), 533 CrossRef CAS.
- B. Dai, G. Zhou, S. Ge, H. Xie, Z. Jiao, G. Zhang and K. Xiong, CO2 reverse water-gas shift reaction on mesoporous M-CeO2 catalysts, Can. J. Chem. Eng., 2017, 95, 634–642 CrossRef CAS.
- Y. Xu, M. Cao and Q. Zhang, Recent advances and perspective on heterogeneous catalysis using metals and oxide nanocrystals, Mater. Chem. Front., 2021, 5, 151–222 RSC.
- K. Sun, Z. Fan, J. Ye, J. Yan, Q. Ge, Y. Li, W. He, W. Yang and C.-J. Liu, Hydrogenation of CO2 to methanol over In2O3 catalyst, J. CO2 Util., 2015, 12, 1–6 CrossRef CAS.
- P. Gao, S. Li, X. Bu, S. Dang, Z. Liu, H. Wang, L. Zhong, M. Qiu, C. Yang, J. Cai, W. Wei and Y. Sun, Direct conversion of CO2 into liquid fuels with high selectivity over a bifunctional catalyst, Nat. Chem., 2017, 9, 1019–1024 CrossRef CAS PubMed.
- P. Gao, S. Dang, S. Li, X. Bu, Z. Liu, M. Qiu, C. Yang, H. Wang, L. Zhong, Y. Han, Q. Liu, W. Wei and Y. Sun, Direct Production of Lower Olefins from CO2 Conversion via Bifunctional Catalysis, ACS Catal., 2018, 8, 571–578 CrossRef CAS.
- S. Wang, P. Wang, Z. Qin, W. Yan, M. Dong, J. Li, J. Wang and W. Fan, Enhancement of light olefin production in CO2 hydrogenation over In2O3-based oxide and SAPO-34 composite, J. Catal., 2020, 391, 459–470 CrossRef CAS.
- S. Dang, P. Gao, Z. Liu, X. Chen, C. Yang, H. Wang, L. Zhong, S. Li and Y. Sun, Role of zirconium in direct CO2 hydrogenation to lower olefins on oxide/zeolite bifunctional catalysts, J. Catal., 2018, 364, 382–393 CrossRef CAS.
- J. Ye, C. Liu, D. Mei and Q. Ge, Active Oxygen Vacancy Site for Methanol Synthesis from CO2 Hydrogenation on In2O3(110): A DFT Study, ACS Catal., 2013, 3, 1296–1306 CrossRef CAS.
- J. Wang, G. Zhang, J. Zhu, X. Zhang, F. Ding, A. Zhang, X. Guo and C. Song, CO2 Hydrogenation to Methanol over In2O3-Based Catalysts: From Mechanism to Catalyst Development, ACS Catal., 2021, 11, 1406–1423 CrossRef CAS.
- K. Sun, N. Rui, Z. Zhang, Z. Sun, Q. Ge and C.-J. Liu, A highly active Pt/In2O3 catalyst for CO2 hydrogenation to methanol with enhanced stability, Green Chem., 2020, 22, 5059–5066 RSC.
- N. H. M. D. Dostagir, C. Thompson, H. Kobayashi, A. M. Karim, A. Fukuoka and A. Shrotri, Rh promoted In2O3 as a highly active catalyst for CO2 hydrogenation to methanol, Catal. Sci. Technol., 2020, 10, 8196–8202 RSC.
- C. Y. Regalado Vera, N. Manavi, Z. Zhou, L.-C. Wang, W. Diao, S. Karakalos, B. Liu, K. J. Stowers, M. Zhou, H. Luo and D. Ding, Mechanistic understanding of support effect on the activity and selectivity of indium oxide catalysts for CO2 hydrogenation, Chem. Eng. J., 2021, 426, 131767 CrossRef CAS.
- W. Wang, Y. Zhang, Z. Wang, J.-M. Yan, Q. Ge and C.-J. Liu, Reverse water gas shift over In2O3–CeO2 catalysts, Catal. Today, 2016, 259, 402–408 CrossRef CAS.
- Q. Sun, J. Ye, C.-J. Liu and Q. Ge, In2O3 as a promising catalyst for CO2 utilization: A case study with reverse water gas shift over In2O3, Greenhouse Gases: Sci. Technol., 2014, 4, 140–144 CrossRef CAS.
- N. Martín, A. Portillo, A. Ateka, F. G. Cirujano, L. Oar-Arteta, A. T. Aguayo and M. Dusselier, MOF-derived/zeolite hybrid catalyst for the production of light olefins from CO2, ChemCatChem, 2020, 12, 5750–5758 CrossRef.
- A. Pustovarenko, A. Dikhtiarenko, A. Bavykina, L. Gevers, A. Ramírez, A. Russkikh, S. Telalovic, A. Aguilar, J.-L. Hazemann, S. Ould-Chikh and J. Gascon, Metal–Organic Framework-Derived Synthesis of Cobalt Indium Catalysts for the Hydrogenation of CO2 to Methanol, ACS Catal., 2020, 10, 5064–5076 CrossRef CAS.
- Z. Cai, J. Dai, W. Li, K. B. Tan, Z. Huang, G. Zhan, J. Huang and Q. Li, Pd Supported on MIL-68(In)-Derived In2O3 Nanotubes as Superior Catalysts to Boost CO2 Hydrogenation to Methanol, ACS Catal., 2020, 10, 13275–13289 CrossRef CAS.
- Y. Wang, W. Zhan, Z. Chen, J. Chen, X. Li and Y. Li, Advanced 3D Hollow-Out ZnZrO@C Combined with Hierarchical Zeolite for Highly Active and Selective CO Hydrogenation to Aromatics, ACS Catal., 2020, 10, 7177–7187 CrossRef CAS.
- L.-L. Ling, W. Yang, P. Yan, M. Wang and H.-L. Jiang, Light-Assisted CO2 Hydrogenation over Pd3Cu@UiO-66 Promoted by Active Sites in Close Proximity, Angew. Chem., Int. Ed., 2022, 61, e202116396 CAS.
- Y.-Z. Chen, R. Zhang, L. Jiao and H.-L. Jiang, Metal–organic framework-derived porous materials for catalysis, Coord. Chem. Rev., 2018, 362, 1–23 CrossRef CAS.
- Y. Yu, Z. Xi, B. Zhou, B. Jiang, Z. Liao, Y. Yang, J. Wang, Z. Huang, J. Sun and Y. Yang, Acidity Modification of ZSM-5 for Methane Conversion in Co-feeding Method with MTA Reaction, Chem. Res. Chin. Univ., 2022, 38, 1012–1017 CrossRef CAS.
- L. Luo, S. Wang, Z. Wu, Z. Qin, M. Dong, J. Wang and W. Fan, Influence of the ZSM-5 Support Acidity on the Catalytic Performance of Pd/ZSM-5 in Lean Methane Oxidation, Chem. Res. Chin. Univ., 2022, 38, 229–236 CrossRef CAS.
- Z. Wan, W. Wu, G. Li, C. Wang, H. Yang and D. Zhang, Effect of SiO2/Al2O3 ratio on the performance of nanocrystal ZSM-5 zeolite catalysts in methanol to gasoline conversion, Appl. Catal., A, 2016, 523, 312–320 CrossRef CAS.
- H. Xu, X. Luo, J. Wang, Y. Su, X. Zhao and Y. Li, Spherical Sandwich Au@Pd@UIO-67/Pt@UIO-n (n = 66, 67, 69) Core–Shell Catalysts: Zr-Based Metal–Organic Frameworks for Effectively Regulating the Reverse Water–Gas Shift Reaction, ACS Appl. Mater. Interfaces, 2019, 11, 20291–20297 CrossRef CAS PubMed.
- G. Zhu, R. Graver, L. Emdadi, B. Liu, K. Y. Choi and D. Liu, Synthesis of zeolite@metal–organic framework core–shell particles as bifunctional catalysts, RSC Adv., 2014, 4, 30673–30676 RSC.
- H. Bunzen, M. Grzywa, M. Hambach, S. Spirkl and D. Volkmer, From Micro to Nano: A Toolbox for Tuning Crystal Size and Morphology of Benzotriazolate-Based Metal–Organic Frameworks, Cryst. Growth Des., 2016, 16, 3190–3197 CrossRef CAS.
- X. Pan, H. Xu, X. Zhao and H. Zhang, Metal–Organic Framework-Membranized Bicomponent Core–Shell Catalyst HZSM-5@UIO-66-NH2/Pd for CO2 Selective Conversion, ACS Sustainable Chem. Eng., 2020, 8, 1087–1094 CrossRef CAS.
- J. Liu, Y. He, L. Yan, K. Li, C. Zhang, H. Xiang, X. Wen and Y. Li, Nano-sized ZrO2 derived from metal–organic frameworks and their catalytic performance for aromatic synthesis from syngas, Catal. Sci. Technol., 2019, 9, 2982–2992 RSC.
- G. Huang, F. Zhang, X. Du, J. Wang, D. Yin and L. Wang, Core–Shell NiFe2O4@TiO2 Nanorods: An Anode Material with Enhanced Electrochemical Performance for Lithium-Ion Batteries, Chem. – Eur. J., 2014, 20, 11214–11219 CrossRef CAS PubMed.
- L. Zhang, H. B. Wu, S. Madhavi, H. H. Hng and X. W. Lou, Formation of Fe2O3 Microboxes with Hierarchical Shell Structures from Metal–Organic Frameworks and Their Lithium Storage Properties, J. Am. Chem. Soc., 2012, 134, 17388–17391 CrossRef CAS PubMed.
- G. Qu, P. Jia, T. Zhang, Z. Li, C. Chen and Y. Zhao, UiO-66(Zr)-derived t-zirconia with abundant lattice defect for remarkably enhanced arsenic removal, Chemosphere, 2022, 288, 132594 CrossRef CAS.
- T. Numpilai, P. Kidkhunthod, C. K. Cheng, C. Wattanakit, M. Chareonpanich, J. Limtrakul and T. Witoon, CO2 hydrogenation to methanol at high reaction temperatures over In2O3/ZrO2 catalysts: Influence of calcination temperatures of ZrO2 support, Catal. Today, 2021, 375, 298–306 CrossRef CAS.
- J. Wang, C.-Y. Liu, T. P. Senftle, J. Zhu, G. Zhang, X. Guo and C. Song, Variation in the In2O3 Crystal Phase Alters Catalytic Performance toward the Reverse Water Gas Shift Reaction, ACS Catal., 2020, 10, 3264–3273 CrossRef CAS.
- C. Yang, C. Pei, R. Luo, S. Liu, Y. Wang, Z. Wang, Z.-J. Zhao and J. Gong, Strong Electronic Oxide–Support Interaction over In2O3/ZrO2 for Highly Selective CO2 Hydrogenation to Methanol, J. Am. Chem. Soc., 2020, 142, 19523–19531 CrossRef CAS PubMed.
- M. M. Zain, M. Mohammadi, N. Kamiuchi and A. R. Mohamed, Development of highly selective In2O3/ZrO2 catalyst for hydrogenation of CO2 to methanol: An insight into the catalyst preparation method, Korean J. Chem. Eng., 2020, 37, 1680–1689 CrossRef CAS.
- Z. Zhang, Y. Huang, H. Ma, W. Qian, H. Zhang and W. Ying, Syngas-to-olefins over MOF-derived ZnZrOx and SAPO-34 bifunctional catalysts, Catal. Commun., 2021, 152, 106292 CrossRef CAS.
- M. Zhang, M. Dou and Y. Yu, Theoretical study of the promotional effect of ZrO2 on In2O3 catalyzed methanol synthesis from CO2 hydrogenation, Appl. Surf. Sci., 2018, 433, 780–789 CrossRef CAS.
- M. Dou, M. Zhang, Y. Chen and Y. Yu, Mechanistic Insight into the Modification of the Surface Stability of In2O3 Catalyst Through Metal Oxide Doping, Catal. Lett., 2018, 148, 3723–3731 CrossRef CAS.
- O. Martin, A. J. Martín, C. Mondelli, S. Mitchell, T. F. Segawa, R. Hauert, C. Drouilly, D. Curulla-Ferré and J. Pérez-Ramírez, Indium Oxide as a Superior Catalyst for Methanol Synthesis by CO2 Hydrogenation, Angew. Chem., Int. Ed., 2016, 55, 6261–6265 CrossRef CAS PubMed.
- Z. Wang, J. Qi, N. Yang, R. Yu and D. Wang, Core–shell nano/microstructures for heterogeneous tandem catalysis, Mater. Chem. Front., 2021, 5, 1126–1139 RSC.
- G.-C. Wang and J. Nakamura, Structure Sensitivity for Forward and Reverse Water-Gas Shift Reactions on Copper Surfaces: A DFT Study, J. Phys. Chem. Lett., 2010, 1, 3053–3057 CrossRef CAS.
- C.-S. Chen, W.-H. Cheng and S.-S. Lin, Mechanism of CO formation in reverse water–gas shift reaction over Cu/Al2O3 catalyst, Catal. Lett., 2000, 68, 45–48 CrossRef CAS.
- A. M. Bahmanpour, M. Signorile and O. Kröcher, Recent progress in syngas production via catalytic CO2 hydrogenation reaction, Appl. Catal., B, 2021, 295, 120319 CrossRef CAS.
- Y. Zhang, L. Liang, Z. Chen, J. Wen, W. Zhong, S. Zou, M. Fu, L. Chen and D. Ye, Highly efficient Cu/CeO2-hollow nanospheres catalyst for the reverse
water-gas shift reaction: Investigation on the role of oxygen vacancies through in situ UV-Raman and DRIFTS, Appl. Surf. Sci., 2020, 516, 146035 CrossRef CAS.
Footnote |
† Electronic supplementary information (ESI) available: Chemicals, general methods, characterization, procedures and additional figures. See DOI: https://doi.org/10.1039/d2qm00307d |
|
This journal is © the Partner Organisations 2022 |