DOI:
10.1039/D2QI01271E
(Research Article)
Inorg. Chem. Front., 2022,
9, 5788-5798
Nanocage-based {In2Tm2}-organic framework for efficiently catalyzing the cycloaddition reaction of CO2 with epoxides and Knoevenagel condensation†
Received
16th June 2022
, Accepted 13th September 2022
First published on 14th September 2022
Abstract
High density active sites and chemical stability for prepared MOFs are the prerequisites for their industrial applications, which prompted us to synthesize cluster-based metal–organic compounds. The combination of [In2Tm2(μ2-OH)2(CO2)10(H2O)2] clusters ({In2Tm2}) and the functional ligand 2,6-bis(2,4-dicarboxylphenyl)-4-(4-carboxylphenyl)pyridine (H5BDCP) produced an extremely stable nanoporous skeleton of {(Me2NH2)2[In2Tm2(BDCP)2(μ2-OH)2(H2O)2]·2DMF·3H2O}n (NUC-56), whose inner surface is functionalized by Lewis acidic and basic groups of metal centers, μ2-OH groups and Npyridine atoms. To the best of our knowledge, NUC-56 is a rarely reported planar-cluster-based {In2Ln2}-organic framework, which possesses an excellent confined pore environment with high porosity, large specific surface area and abundant co-existing Lewis acid and base sites. Consequently, NUC-56a exhibited a good catalytic performance for the cycloaddition reaction of various epoxides with CO2 and the Knoevenagel condensation reaction of different aldehydes and malononitrile under mild conditions. Therefore, this study can provide some guidance for the precise design of practical MOFs with excellent catalytic, stability and regeneration performances through the participation of rare earth ions.
Introduction
Metal organic frameworks (MOFs), as a new type of porous inorganic–organic hybrid crystalline materials constructed from the exquisite combination of metal ions/clusters and diverse organic ligands, have attracted tremendous attention due to their desirable structural properties including adjustable pore size, high porosity, multiple active sites, and tailorable architectures, which have led to a wide range of applications, such as gas adsorption and separation, fluorescence sensing and heterogeneous catalysis.1–7 To date, there are relatively few reports on the applications of heterometallic frameworks due to the lack of mature synthetic regulation strategies. However, it has been proven that the introduction of a second metal ion in the framework is a valuable project to enhance the gas capture capacity and catalytic performance of MOFs because of the synergistic effect of two metal ions in the absence of specific mechanism processes.8–10 Therefore, the self-assembly of heterometallic MOFs with microchannels and extremely exposed active sites is particularly challenging, but can be achieved by carefully designing organic ligands, reasonably configuring different metal ions and finely controlling the growth environment.11,12
In addition, with the continuous expansion of industrial production and excessive use of fossil fuels, carbon dioxide (CO2) emissions have increased at a rate of 4% per year, resulting in serious air pollution and greenhouse effect.13–19 However, from the perspective of resource utilization, CO2 serves as one of the richest C1 resources on Earth with the advantages of large reserves, safety, non-toxicity and low price. Thus, converting CO2 into valuable chemicals has strategic significance of environmental friendliness and sustainability.20–23 Accordingly, it is significant for the sustainable development of society to implement strategies of CO2 capture and storage/sequestration (CCS), which have prompted the innovative preparation of functional microporous MOF-based materials.24–26 At present, the method for the preparation of cyclic carbonates by cycloaddition reaction using CO2 and epoxides is consistent with the concept of “green preparation theory” and “atomic economy principle”. Also, carbonate products are widely used in lithium battery electrolytes, textiles, chemical industry, pharmaceuticals and preparation of functional polymers because of their excellent properties including high boiling point and solubility, low toxicity and degradability.27–29 Nevertheless, owing to the thermodynamic stability of CO2, cycloaddition reactions usually demand a high reaction temperature and pressure. Thus, to overcome this disadvantageous problem, various homogeneous and heterogeneous catalysts have been developed to drive the reaction process. Among them, homogeneous catalysts have some drawbacks, such as difficult separation between catalyst and product, high energy consumption, and poor economic performance.30–32 Therefore, based on the above-mentioned problems, a variety of heterogeneous catalysts (ionic liquids, carrier solids, metal complexes, etc.) have been developed. However, solid heterogeneous catalysts usually need harsh conditions because they lack accessible surface area and exposed active sites to fully activate CO2 molecules. Thus, it is of great significance to explore innovative heterogeneous catalysts with excellent stability, cyclic performance, and high activity to promote the reaction under mild conditions.33–36 Thus far, MOFs have been widely used in the field of heterogeneous catalysis due to their permanent porosity, adjustable pore structure, large specific surface area, multiple reaction active sites and excellent ability to adsorb CO2.37–41 In-MOFs account for a small proportion of the reported MOFs although they are based on the most common SBUs, for instance, [In(CO2)n], [In3O(CO2)n(OH2)n], and [In(O/OH)]n and sporadic heterometallic clusters of {InM2}, {In2M2}, {In3Ln}, and {In3Ln2}.42–50 Nevertheless, the outstanding catalytic performance from the high-level p-orbitals of the In3+ ion provides motivation for scientists to pursue a superior indium-organic frame. In addition, Ln-MOFs have attracted increasing attention because of their high chemical stability and catalytic ability for most organic reactions, which should be attributed to the powerful positive charge of LnIII cations and abundant vacant electron orbitals.51,52 However, promising heterometallic MOFs based on the combination of rare earth ions and In3+ ions are rarely reported.
In view of the considerations discussed above, the multi-functional ligand of 2,6-bis(2,4-dicarboxylphenyl)-4-(4-carboxylphenyl)pyridine (H5BDCP) was reacted with In3+ and Tm3+ for the successful synthesis of a highly stable channel-based heterometallic {In2Tm2}-organic framework of {[In2Tm2(BDCP)2(μ2-OH)2(H2O)2]·2DMF·3H2O}n (NUC-56) with excellent water resistance, thermal stability and a series of structural advantages, which rendered it an outstanding heterogeneous catalyst to boost cycloaddition reactions. Moreover, it also simultaneously exhibited efficient catalytic ability in the Knoevenagel condensation of aldehydes and malononitrile.
Experimental
Preparation of NUC-56 and NUC-56a
A sample of Tm2O3 (0.10 mmol), In(NO3)3·5H2O (0.10 mmol) and H5BDCP (0.08 mmol) in 10 mL solvent of DMF and H2O (v
:
v = 8
:
2) with one drop of concentrated HNO3 was heated in a Teflon-lined stainless-steel vessel at 110 °C for 2 days. The cube crystals were collected by filtration after cooling, and the yield of crystals based on the H5BDCP ligand was 53%. Anal. calcd for NUC-56 (C40H26N3O17InTm): C, 24.64; H, 2.36; N, 3.81 (%). Found: C, 24.60; H, 2.40; N, 3.77 (%). FT-IR (KBr pellet, cm−1, Fig. S3†): 3750 (m), 2923 (w), 1857 (w), 1552 (w), 1500 (m), 1469 (m), 1415 (s), 1456 (s), 1089 (vs), 1014 (s), 921 (m), 858 (s), 819 (s), 777 (vs), 682 (m), 657 (m).
Results and discussion
Crystal structure description
Single-crystal X-ray diffraction analysis showed that NUC-56 crystallizes in a monoclinic system with the C2/m space group and exhibits a microporous three-dimensional framework of {[In2Tm2(BDCP)2(μ2-OH)2(H2O)2]·4DMF·3H2O}n with coplanar [In2Tm2(μ2-OH)2(CO2)10(H2O)2] clusters as the basic building units (Fig. 1a and Fig. S1†). Astonishingly, NUC-56 is a planar-cluster-based {In2Ln2}-organic framework, whose inner surface is functionalized by abundant coexisting Lewis acid–base sites of In3+, Tm3+, μ2-OH and Npyridine atoms. Moreover, it is noteworthy that the {In2Tm2} clusters exist in a coplanar configuration and NUC-56 is synthesized by the structurally oriented ligand of BDCP5−, forming a highly distorted connection mode, which resulted in the maximum exposure of the open metal sites of In3+ and Tm3+ and μ2-OH. Therefore, the successful construction of NUC-56 offers a valuable technique for the synthesis of deeply functionalized common In-MOFs by introducing rare earth ions, which potentially have wider applicability in various catalysis fields.
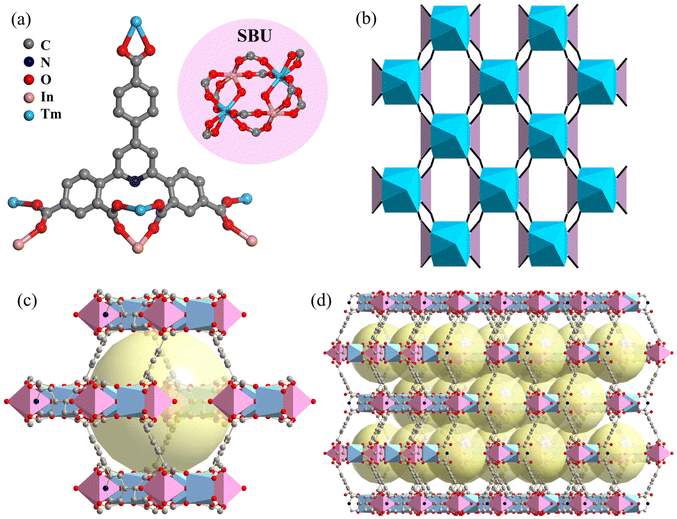 |
| Fig. 1 Coplanar [In2Tm2(μ2-OH)2(CO2)10(H2O)2] cluster and the coordination environment of BDCP5− ligand (a). Topology network with the Schläfli symbol of {412·612·84}{46}2 of NUC-56 (b). {In2Tm2} cluster-shaped nanocage of NUC-56 (c). 3D framework of NUC-56 featured by oval nanocages (d). | |
Regarding the detailed internal links in NUC-56, there is a half Tm3+ ion, a half In3+ ion, a half BDCP5−, two half μ2-OH and two half associated H2O in the asymmetric unit. The Tm(1) and In(1) ions are firstly bridged by two μ2–η1:η1 α-carboxyl groups from two phenyl rings arranged on the 2- and 6-position of pyridine in one BDCP5− to form a binuclear unit of [InTm(CO2)2] (Fig. 1a), which are further connected by one μ2-OH group, leaving a unit of [InTm(μ2-OH)(CO2)2]. Also, via the symmetry extension, two [InTm(μ2-OH)(CO2)2] units are each bridged by four μ2–η1:η1 γ-carboxyl groups from four phenyl rings arranged on the 2- and/or 6-position of pyridine of the BDCP5− ligand to form a 2D layer with the initial quadrangular [In2Tm2(μ2-OH)2(CO2)8(H2O)2] clusters as nodes, in which each adjacent Tm3+ and In3+ are bridged by two μ2–η1:η1 carboxyl groups, and each In3+ is saturated by one associated water molecule. Furthermore, each Tm3+ ion in the [In2Tm2(μ2-OH)2(CO2)8(H2O)2] SBU is further cheated by one γ-carboxyl group on the 4-position of the phenyl ring from the upper and lower planes to form a 3D anionic skeleton with the formation of a complete cluster of [In2Tm2(μ2-OH)2(CO2)10(H2O)2] (Fig. 1d). In conclusion, each tetranuclear heterometallic {In2Tm2} cluster is supported by ten carboxyl groups from eight deprotonated BDCP5− linkers. It is worth mentioning that the eight {In2Tm2} clusters are each woven together to generate elliptical nanocages (aperture ca. 14.1 Å) (Fig. 1c) with their functionalized inner surface decorated by the coexisting Lewis acid and base sites. Besides, the H5BDCP ligand has the two unique advantages, i.e., spatial self-regulation and metal-cluster-orientation. Because the triaxial angle-adjustable planar H5BDCP ligand enables three carboxylphenyl groups to rotate freely around the pyridine to obtain a more dynamic structure and the two α-carboxyl groups in the BDCP5− ligand play a structure-oriented role, causing the other carboxyl groups to grab other metal ions to form binuclear or polynuclear metal-cluster nodes, NUC-56 possesses excellent characteristics.
From a topological viewpoint, with BDCP5− and {In2Tm2} SBUs being seen as 4 and 8-c nodes, respectively, NUC-56 can be simplified as a novel 3D (4-c)2(8-c) topology with the Schläfli symbol of {412·612·84}{46}2 analyzed by TOPOS40, as shown in Fig. 1b.
Analysis of physicochemical properties
As shown in Fig. S2,† the PXRD pattern of NUC-56 and the simulated pattern derived from the single-crystal X-ray data are consistent, proving its phase purity. In addition, the PXRD modes show that NUC-56 has high water and chemical stability, which was proved by soaking it in water for 7 days at room temperature, 72 h in boiling water, and 7 h in water with a pH in the range of 2 to 12, as shown in Fig. S6 and S7.† The thermal stability of the as-prepared NUC-56 and NUC-56a samples was tested through thermogravimetric analysis experiment (TGA) in the temperature range of 25–800 °C (Fig. S5†), which showed a weight loss of ∼5.4% (calcd 5.0%) from 25 °C to 100 °C, corresponding to the loss of free H2O molecules. Next, the second loss of ∼23.5% (calcd 22.0%) corresponded to the loss of solvent and associated H2O molecules from 100 °C to 400 °C. Finally, NUC-56 was stable up to 450 °C with insignificant further weight loss observed, demonstrating its outstanding thermal stability.
Gas adsorption behaviour
To measure the permanent porosity of NUC-56a, the newly-synthesized compound was firstly activated by immersing it in CH3OH solvent for 3 days, and then heated under dynamic vacuum at 150 °C for 12 h, resulting in the de-solvated NUC-56a with abundant open metal active sites on the inner wall of its porous channels. Notably, the skeleton of the activated sample could still maintain its excellent integrity, which is supported by the PXRD pattern (Fig. S4†). The N2 adsorption isotherms of NUC-56a were measured at 77 K, as shown in Fig. S8.† The obtained results exhibited that NUC-56a exhibited the reversible classical Type-I isotherm, confirming that NUC-56 had microporous characteristics. The BET and Langmuir surface area were 807 m2 g−1 and 914 m2 g−1, respectively. Furthermore, the aperture distribution measurement showed that its pore size ranged from 8.1 to 14.3 Å, which illustrates that there were inhomogeneous micro-porous channels in the framework (Fig. S9†).53–55 Furthermore, NUC-56a also offered the maximum uptake capacity of CO2 with a value of 90.8 cm3 g−1 at 273 K and 53.5 cm3 g−1 at 298 K (Fig. S10†). To explain the binding force between CO2 and NUC-56a, the isosteric heat of CO2 adsorption (Qst) determined from the Clausius–Clapeyron equation was calculated to be 28.2 kJ mol−1 at zero loading (Fig. S11†).56–59
The distribution of acidic and basic sites in the NUC-56 catalyst was evaluated by TPD (temperature-programmed desorption) technology, as shown in Fig. 2. In the NH3-TDP profile, three groups of ammonia desorption peaks appeared in the temperature range of 100–450 °C, indicating that the NH3 desorption is from the Lewis acid sites.60,61 According to the amount of ammonia desorbed (the integral area of NH3-TDP), it is obvious that the amount of stronger Lewis acid sites is greater than weak Lewis basic sites (Fig. 2a). Similarly, it can be seen from the CO2-TPD profile in Fig. 2b that Lewis basic sites also exist on the surface of the NUC-56 catalyst. Also, the number of stronger basic sites is larger than that of weaker basic sites. The TDP results indicate that Lewis acid–base sites exist on the surface of NUC-56 simultaneously, which is helpful to improve its catalytic performance.
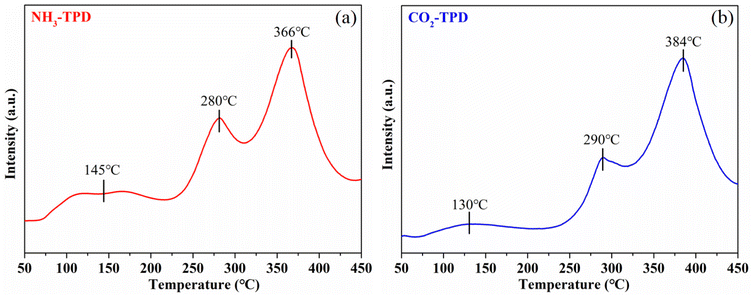 |
| Fig. 2 NH3-TPD (a) and CO2-TPD (b) profiles of NUC-56. | |
Catalytic cycloaddition of CO2 and epoxides
Motivated by the features of NUC-56a including high stability, large CO2 sorption capacity, accessible microporous channels, and coexisting high-density Lewis acid and base sites (In3+ and Ln3+, Npyridine atoms and μ2-OH groups), its heterogeneous catalysis of the cycloaddition reaction was evaluated by using styrene oxide (SO) as a model substrate under solvent-free and mild conditions. Further studies on the reaction temperature, time and catalyst dosage were carried out to optimize the reaction conditions. As described in Table 1, only a few of the reaction substrates could be converted to the target product at 7% yield in the presence of 0.05 mol% NUC-56a at ambient temperature. In contrast, 5% yield was observed when only 1 mol% co-catalyst of n-Bu4NBr as a catalyst was introduced for the cycloaddition reaction, as shown in entry 2. However, when 0.05 mol% of NUC-56a and 1 mol% of co-catalyst of n-Bu4NBr were introduced in the cycloaddition reaction under the same reaction conditions, the product yield significantly increased to 23%, indicating that the cycloaddition reaction was completed by the synergistic catalysis of NUC-56a and n-Bu4NBr (entry 3). Besides, with an increase in temperature, the conversion rate increased rapidly with high yield, and when the temperature reached 65 °C, the yield of styrene carbonate (SC) reached 96%, implying that the optimum reaction temperature should be set at 65 °C (entries 4–7). Furthermore, the influence of n-Bu4NBr dosage on the SC yield was investigated. As shown in entries 8–11, as the amount of co-catalyst n-Bu4NBr increased from 1 mol% to 5 mol%, the time taken to produce almost the same yield of SC gradually decreased from 48 h to 12 h. Consequently, the influence of NUC-56a dosage was discussed, and the data exhibited that the reaction time was reduced from 12 h to 7 h with the NUC-56a dosage from 0.06 to 0.10 mol%, exhibiting that the amount of catalyst has a significant influence on the reaction rate (entries 12–16). In addition, a set of control experiments conducted at 65 °C for 7 h using only 5.0 mol% n-Bu4NBr or 0.10 mol% NUC-56a as a new catalytic system exhibited a significant reduction in yield, indicating that the combination of NUC-56a and n-Bu4NBr played a very important role in the catalytic process (entries 17 and 18). Thus, it was concluded from the above investigations that the optimum catalytic conditions are 0.10 mol% NUC-56a, 5 mol% n-Bu4NBr, 1 atm CO2, 65 °C and 7 h. As shown in Table S3,† compared with some reports on MOF-based catalytic systems, it is noteworthy that the combination of NUC-56a and n-Bu4NBr showed an excellent catalytic effect due to the structural advantages of NUC-56a, including high CO2 adsorption capacity, plentiful Lewis acid and base sites and stable structure.
Table 1 Cycloaddition reaction of CO2 with styrene oxide under various conditionsa
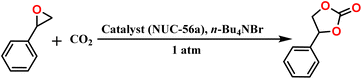
|
Entry |
NUC-56a (mol%) |
n-Bu4NBr (mol%) |
Temp. (°C) |
Time (h) |
Yieldb (%) |
Reaction conditions: 20 mmol styrene oxide, solvent free, CO2 (1 atm).
The n-dodecane was internal standard and checked by 1H NMR and GC-MS spectroscopy.
|
1 |
0.05 |
0 |
rt |
48 |
7 |
2 |
0 |
1 |
rt |
48 |
5 |
3 |
0.05 |
1 |
rt |
48 |
23 |
4 |
0.05 |
1 |
35 |
48 |
39 |
5 |
0.05 |
1 |
45 |
48 |
66 |
6 |
0.05 |
1 |
55 |
48 |
89 |
7 |
0.05 |
1 |
65 |
48 |
96 |
8 |
0.05 |
2 |
65 |
48 |
99 |
9 |
0.05 |
3 |
65 |
36 |
87 |
10 |
0.05 |
4 |
65 |
24 |
92 |
11 |
0.05 |
5 |
65 |
12 |
95 |
12 |
0.06 |
5 |
65 |
12 |
96 |
13 |
0.07 |
5 |
65 |
12 |
98 |
14 |
0.08 |
5 |
65 |
10 |
97 |
15 |
0.09 |
5 |
65 |
8 |
96 |
16 |
0.10 |
5 |
65 |
7 |
98 |
17 |
0.10 |
0 |
65 |
7 |
58 |
18 |
0 |
5 |
65 |
7 |
43 |
Inspired by the high catalytic efficiency of NUC-56a, epoxides with different substituents were selected to investigate the catalytic universality and size-selectivity under the optimal reaction conditions. As summarized in Table 2, all the selected epoxides with different electronic effects and distinctive steric hindrance were favorably transformed into the corresponding cyclic carbonates with a yield of more than 96%, which were certified by 1H NMR spectroscopy (Fig. S12–19†). Apparently, the epoxides with strong electron-withdrawing groups (–F, –CF3, –Cl, and –C
C) and small steric hindrance were almost entirely converted to the desired products because they could contact the reaction active sites without hindrance and electron-withdrawing groups caused a reduction in the electron density of oxygen atoms, which facilitated the critical step of ring opening (Table 2, entries 1–4 and 6).62,63 For entries 5 and 7, although the product molecules of 2-ethyloxirane and oxiran-2-ylmethanol had a smaller size than the channel widow of NUC-56a, the yield still decreased slightly, which can be attributed to the fact that the substituent groups possessed a notable electron-donating feature.64,65 However, the slightly lower yield of styrene carbonate proved that the microporous windows of NUC-56a had extremely adverse effects on the large-scale transport of large substrate molecules, reflecting that styrene oxide could not be efficiently reactivated in the catalytic sites.66
Table 2 The cycloaddition reactions of CO2 with various epoxides with NUC-56a as the catalysta
The recyclability and heterogeneity of a heterogeneous catalyst are key factors for its practical application in organic synthesis, which prompted us to perform hot leaching and recycling experiments using NUC-56a under the optimum conditions. Primarily, the NUC-56a catalyst involved in the model reaction was removed by centrifugation after 3 h. The observed SC yield of 63.0% did not change significantly as the reaction time was extended, indicating that NUC-56a did not liberate the metal cations and possessed typical heterogeneity (Fig. S23†). Subsequently, the recyclability of NUC-56a was evaluated in ten cycle experiments. After each reaction, NUC-56a was recovered by centrifugation, washed with strongly polar DMF solvent and the volatile acetone solvent, and dried under vacuum at 120 °C for 5 h. Subsequently, the recovered NUC-56a was reused for the next run in the same case. As depicted in Fig. 3, the tenth run gave the yield of SC of 95% with the selectivity remaining over 97%, proving that NUC-56a possessed outstanding catalytic ability and superior recoverability. The PXRD and FT-IR profiles of the recycled catalyst used for 10 times were in accordance with the freshly synthesized catalyst. However, there was no significant change in the N2 adsorption–desorption isotherms, confirming the good stability during the reactions (Fig. S20–S22†). Also, the filtrate obtained after removing the catalyst was tested by ICP-OES (inductively coupled plasma atomic emission spectrometry) technology, which displayed a negligible quantity of In3+ (0.020%) and Tm3+ (0.024%) (Table S5†). Thus, the above-mentioned results suggest that NUC-56a is an extremely stable and highly efficient heterogeneous catalyst in the field of chemical fixation CO2, and has great application prospects.
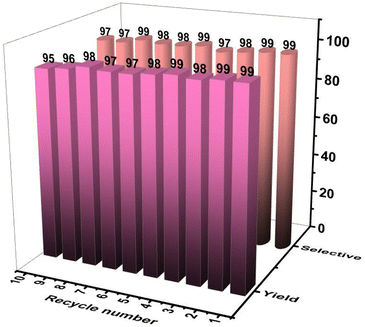 |
| Fig. 3 Recyclability study for catalytic activities of NUC-56a in cycloaddition reaction. | |
Therefore, based on the relevant references combined with the excellent characterization and catalytic performance of NUC-56a, the plausible catalytic mechanism for the cycloaddition is exhibited in Fig. 4.67–71 Firstly, the cycloaddition reaction began with the O atom of epoxide being activated by the Lewis acidic In3+ and Tm3+ sites to form In/Tm-epoxide adducts, resulting in the instability of the ternary rings. Subsequently, the Br− ions released from the co-catalyst of n-Bu4NBr launched an affinity attack on the C atom with less steric hindrance to produce the main intermediates of alkylcarbonate anions, whose O− atoms served as a nucleophilic reagent to react with CO2 in the pores, generating the carbonate ester as the secondary intermediate. Finally, a closed-loop process was performed to end the reaction, accompanied by the release of NUC-56a and Br− to participate in the next reaction process.
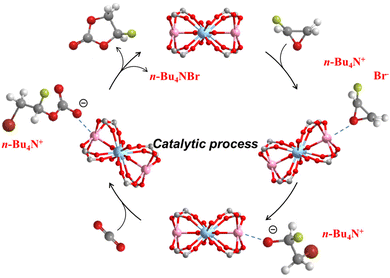 |
| Fig. 4 Proposed reaction mechanism of catalytic cycloaddition reaction. | |
Catalytic activity of NUC-56a on Knoevenagel condensation
Benefiting from the large number of open Lewis acid–base sites of In3+, Tm3+, Npyridine atoms and μ2-OH groups in its nanoporous channels, NUC-56a, as a perfect bifunctional heterogeneous catalyst, could be implemented to catalyze Knoevenagel condensation. As shown in Table 3, in a typical catalytic reaction, benzaldehyde and malononitrile were both introduced in a flask and slowly stirred for 36 h at room temperature under the condition of no NUC-56a. Consequently, the reaction process was continuously monitored by thin layer chromatography (TLC), and only 2% yield was obtained after 36 h, proving that the catalytic reaction hardly occurred the without NUC-56a catalyst, which could be seen as the blank control group for comparison with entry 2. Subsequently, 0.1 mol% NUC-56a was introduced as the catalyst with all the other conditions the same (entry 2). Surprisingly, it was found that the yield of 2-benzylidenemalononitrile in entry 2 (29%) was significantly higher than entry 1, indicating that NUC-56a had a certain promotion effect on the Knoevenagel condensation reaction. In addition, as revealed in entries 2–6, the effect of temperature on the reaction was gradually explored by increments of 10 °C, and the yield reached 99% when the Knoevenagel condensation reaction occurred at 65 °C. Setting 65 °C as the optimal reaction temperature, the influencing factor of the dose of NUC-56a on the reaction was further evaluated. As shown in entries 6–9, when the dose range of NUC-56a increased from 0.1 to 0.4 mol%, the reaction efficiency greatly improved, as evidenced by the high yield obtained in a shorter time. Therefore, the optimal conditions were determined to be 10 mmol benzaldehyde, 20 mmol malononitrile, 0.4 mol% NUC-56a, 65 °C and 6 h. Notably, compared with the reported MOFs used as Knoevenagel condensation catalysts, the reaction catalyzed by NUC-56a exhibited excellent yield and high TON value, which saved more time and conformed to the principle of green chemistry (Table S6†). Additionally, as illustrated in Fig. S24,† the activating energy of the Knoevenagel condensation reaction calculated using Arrhenius equation was 41.4 kJ mol−1.72,73
Table 3 Schematic sketch of Knoevenagel condensation using substrates of benzaldehyde and malononitrilea

|
Entry |
NUC-56a (mol%) |
Time (h) |
T (°C) |
Yieldb (%) |
Reaction conditions: malononitrile (20 mmol), benzaldehyde (10 mmol), ethanol 3 mL.
The internal standard was n-dodecane and checked by GC-MS spectroscopy.
|
1 |
0 |
36 |
25 |
2 |
2 |
0.1 |
36 |
25 |
29 |
3 |
0.1 |
36 |
35 |
47 |
4 |
0.1 |
36 |
45 |
72 |
5 |
0.1 |
36 |
55 |
93 |
6 |
0.1 |
36 |
65 |
99 |
7 |
0.2 |
24 |
65 |
99 |
8 |
0.3 |
12 |
65 |
98 |
9 |
0.4 |
8 |
65 |
99 |
10 |
0.4 |
2 |
65 |
67 |
11 |
0.4 |
4 |
65 |
92 |
12 |
0.4 |
6 |
65 |
98 |
To investigate the effect of different substituents and steric hindrance on the catalytic performance of Knoevenagel condensation, a series of aldehyde derivatives was selected as reactants to carry out the Knoevenagel condensation under the optimal conditions. As shown in entries 2–5 of Table 4, the Knoevenagel condensation reaction of malononitrile with benzaldehyde derivatives substituted with different electron-withdrawing groups (–NO2, –F and –Br) led to 99% yield, indicating that the electron-withdrawing groups may play a beneficial role in the catalytic process. However, the substrates with electron-donating groups of –CH3 had a slightly negative impact on the reaction conversion process, showing a decrease in yield (Table 4, entries 5 and 6). Amazingly, the substrate of 3,4-dimethyl benzaldehyde showed an obviously low yield, which should be attributed to its large molecular size affecting the transport effect of the molecules and the contact opportunity with the reaction sites, as shown in entry 6. All the 1H HMR spectra of the Knoevenagel condensation products are shown in Fig. S25–S30.†
Table 4 The Knoevenagel condensation reaction of aldehyde derivatives containing different groupsa
Moreover, repeatability is an important factor in the practical application of heterogeneous catalysts. After each model reaction of Knoevenagel condensation, NUC-56a was separated by centrifugation, washed with DMSO and acetone, and dried under vacuum. As shown in Fig. 5, the recycled NUC-56a could maintain its catalytic performance with the yield and selectivity of over 95% even after reuse for ten times. Furthermore, the PXRD profile of NUC-56a after ten cycles well matched with the as-synthesized sample, indicating the retention of the initial frame structure during the reaction (Fig. S31†). Alternatively, the FT-IR spectra and the N2 adsorption–desorption isotherms of the recycled samples used for ten cycles are in accordance with the original crystals, further proving the stability of the reused NUC-56a (Fig. S32 and S33†), respectively. Moreover, the supernatant obtained after removing the catalyst was tested by ICP-OES, showing that a negligible quantity of In3+ and Tm3+ ions leaked from NUC-56a (Table S8†). Furthermore, the conducted hot filtration experiments proved that NUC-56a owned typical heterogeneity because the model reaction was close to stagnation after removing the catalyst by filtration after 3 h (Fig. S34†).
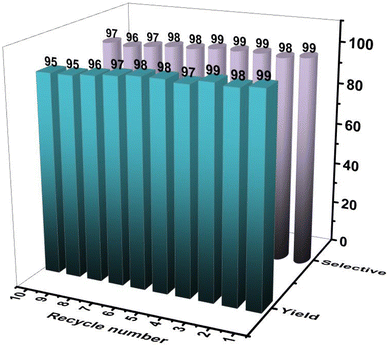 |
| Fig. 5 Recyclability study for catalytic activities of NUC-56a in Knoevenagel condensation reaction. | |
According to the structural features and composition of NUC-56a and the related catalytic systems recorded in the literature, the reaction mechanism of Knoevenagel condensation in the presence of NUC-56a was proposed, as shown in Fig. 6.74–80 Firstly, the active sites of In3+, Tm3+, and μ3-OH ions contact with the O atoms of the aldehyde groups by van der Waals force or hydrogen bonds, weakening the C
O bonds, and then the carbonyl carbon of malononitrile is activated by the N-pyridine atom to form an active imine intermediate. The imine intermediate tends to undergo a Knoevenagel condensation reaction with the weakened C
O bond of aldehyde. Finally, with the release of water molecules, the product of benzylidenemalonitrile is generated.
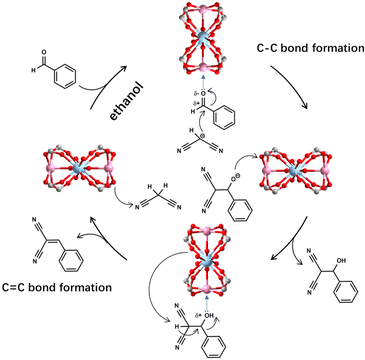 |
| Fig. 6 Proposed reaction mechanism of catalytic Knoevenagel condensation reaction. | |
Conclusions
The solvothermal self-assembly of In3+, Tm3+ and H5BDCP led to the formation of an extremely stable microporous framework of {(Me2NH2)2[In2Tm2(BDCP)2(μ2-OH)2(H2O)2]·2DMF·3H2O}n (NUC-56) possessing an excellent confined pore environment with large specific surface area, high porosity, and abundant co-existing Lewis acid and base sites of In3+, Tm3+, μ2-OH and Npyridine atoms, which are built based on the undocumented heterometallic clusters of [Tm2In2(μ2-OH)2(CO2)10(H2O)2]. Due to the excellent characteristics of NUC-56a, it showed outstanding stability and excellent catalytic activity for the cycloaddition reactions and Knoevenagel condensation reactions under mild chemical conditions. Thus, this work exhibits that the intervention of high-coordinated rare-earth ions in MOF-based catalysts can realize excellent catalysis, stability and regenerative behaviour, meeting the requirements of long-term stability for the industrial application of these materials.
Author contributions
Hongxiao Lv: conceptualization, writing – original draft, methodology, data curation. Hongtai Chen: formal analysis, investigation. Tuoping Hu: performance testing. Xiutang Zhang: supervision, writing – review & editing, project administration.
Conflicts of interest
All authors express that they have no conflict of interest.
Acknowledgements
The authors thank the National Natural Science Foundation (21101097), Basic Research Program of Shanxi Province (202103021224180), Shanxi Key Laboratory of Advanced Carbon Electrode Materials (202104010910019) and the Opening Foundation of Key Laboratory of Laser & Infrared System of Shandong University (2019-LISKFJJ-005) for funding this work.
References
- X. Liu, L. Xie and Y. Wu, Recent Advances in the Shaping of Metal–organic Frameworks, Inorg. Chem. Front., 2020, 7, 2840–2866 RSC.
- S. Bhattacharjee, S. Bera, R. Das, D. Chakraborty, A. Basu, P. Banerjee, S. Ghosh and A. Bhaumik, A Ni(II) Metal–Organic Framework with Mixed Carboxylate and Bipyridine Ligands for Ultrafast and Selective Sensing of Explosives and Photoelectrochemical Hydrogen Evolution, ACS Appl. Mater. Interfaces, 2022 DOI:10.1021/acsami.2c01647.
- H. Xu, K. Ye, K. Zhu, Y. Gao, J. Yin, J. Yan, G. Wang and D. Cao, Hollow Bimetallic Selenide Derived from a Hierarchical MOF-based Prussian Blue Analogue for Urea Electrolysis, Inorg. Chem. Front., 2021, 8, 2788–2797 RSC.
- K. Berijani and A. Morsali, Photocatalytic Materials: Recent Achievements and Near Future Trends, Inorg. Chem. Front., 2021, 8, 3618–3658 RSC.
- S. V. Dummert, H. Saini, M. Z. Hussain, K. Yadava, K. Jayaramulu and A. Casini, Cyclodextrin Metal–organic Frameworks and Derivatives: Recent Developments and Applications, Chem. Soc. Rev., 2022, 51, 5175–5213 RSC.
- H. Xu, K. Ye, K. Zhu, Y. Gao, J. Yin, J. Yan, G. Wang and D. Cao, Template-directed Assembly of Urchin-like CoSx/Co-MOF as an Efficient Bifunctional Electrocatalyst for Overall Water and Urea Electrolysis, Inorg. Chem. Front., 2020, 7, 2602–2610 RSC.
- X. Zou and Y. Zhang, Noble Metal-free Hydrogen Evolution Catalysts for Water Splitting, Chem. Soc. Rev., 2015, 44, 5148–5180 RSC.
- S. Abednatanzi, P. G. Derakhshandeh, H. Depauw, F. X. Coudert, H. Vrielinck, P. V. D. Voort and K. Leus, Mixed-metal Metal-organic Frameworks, Chem. Soc. Rev., 2019, 48, 2535–2565 RSC.
- Y. B. Huang, J. Liang, X. S. Wang and R. Cao, Multifunctional Metal-organic Framework Catalysts: Synergistic Catalysis and Tandem Reactions, Chem. Soc. Rev., 2017, 46, 126–157 RSC.
- L. Chen, H. F. Wang, C. Li and Q. Xu, Bimetallic Metal-organic Frameworks and Their Derivatives, Chem. Sci., 2020, 11, 5369–5403 RSC.
- Y. Zhao, J. Jing, N. Yan, M. L. Han, G. P. Yang and L. F. Ma, Different Benzendicarboxylate-Directed Structural Variations and Properties of Four New Porous Cd(II)-Pyridyl-Triazole Coordination Polymers, Inorg. Chem. Front., 2020, 8, 616468 CAS.
- J. Ma, F. Meng, Y. Yu, D. Liu, J. Yan, Y. Zhang, X. Zhang and Q. Jiang, Prevention of Dendrite Growth and Volume Expansion to Give High-performance Aprotic Bimetallic Li-Na Alloy–O2 Batteries, Nat. Chem., 2019, 11, 64–70 CrossRef CAS PubMed.
- R. Das, S. S. Dhankhar and C. M. Nagaraja, Construction of a Bifunctional Zn(II)-organic Framework Containing a Basic Amine Functionality for Selective Capture and Room Temperature Fixation of CO2, Inorg. Chem. Front., 2020, 7, 72–81 RSC.
- B. Yan, Luminescence Response Mode and Chemical Sensing Mechanism for Lanthanide-functionalized Metal–organic Framework Hybrids, Inorg. Chem. Front., 2021, 8, 201–233 RSC.
- J. Lan, Y. Qu, Z. Wang, P. Xu and J. Sun, A Facile Fabrication of a Multi-functional and Hierarchical Zn-based MOF as an Efficient Catalyst for CO2 Fixation at Room-temperature, Inorg. Chem. Front., 2021, 8, 3085–3095 RSC.
- M. Hu, S. A. A. Razavi, M. Prioozzadeh and A. Morsali, Sensing Organic Analytes by Metal–organic Frameworks: a New Way of Considering the Topic, Inorg. Chem. Front., 2020, 7, 1598–1632 RSC.
- H. Wang, H. Sun, Y. Fu, X. Meng, Y. Zou, Y. He and R. Yang, Varied Proton Conductivity and Photoreduction CO2 Performance of Isostructural Heterometallic Cluster based Metal–organic Frameworks, Inorg. Chem. Front., 2021, 8, 4062–4071 RSC.
- Q. Mou, Z. Xu, G. Wang, E. Li, J. Liu, P. Zhao, X. Liu, H. Li and G. Cheng, A Bimetal Hierarchical Layer Structure MOF Grown on Ni Foam as a Bifunctional Catalyst for the OER and HER, Inorg. Chem. Front., 2021, 8, 2889–2899 RSC.
- M. Ahmed, Recent Advancement in Bimetallic Metal Organic Frameworks (M′MOFs): Synthetic Challenges and Applications, Inorg. Chem. Front., 2022, 9, 3003–3033 RSC.
- S. Liu, H. Chen and X. Zhang, Bifunctional {Pb10K2}–Organic Framework for High Catalytic Activity in Cycloaddition of CO2 with Epoxides and Knoevenagel Condensation, ACS Catal., 2022, 12(16), 10373–10383 CrossRef CAS.
- M. Pander, M. Janeta and W. Bury, Quest for an Efficient 2-in-1 MOF-based Catalytic System for Cycloaddition of CO2 to Epoxides under Mild Conditions, ACS Appl. Mater. Interfaces, 2021, 13, 8344–8352 CrossRef CAS PubMed.
- Z. Gao, L. Liang, X. Zhang, P. Xu and J. Sun, Facile One-pot Synthesis of Zn/Mg-MOF-74 with Unsaturated Coordination Metal Centers for Efficient CO2 Adsorption and Conversion to Cyclic Carbonates, ACS Appl. Mater. Interfaces, 2021, 13, 61334–61345 CrossRef CAS.
- Y. Bai, M. Han, X. Li, S. Feng, L. Lu and S. Ma, Facile and Efficient Photocatalyst for Degradation of Chlortetracycline Promoted by H2O2, Inorg. Chem. Front., 2022, 9, 2952–2963 RSC.
- A. J. Kamphuis, F. Picchioni and P. P. Pescarmona, CO2-fixation into Cyclic and Polymeric Carbonates: Principles and Applications, Green Chem., 2019, 21, 406–448 RSC.
- L. Jiao, J. Zhu, Y. Zhang, W. Yang, S. Zhou, A. Li, C. Xie, X. Zheng, W. Zhou, S.-H. Yu and H.-L. Jiang, Non-Bonding Interaction of Neighboring Fe and Ni Single-Atom Pairs on MOF-Derived N-Doped Carbon for Enhanced CO2 Electroreduction, J. Am. Chem. Soc., 2021, 143, 19417–19424 CrossRef CAS PubMed.
- X. Li, Y. Li, Y. Yang, L. Hou, Y. Wang and Z. Zhu, Efficient Light Hydrocarbon Separation and CO2 Capture and Conversion in a Stable MOF with Oxalamide-decorated Polar Tubes, Chem. Commun., 2017, 53, 12970–12973 RSC.
- T. Zhang, H. Chen, S. Liu, H. Lv, X. Zhang and Q. Li, Highly Robust {Ln4}-organic Frameworks (Ln = Ho, Yb) for Excellent Catalytic Performance on Cycloaddition Reaction of Epoxides with CO2 and Knoevenagel Condensation, ACS Catal., 2021, 11, 14916–14925 CrossRef CAS.
- J. F. Kurisingal, R. Babu, S. Kim, Y. X. Li, J. S. Chang and S. J. Cho, Microwave-induced Synthesis of a Bimetallic Charge-transfer Metal Organic Framework: a Promising Host for the Chemical Fixation of CO2 via Cyclic Carbonate Synthesis, Catal. Sci. Technol., 2018, 8, 591–600 RSC.
- V. Aomchad, A. Cristofol, F. D. Monica, B. Limburg, V. D'Elia and A. W. Kleij, Recent Progress in the Catalytic Transformation of Carbon Dioxide into Biosourced Organic Carbonates, Green Chem., 2021, 23, 1077–1113 RSC.
- C. C. Hou, H. F. Wang, C. Li and Q. Xu, From Metal-organic Frameworks to Single/dual-atom and Cluster Metal Catalysts for Energy Applications, Energy Environ. Sci., 2020, 13, 1658–1693 RSC.
- S. Liu, H. Chen, H. Lv, Q.-P. Qin, L. Fan and X. Zhang, Chemorobust 4p-5p {InPb}-organic framework for efficiently catalyzing cycloaddition of CO2 with epoxides and deacetalization-Knoevenagel condensation, Mater. Today Chem., 2022, 24, 100984 CrossRef CAS.
- C. C. Hou, H. F. Wang, C. Li and Q. Xu, From Metal-organic Frameworks to Single/dual-atom and Cluster
Metal Catalysts for Energy Applications, Energy Environ. Sci., 2020, 13, 1658–1693 RSC.
- B. Parmar, P. Patel, R. S. Pillai, R. K. Tak, R. I. Kureshy, N. H. Khan and E. Suresh, Cycloaddition of CO2 with an Epoxide Bearing Oxindole Scaffold by a Metal-Organic Framework-Based Heterogeneous Catalyst under Ambient Conditions, Inorg. Chem., 2019, 58, 10084–10096 CrossRef CAS PubMed.
- S. Zhang, F. Ou, S. Ning and P. Cheng, Polyoxometalate-based Metal-organic Frameworks for Heterogeneous Catalysis, Inorg. Chem. Front., 2021, 8, 1865–1899 RSC.
- J. Lan, Y. Qu, X. Zhang, H. Ma, P. Xu and J. Sun, A Novel Water-stable MOF Zn(Py)(Atz) as Heterogeneous Catalyst for Chemical Conversion of CO2 with Various Epoxides under Mild Conditions, J. CO2 Util., 2020, 35, 216–224 CrossRef CAS.
- A. K. Ghosh, U. Saha, S. Biswas, Z. A. Alothman, M. A. Islam and M. Dolai, Anthracene-triazole-dicarboxylate-based Zn(II) 2D Metal Organic Frameworks for Efficient Catalytic Carbon Dioxide Fixation into Cyclic Carbonates under Solvent-free Condition and Theoretical Study for the Reaction Mechanism, Ind. Eng. Chem. Res., 2022, 61, 175–186 CrossRef CAS.
- S. S. Dhankhar and C. M. Nagaraja, Construction of a 3D Porous Co(II) Metal-organic Framework (MOF) with Lewis Acidic Metal Sites Exhibiting Selective CO2 Capture and Conversion under Mild Conditions, New J. Chem., 2019, 43, 2163–2170 RSC.
- J. Gu, X. Sun, X. Liu, Y. Yuan, H. Shan and Y. Liu, Highly Efficient Synergistic CO2 Conversion with Epoxide Using Copper Polyhedron-based MOFs with Lewis acid and Base sites, Inorg. Chem. Front., 2020, 7, 4517–4526 RSC.
- R. Das, V. Parihar and C. M. Nagaraja, Strategic Design of a Bifunctional Ag(I)-grafted NHC-MOF for Efficient Chemical Fixation of CO2 from a Dilute Gas under Ambient Conditions, Inorg. Chem. Front., 2022, 9, 2583–2593 RSC.
- R. Das, T. Ezhil, A. S. Palakkal, D. Muthukumar, R. S. Pillai and C. M. Nagaraja, Efficient Chemical Fixation of CO2 from Direct Air under Environment-friendly Co-catalyst and Solvent-free Ambient Conditions, J. Mater. Chem. A, 2021, 9, 23127–23139 RSC.
- U. Patel, P. Patel, B. Parmar, A. Dadhania and E. Suresh, Synergy of Dual Functional Sites for Conversion of CO2 in a Cycloaddition Reaction under Solvent-Free Conditions by a Zn(II)-Based Coordination Network with a Ladder Motif, Cryst. Growth Des., 2021, 21, 1833–1842 CrossRef CAS.
- X.-R. Tian, Y. Shi, S.-L. Hou, Y. Ma and B. Zhao, Efficient Cycloaddition of CO2 and Aziridines Activated by a Quadruple-Interpenetrated Indium–Organic Framework as a Recyclable Catalyst, Inorg. Chem., 2021, 60, 15383–15389 CrossRef CAS PubMed.
- S. Chong, D. T. Park and J. Kim, Exploring Guest-Dependent Photoconductivity in a Donor-containing Metal–organic Framework, J. Phys. Chem. C, 2021, 125, 10198–10206 CrossRef CAS.
- S. S. Wang, H. H. Huang, M. Liu, S. Yao, S. Guo and J. W. Wang, Encapsulation of Single Iron Sites in a Metal–porphyrin Framework for High-performance Photocatalytic CO2 reduction, Inorg. Chem., 2020, 59, 6301–6307 CrossRef CAS PubMed.
- Y. H. Li, S. L. Wang, Y. C. Su, K. Bao-Tsan, T. Chen-Yen and C. H. Lin, Microporous 2D Indium Metal-organic Frameworks for Selective CO2 Capture and their Application in the Catalytic CO2-cycloaddition of Epoxides, Dalton Trans., 2018, 47, 9474–9481 RSC.
- A. Kong, Q. Lin, C. Mao, X. Bu and P. Feng, Efficient Oxygen Reduction by Nanocomposites of Heterometallic Carbide and Nitrogen-Enriched Carbon Derived from the Cobalt-encapsulated Indium–MOF, Chem. Commun., 2014, 50, 15619–15622 RSC.
- Y. Sun, B. Xia, S. Ding, L. Yu, S. Chen and J. Duan, Rigid Two-Dimensional Indium Metal–organic Frameworks Boosting Nitrogen Electroreduction at all pH Values, J. Mater. Chem. A, 2021, 9, 20040–20047 RSC.
- G. Verma, K. Forrest, B. A. Carr, H. Vardhan and S. Ma, Indium–organic Framework with soc Topology as a Versatile Catalyst for Highly Efficient One-pot Strecker Synthesis of α-aminonitriles, ACS Appl. Mater. Interfaces, 2021, 13, 52023–52033 CrossRef CAS.
- C. Lu, D. Xiong, C. Chen, J. Wang, Y. Kong, T. Liu, S. Ying and F. Yi, Indium-based Metal–organic Framework for Efficient Photocatalytic Hydrogen Evolution, Inorg. Chem., 2022, 61, 2587–2594 CrossRef CAS.
- L. Zhai, J. W. Yu, J. Zhang, W. W. Zhang, L. Wang and X. M. Ren, High Quantum Yield Pure Blue Emission and Fast Proton Conduction from an Indium–metal–organic Framework, Dalton Trans., 2019, 48, 12088–12095 RSC.
- Y. Zhang, S. Liu, Z. S. Zhao, Z. Wang, R. Zhang and L. Liu, Recent Progress in Lanthanide Metal–organic Frameworks and Their Derivatives in Catalytic Applications, Inorg. Chem. Front., 2021, 8, 590–619 RSC.
- M. Tian, J. Zheng, J. Xue, X. Pan, D. Zhou and Q. Yao, A Series of Microporous and Robust Ln-mofs Showing Luminescence Properties and Catalytic Performances towards Knoevenagel Reactions, Dalton Trans., 2021, 50, 17785–17791 RSC.
- H. Chen, Z. Zhang, T. Hu and X. Zhang, An NH2-modified {EuIII2}–Organic Framework for the Efficient Chemical Fixation of CO2 and Highly Selective Sensing of 2,4,6-Trinitrophenol, Inorg. Chem. Front., 2021, 8, 4376–4385 RSC.
- R. Babu, R. Roshan, A. C. Kathalikkattil, D. Kim and D. W. Park, Rapid, Microwave-assisted Synthesis of Cubic, Three-dimensional, Highly Porousmof-205 for Room Temperature CO2 Fixation via Cyclic Carbonatesynthesis, ACS Appl. Mater. Interfaces, 2016, 8, 33723–33731 CrossRef CAS PubMed.
- P. C. Purba, M. Venkateswaralu, S. Bhattacharyya and P. S. Mukherjee, Silver(I)–Carbene Bond-Directed Rigidification-Induced Emissive Metallacage for Picric Acid Detection, Inorg. Chem., 2022, 61, 713–722 CrossRef CAS PubMed.
- Y. B. N. Tran, P. T. K. Nguyen, Q. T. Luong and K. D. Nguyen, Series of M-MOF-184 (M=Mg, Co, Ni, Zn, Cu, Fe) Metal–Organic Frameworks for Catalysis Cycloaddition of CO2, Inorg. Chem., 2020, 59, 16747–16759 CrossRef CAS PubMed.
- G. Mercuri, M. Moroni, S. Galli, G. Tuci, G. Giambastiani, T. Yan, D. Liu and A. Rossin, Temperature-dependent Nitrous Oxide/Carbon Dioxide Preferential Adsorption in a Thiazolium-functionalized NU-1000 Metal–organic Framework, ACS Appl. Mater. Interfaces, 2021, 13, 58982–58993 CrossRef CAS PubMed.
- W. L. Xue, L. Wang, Y. K. Li, H. Chen and C. Q. Wan, Reticular Chemistry for Ionic Liquid-functionalized Metal–organic Frameworks with High Selectivity for CO2, ACS Sustainable Chem. Eng., 2020, 8, 18558–18567 CrossRef CAS.
- Z. Bian, Y. Zhang, D. Tian, X. Zhang, L. Xie, M. Zhao, Y. Xie and J. Li, Co7-Cluster-Based Metal-organic Frameworks with Mixed Carboxylate and Pyrazolate Ligands: Construction and CO2 Adsorption and Fixation, Cryst. Growth Des., 2020, 20, 7972–7978 CrossRef CAS.
- X. Chen, E. Yu, S. Cai, H. Jia, J. Chen and P. Liang, In Situ Pyrolysis of Ce-MOF to Prepare CeO2 Catalyst with Obviously Improved Catalytic Performance for Toluene Combustion, Chem. Eng. J., 2018, 344, 469–479 CrossRef CAS.
- L. Yang, H. Zhang, P. Tao, X. Lu and Q. Xia, Microwave-assisted Air Epoxidation of Mixed Biolefins over a Spherical Bimetal ZnCo-MOF Catalyst, ACS Appl. Mater. Interfaces, 2021, 13, 8474–8487 CrossRef CAS PubMed.
- R. Cao, Z. Chen, Y. Chen, K. B. Idrees, S. L. Hanna, X. Wang, T. A. Goetjen, Q. Sun, T. Islamoglu and O. K. Farha, Benign Integration of a Zn-azolate Metal-organic Framework onto Textile Fiber for Ammonia Capture, ACS Appl. Mater. Interfaces, 2020, 12, 47747–47753 CrossRef CAS PubMed.
- D. Chen, W. Yang, L. Jiao, L. Li, S.-H. Yu and H.-L. Jiang, Boosting Catalysis of Pd Nanoparticles in MOFs by Pore Wall Engineering: the Roles of Electron Transfer and Adsorption Energy, Adv. Mater., 2020, 32, 2000041 CrossRef CAS PubMed.
- J. Lan, Y. Qu, Z. Wang, P. Xu and J. Sun, A Facile Fabrication of a Multi-functional and Hierarchical Zn-based MOF as an Efficient Catalyst for CO2 Fixation at Room-temperature, Inorg. Chem. Front., 2021, 8, 3085–3095 RSC.
- Y. Guo, B. Gao, Z. Deng, Y. Liu, X. Peng and Y. Zhao, Charge Separation in Hybrid Metal-organic Framework Films for Enhanced Catalytic CO2 conversion, J. Mater. Chem. A, 2021, 9, 2694–2699 RSC.
- H. Lv, Z. Zhang, L. Fan, Y. Gao and X. Zhang, A Nanocaged Cadmium–organic Framework with High Catalytic Activity on the Chemical Fixation of CO2 and Deacetalization-knoevenagel Condensation, Microporous Mesoporous Mater., 2022, 51, 111791 CrossRef.
- H. Chen, T. Zhang, S. Liu, H. Lv, L. Fan and X. Zhang, Fluorine-Functionalized NbO-Type {Cu2}-Organic Framework: Enhanced Catalytic Performance on the Cycloaddition Reaction of CO2 with Epoxides and Deacetalization-Knoevenagel Condensation, Inorg. Chem., 2022, 61, 11949–11958 CrossRef CAS.
- T. Stolar, A. Pranikar, V. Martinez, B. Karadeniz and K. Uarevi, Scalable Mechanochemical Amorphization of Bimetallic Cu-Zn MOF-74 Catalyst for Selective CO2 Reduction Reaction to Methanol, ACS Appl. Mater. Interfaces, 2021, 13, 3070–3077 CrossRef CAS.
- F. Ma, F. Mi, M. Sun, T. Huang, Z. Wang, T. Zhang and R. Cao, A Highly Stable Zn9-pyrazolate Metal-organic Framework with Metallosalen Ligands as a Carbon Dioxide Cycloaddition Catalyst, Inorg. Chem. Front., 2022, 9, 1812–1818 RSC.
- A. Liu, Y. Chen, P. Liu, W. Qi and B. Li, Conveision of CO2 to Epoxide or Oxazolidinone Enable by a CuI/CuII -organic Frameworks Bearing a tri-functional linker, Inorg. Chem. Front., 2022, 9, 4425–4432 RSC.
- M. Pander, M. Janeta and W. Bury, Quest for an Efficient 2-in-1 MOF-based Catalytic System for Cycloaddition of CO2 to Epoxides under Mild Conditions, ACS Appl. Mater. Interfaces, 2021, 13, 8344–8352 CrossRef CAS.
- Q. Niu, Y. Wang, G. Li, Y. Zhao, V. Singh, J. Niu and J. Wang, Polyoxoniobates as a Superior Lewis base Efficiently Catalyzed Knoevenagel Condensation, Mol. Catal., 2018, 453, 93–99 CrossRef.
- Y. Ren, H. Li, W. Yang, D. Shi, Q. Wu, Y. Zhao, C. Feng, H. Liu and Q. Jiao, Alkaline Ionic Liquids Immobilized on Protective Copolymers Coated Magnetic Nanoparticles: an Efficient and Magnetically Recyclable Catalyst for Knoevenagel Condensation, Ind. Eng. Chem. Res., 2019, 58, 2824–2834 CrossRef CAS.
- A. Das and K. Thomas, Rose Bengal Photocatalyzed Knoevenagel Condensation of Aldehydes and Ketones in Aqueous Medium, Green Chem., 2022, 24, 4952–4957 RSC.
- H. Lv, L. Fan, H. Chen, X. Zhang and Y. Gao, Nanochannel-based {BaZn}–organic Framework for Catalytic Activity on the Cycloaddition Reaction of Epoxides with CO2 and Deacetalization-knoevenagel condensation, Dalton Trans., 2022, 51, 3546–3556 RSC.
- F. Ghobakhloo, D. Azarifar, M. Mohammadi, H. Keypour and H. Zeynail, Copper(II) Schiff-Base Complex Modified UiO-66-NH2(Zr) Metal–Organic Framework Catalysts for Knoevenagel Condensation–Michael Addition–Cyclization Reactions, Inorg. Chem., 2022, 61, 4825–4841 CrossRef CAS PubMed.
- J. N. Appaturi, R. Ratti, B. L. Phoon, S. M. Batagarawa, I. Dia, M. Selvaraj and R. J. Ramalingam, Mixed-Linker Isoreticular Zn(II) Metal–Organic Frameworks as Brønsted Acid–Base Bifunctional Catalysts for Knoevenagel Condensation Reactions, Dalton Trans., 2022, 51, 2567–2576 RSC.
- S. Johari, M. R. Johan and N. G. Khaligh, An Overview of Metal-Free Sustainable Nitrogen-based Catalytic Knoevenagel Condensation Reaction, Org. Biomol. Chem., 2022, 20, 2164–2186 RSC.
- J. Qiao, B. Zhang, L. Zhang and Y. Liu, Practice of Function-oriented Synthesis: Efficient CO2 Conversion and Knoevenagel Condensation by Two New In3 based MOFs with High-density Active Sites under Mild Conditions, J. Mater. Chem. A, 2022, 10, 17773–17781 RSC.
- A. Karmakar, M. M. A. Soliman, G. M. D. M. Rubio, M. F. C. Silva and A. J. L. Pombeiro, Synthesis and Catalytic Activities of a Zn(II) Based Metallomacrocycle and a Metal–organic Framework towards One-pot Deacetalization-knoevenagel Tandem Reactions under Different Strategies: a Comparative Study, Dalton Trans., 2020, 49, 8075–8085 RSC.
|
This journal is © the Partner Organisations 2022 |