DOI:
10.1039/D2QI00407K
(Research Article)
Inorg. Chem. Front., 2022,
9, 2997-3002
Sequential enhancement of proton conductivity by aliovalent cadmium substitution and post-synthetic esterolysis in a carboxylate-functionalized indium framework with dimethylaminium templates†
Received
22nd February 2022
, Accepted 29th April 2022
First published on 30th April 2022
Abstract
A sequential improvement strategy has been devised and implemented on a 3D open framework In-BQ showing 2D intersected channels filled by dimethylamine and its protonated cation constructed by –COOCH3–functionalized anilicate linkers. In situ aliovalent metal substitution and post-synthetic ligand esterolysis led to Cd-BQ-COOH with a doubling of Me2NH2+ carriers and a great number of residual –COOH groups, resulting in maximum proton concentration and frequent jumping sites. As a result, the modified Cd-BQ-COOH exhibits a 300-fold enhanced value of proton conductivity compared with that of pristine In-BQ, reaching 6.06 × 10−2 S cm−1. MD calculations reveal that the entire process of proton transportation in Cd-BQ-COOH is achieved by the vehicle mechanism.
Introduction
Solid-state proton conductors have attracted considerable attention for a wide variety of applications in hydrogen separation, water electrolysis, biological sensors and fuel cells,1,2 which are currently focused on dramatically improving their proton conductivity and deeply illuminating their intrinsic conducting pathways.3,4 Compared with other inorganic metal oxides or organic polymers,5,6 metal–organic frameworks (MOFs) are studied and accepted as preferred candidates for next-generation conducting materials due to their ordered crystalline nature, high internal porosity and tunable modular functionality.7–10 These unique characteristics are beneficial not only to acquire rich proton sources in a restricted volume to increase proton carrier concentration but also to create abundant hopping sites in a specific alignment to elevate the proton mobility.11,12 Although most MOF materials show good prospects, it is still required to tactically fine-tune key structural components towards the precise design of high-level performance and long-term durability of proton conduction.13–15
For this purpose, two distinct strategies involving predesigned methods or post-synthetic modifications16–19 have been proposed and implemented to draw multiple proton carriers into MOFs: (1) the introduction of protophilic groups (–SO3H, –PO3H2, –COOH, –OH, etc.) on the backbones by retaining residual acidic groups or transforming the precursors into functional groups;20,21 (2) the incorporation of protic entities (H2SO4, H3PO4, imidazole, triazole, ammonium cations, etc.) into the channels by balancing charged frameworks or exchanging guest molecules.22,23 Particularly, there have been some recent synergistic highly proton-conducting materials originating from the aforementioned approaches, for example H2SO4@MIL-101-SO3H,24 BUT-8(Cr)A,25 and PCMOF2½(Tz).26 These reasonable and sequential modifications of the products afford maximum proton donor–acceptor and strong host–guest interactions to establish successive hydrogen-bond networks and efficient proton-transfer pathways, leading to conductivity values surpassing 10−1 S cm−1 below 373 K with humidification, which are comparable to that of commercial Nafion materials.27–29
Inspired by the feasibility of the above tactics, we attempt to explore enhancing strategies with synergistic effects to optimize proton conduction performance. In a previous report, we proposed an aliovalent metal substitution strategy30,31 and implemented it on a dimethylaminium-templated compound In-BQ.31 This diamond-topology open framework featuring 2D intersected channels filled with dimethylamine and its protonated dimethylaminium constructed by –COOCH3-functionalized anilicate linkers prompted us to sequentially modify the cooperation of aliovalent Cd(II) substitution and post-synthetic ligand esterolysis. Surprisingly, the modified framework Cd-BQ-COOH has three kinds of proton sources: the first are the attached –OH groups, which could dissociate H+ during the coordinate progress; the second are the filled Me2NH molecule and Me2NH2+ cation, which could protonate into the doubling of Me2NH2+ cations through the aliovalent replacement of In(III) by Cd(II); and the third are the functionalized –COOH groups, which are potentially converted from the post-synthetic esterolysis of –COOCH3 on anilicate ligands. In contrast to the Cd(II)-substituted Cd-BQ and carboxyl-functionalized In-BQ-COOH, which have already boosted the proton conductivities by 100-fold (2.30 × 10−2 S cm−1, 303 K and 95% RH) and 15-fold (3.54 × 10−3 S cm−1, 303 K and 95% RH), respectively, when compared with pristine In-BQ, the sequentially modified Cd-BQ-COOH exhibits a 300-fold enhancement with up to 6.06 × 10−2 S cm−1 at the same condition.
Experimental section
Chemicals and materials
The starting materials and solvents were purchased and utilized from commercial sources without further purification. In-BQ and Cd-BQ were prepared according to our previously described procedure.31 To maintain the integrity of the skeletons and reduce the possibility of losing molecules/cations in the pores as much as possible, a mild and reversible acid hydrolysis was employed. In-BQ (0.2 g) and Cd-BQ (0.2 g) were separately hydrolyzed with 1 M H2SO4 solution (20 mL) in a 50 mL round-bottom flask. The suspensions of the solid were stirred for 10 h at 40 °C. After cooling to about 30 °C, the resulting powdered solids were isolated by centrifugation and washed with H2O several times until the upper fluid was neutral. The morphological changes in both materials before and after esterolysis are shown in Fig. S1.†
Physical characterization
Scanning electron microscopy (SEM) images were recorded using a Thermofisher APR 20 emission scanning electron microscope with an accelerating voltage of 2–10 kV. Powder X-ray diffraction (PXRD) was measured on a Rigaku D/max-2550 diffractometer using Cu Kα radiation with an angle range (2θ) from 5° to 50°. Fourier transform infrared spectra (FT-IR) were obtained on KBr pellets with a PERKIN-ELMER 100-IR spectrometer (400–4000 cm−1) at 298 K. Thermal gravimetric analysis (TGA) curves were collected using a JUPITER STA 449F3 instrument in an air atmosphere with a heating rate of 10 °C min−1. Nuclear magnetic resonance (NMR) data were analysed using a BRUKER AVANCE 400 spectrometer. Electrospray ionization-mass spectrometry (ESI-MS) was performed on a BRUKER SolanX 70 FT-MS. The H2O vapor adsorption isotherms were recorded using a BSD-VVS gravimetric vacuum steam adsorber at room temperature.
Proton conductivity measurement
The proton conductivity was tested using a Princeton ParStat-4000 electrochemical analyzer with a two-probe system at an AC voltage of 100 mV in the frequency range of 1 MHz to 0.1 Hz. The as-synthesized samples were pressed into pellets of 6.0 mm diameter and 1–2 mm thickness at a pressure of 20 MPa. The measured slice pellet was sandwiched between two stainless steel wafers and clamped with an electrode clip. The system temperature and humidity were controlled by a BLUEPARD BPS-50L programmable incubator. The proton conductivity (σ) was calculated according to eqn (1), where σ = proton conductivity (S cm−1), L = the thickness (cm), A = surface area (cm2), and R = impedance (Ω). |  | (1) |
Ea is the activation energy, which was determined from the fitted slope of the Arrhenius eqn (2). | 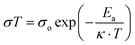 | (2) |
Computational details
The initial and fully hydrolyzed In-BQ-COOH and Cd-BQ-COOH structures were built and optimized on the basis of the In-BQ and Cd-BQ crystal structures, respectively. Subsequently, a computational unit involving a couple of Me2NH2+ (or one Me2NH2+ and one Me2NH) and associated framework molecules was chosen as a theoretical model. The positions of the O atoms and C atoms of the framework were highly fixed to avoid crumbling or large structural distribution. Meanwhile, all atoms of H and the dimethylaminium templates remained free, allowing proton carriers with H+ to perform transportation or exchange. The entire model was fixed in a simulation box with a vacuum area of 10 Å3. The quantum molecular dynamics (MD) were simulated using the SIESTA package32 with the GGA-PBE functional33 and Troullier–Martins norm-conserving pseudopotentials.34 A double-ζ polarized (DZP) basis set was employed for the valence electronic orbitals of all atoms and the mesh cut-off was set as 250 Ry. The Monkhorst–Pack type of k-point sampling with a (1 × 1 × 1) mesh was used for the In-BQ-COOH and Cd-BQ-COOH structure models. The MD simulation was controlled by the Nosé35 method employing 2000 steps at a time step of 1 fs at 298 K.
Results and discussion
In the host backbone of In-BQ, each In(III) ion is eight-coordinated with O atoms belonging to four anilicate ligands in the chelate mode, and each linker is connected to two In(III) ions in a bis-bidentate fashion, building a 3D diamond-topology structure containing 2D intersected channels. The channels are decorated by coordinated –OH groups and residual –COOCH3 groups, as well as filled with a Me2NH molecule and a Me2NH2+ counterion. Accompanied with the in situ aliovalent replacement of In(III) by Cd(II), the original Me2NH/Me2NH2+ in In-BQ protonate into the doubling of Me2NH2+ cations in Cd-BQ for charge balance. Moreover, the absolute freedom of the –COOCH3 groups on the anilicate linkers offers an uncommon opportunity for the –COOH ligand functionalization to confer this material with advanced proton conduction performance. Through the post-synthetic esterolysis of In-BQ and Cd-BQ by H2SO4 (1 M) aqueous solution, the modified frameworks have been generated, denoted as Cd-BQ-COOH and In-BQ-COOH, respectively (Fig. 1).
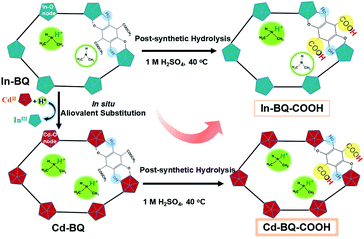 |
| Fig. 1 The formation processes for In-BQ-COOH and Cd-BQ-COOH. | |
Notably, the hydrogen bonds in Cd-BQ-COOH [N–H⋯O (2.86–3.10 Å) and O–H⋯O (2.61–3.69 Å)] are much shorter than those in In-BQ-COOH [N–H⋯O (2.97–3.65 Å) and O–H⋯O (2.71 Å)] (Table S1† and Fig. 2), and are much richer than those in Cd-BQ and In-BQ as well (Fig. S2†). Apparently, the multi-step route of Cd(II) substitution and –COOH functionalized modification gives rise to an expanded hydrogen-bond network, and endows Cd-BQ-COOH with progressively boosted proton conductivity.
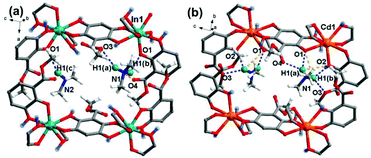 |
| Fig. 2 Comparison of the H-bond arrangements of (a) In-BQ-COOH and (b) Cd-BQ-COOH. Note: The H atoms of –OH, –COOH, and Me2NH/Me2NH2+ are highlighted in blue, yellow, and green, respectively. The blue and orange dashed lines denote the N–H⋯O and O–H⋯O bonds, respectively. | |
The PXRD profiles before and after the proton-conducting tests of In-BQ-COOH and Cd-BQ-COOH are matched well with the simulated and as-synthesized patterns of the In-BQ and Cd-BQ frameworks, respectively, demonstrating the existence of the crystalline structures after the entire post-synthetic treatments and impedance measurements (Fig. S3†). Additionally, the –COOH functionalization frameworks were confirmed by FT-IR (Fig. S4†). The absorption peaks of the –COOCH3 group located at 1720 and 1150 cm−1 in In-BQ and 1730 and 1300 cm−1 in Cd-BQ, respectively, are weakened in the spectra of In-BQ-COOH and Cd-BQ-COOH, and several peaks appear near 1710 and 900 cm−1 stemming from the C
O and O–H stretching vibrations, respectively. To further confirm the production of the –COOH group, we performed ESI-MS and NMR analyses (Fig. S5 and S6†). The ESI-MS spectrum after hydrolysis shows high intensity peaks at m/z values of 227.45 and 256.24, while the spectrum before hydrolysis only shows one intensity peak at 256.77. The new peak position fits well with the mass of the expected carboxylic fragment. In the 1H-NMR spectra, where dibromide was used as a reference, the integral area of –CH3 was obviously diminished after hydrolysis, indirectly demonstrating the formation of –COOH. Both frameworks after hydrolysis show almost identical proportion of losing weight from In-BQ and Cd-BQ at the first step in the range of 30–150 °C, suggesting that the guest molecules are retained without much damage (Fig. S7†). The different hydrophilicity of the inner pores has been characterized by H2O vapor adsorption at 298 K (Fig. S8†). The H2O vapor uptakes of In-BQ-COOH and Cd-BQ-COOH are 140 wt% and 102 wt%, respectively, much higher than the 58 wt% of In-BQ and 38 wt% of Cd-BQ.31 This typical increase is associated with the effective transition of hydrophobic groups into hydrophilic groups hanging in the inner pore wall.
The proton-conductivities of In-BQ-COOH and Cd-BQ-COOH were measured by performing electrochemical impedance spectroscopy (EIS) on the pelletized powder samples. All resistance values were estimated from the Z′ intercept values due to the imperfect semicircle of the Nyquist plots.22,36 In order to evaluate the water affinities of these two compounds, under similar testing conditions to those of In-BQ and Cd-BQ, humidity-dependent proton conducting data were measured at 25 °C with RH increasing from 55% to 95% (Table S2 and Fig. S9†). At 55% RH, the conductive value of Cd-BQ-COOH is 4.85 × 10−6 S cm−1, compared with the 8.27 × 10−6 S cm−1 of In-BQ-COOH. With rising humidity, the proton conductivity of Cd-BQ-COOH exceeds that of In-BQ-COOH and exhibits an ultrahigh conductive value of 3.03 × 10−2 S cm−1 at 95% RH, which is nearly 20 times higher than that of In-BQ-COOH (1.57 × 10−3 S cm−1) at the same humidity. This increasing trend is consistent with the humidity-dependent results of In-BQ and Cd-BQ, and the proton conductivities are all improved under the parallel comparison. This is mainly attributed to the hydrophilicity of the –COOH groups, where absorbed water molecules acting as proton carriers could increase the efficiency of proton transfer.
To further investigate the potential conducting mechanism, the temperature-dependent proton conductivity, which is a key factor for the calculation of the resulting activation energy (Ea), was tested. Analogous to Cd-BQ and In-BQ, both Cd-BQ-COOH and In-BQ-COOH show better conductive behaviors in the low temperature region,12,37,38 and thus the temperature-dependent conductive ability of these two compounds was characterized from 10 to 30 °C under 95% RH (Fig. 3, Fig. S10 and Table S3†). At 10 °C, the conducting values of Cd-BQ-COOH and In-BQ-COOH are 2.01 × 10−2 S cm−1 and 8.75 × 10−4 S cm−1, respectively. As the temperature increases to 30 °C, the conductivities increase and reach their maximum values of 6.06 × 10−2 S cm−1 and 3.54 × 10−3 S cm−1, respectively. The conductivities of Cd-BQ-COOH and In-BQ-COOH are also compared with other reported MOF-based proton conductors by ligand post-synthetic modification, as shown in Table S4.† Obviously, the 20-fold increased conductivities of the two compounds in the same range are almost maintained. As a comparison, the Ea values of Cd-BQ-COOH and In-BQ-COOH are determined using the Arrhenius equation and found to be 0.41 eV and 0.56 eV, respectively, much lower than the 0.48 eV of Cd-BQ and 0.73 eV of In-BQ.31 This result suggests that the energy consumption of the proton transportation in both compounds decreases with the participation of the –COOH groups, and the intrinsic proton migration may be due to the vehicle mechanism.
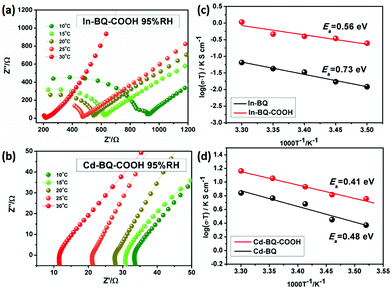 |
| Fig. 3 Impedance spectra of (a) In-BQ-COOH and (b) Cd-BQ-COOH at 10–30 °C under 95% RH. Comparison of the Arrhenius plots of (c) In-BQ-COOH and (d) Cd-BQ-COOH with In-BQ and Cd-BQ, respectively, at 10–30 °C under 95% RH. | |
MD calculation has been recognized as a promising method to clearly elucidate the proton transportation mechanism at the atomic scale.39–43 Based on this, further simulation studies on Cd-BQ-COOH and In-BQ-COOH were performed by first-principles calculations with an assumption that all the ester groups in Cd-BQ and In-BQ were completely replaced by –COOH groups.
At the initial situation in Cd-BQ-COOH (Fig. 4a), the Me2NH2+ cation mainly spins and glides around the Cd1(a) atom through the breakage and reestablishment of H-bonds. Then, at ∼500 fs (Fig. 4b ), the H1(a) and H1(b) atoms on the Me2NH2+ cation keep on moving towards the central region of the channel, building almost equal lengths of H-bonds with O1(a) and O1(b). Accompanied by the continuous movement of the Me2NH2+ cation at 650 fs, the H-bonds between H1(a) and H1(b) with O1(b) and O2(b) are re-established, as shown in Fig. 4c, which lead to the protons transferring near Cd1(b). In the final process (Fig. 4d), the Me2NH2+ cation migrates and returns near Cd1(a) by means of the H-bonds established with O2(a) and O3(a). It can be clearly seen that in the whole progress of proton transmission, the Me2NH2+ cation acts as a whole to undergo self-rotation and migration in the counterclockwise direction of “Cd1(a)–Cd1(b)–Cd1(a)”.
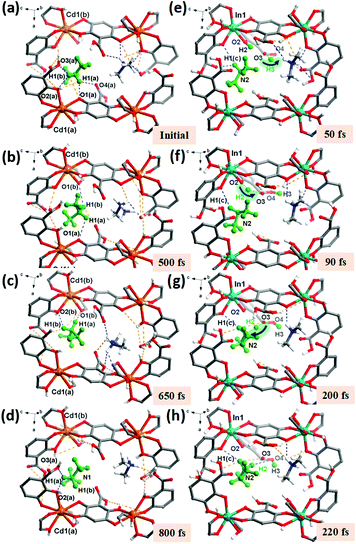 |
| Fig. 4 Migration trajectory of Me2NH2+ in Cd-BQ-COOH at (a) the initial state; (b and c) the intermediate states; and (d) the later state. (e and f) The pathway of proton hopping between the –OH group and –COOH group in In-BQ-COOH. (g) A strong interaction is established between the H+ and Me2NH. (h) Formation of a protonated Me2NH2+. Note: The Me2NH/Me2NH2+ and the hopping protons are highlighted in green. The blue and orange dashed lines illustrate the changes of N–H⋯O and O–H⋯O bonds, respectively. | |
Besides, in In-BQ-COOH, an interesting consecutive proton reorientation occurs on the Me2NH molecule and finally protonates the Me2NH2+ cation. Fig. 4e represents the situation at 50 fs, where the H3 atom on the –COOH group jumps from the O3 atom to the O4 atom following the intramolecular H-bond. Subsequently at ∼90 fs, the O3 atom acting as a hopping site accepts the H2 atom coordinated with the O2 atom belonging to –OH moieties (Fig. 4f). This intermediate stage of the H2 proton on O3 is estimated to be ∼100 fs. Then with the self-rotation and vibration of the Me2NH molecule, the H2 atom jumps from the O3 atom to the N2 atom at 200 fs, which corresponds to an O⋯N distance of 2.58 Å and an O3–H2⋯N2 angle of 162(08)° (Fig. 4g). In the later process, the protonated Me2NH2+ cation as a whole continues to migrate (Fig. 4h). Overall, the entire process of proton migration involves the moving sequence of “O2–O3–N2”, which is jointly achieved by the hopping mechanism and the vehicle mechanism.
Conclusions
In summary, by sequentially realizing in situ aliovalent Cd(II) substitution and post-synthetic ligand esterolysis on a 3-D diamond-like open framework In-BQ, we have successfully acquired the modified framework Cd-BQ-COOH, which possesses the doubling of Me2NH2+ proton carriers and a great number of residual –COOH groups. These proton sources and hopping sites are beneficial to establish abundant hydrogen-bond networks and strong host–guest interaction, which lead to a 300-fold enhanced conductivity compared with that of pristine In-BQ, reaching 6.06 × 10−2 S cm−1 at 303 K and 95% RH. This work not only confirms the effectiveness and feasibility of the aliovalent metal substitution strategy extended to ligand modification but also provides a promising route to maximize the proton conduction performance of MOF materials.
Author contributions
Hui Gao: Conceptualization, investigation, formal analysis, visualization, writing-original draft. Ying-Xia Wang: Investigation, formal analysis, writing-review & editing. Yan-Bin He: Conceptualization (computations), investigation (computations). Xian-Ming Zhang: Conceptualization, project administration, funding acquisition, supervision, writing-review & editing.
Conflicts of interest
The authors declare that they have no conflict of interest. The authors declare no competing financial interests.
Acknowledgements
We thank the support of NSFC (21871167), 1331 Project of Shanxi, Shanxi Province Science Foundation for Youths (201901D211391), research project supported by Shanxi Scholarship Council of China (2020-088), and the Technology Innovation Team (CX201904).
Notes and references
- K. D. Kreuer, S. J. Paddison, E. Spohr and M. Schuster, Transport in proton conductors for fuel-cell applications: simulations, elementary reactions, and phenomenology, Chem. Rev., 2004, 104, 4637–4678 CrossRef CAS.
- H. Zhang and P. K. Shen, Recent development of polymer electrolyte membranes for fuel cells, Chem. Rev., 2012, 112, 2780–2832 CrossRef CAS.
- X. Meng, H. N. Wang, S. Y. Song and H. J. Zhang, Proton-conducting crystalline porous materials, Chem. Soc. Rev., 2017, 46, 464–480 RSC.
- A. Shigematsu, T. Yamada and H. Kitagawa, Wide control of proton conductivity in porous coordination polymers, J. Am. Chem. Soc., 2011, 133, 2034–2036 CrossRef CAS.
- E. A. Sanginov, K. S. Novikova, N. N. Dremova and Y. A. Dobrovolskii, Formation of proton-conducting polymer additives based on sulfonated crosslinked polystyrene in nafion membranes, Polym. Chem., 2019, 61, 98–107 CAS.
- H. Y. Sun, S. H. Sun, B. Hu, L. K. Gong, Y. M. Zou, J. L. Li, M. L. Feng and X. Y. Huang, Anisotropic proton conduction realized by a layered vanadium selenite single crystal, Inorg. Chem. Front., 2020, 7, 1699–1703 RSC.
- J. H. Wang, Y. Zhang, M. Li, S. Yan, D. Li and X. M. Zhang, Solvent-assisted metal metathesis: a highly efficient and versatile route towards synthetically demanding chromium metal-organic frameworks, Angew. Chem., Int. Ed., 2017, 56, 6478–6482 CrossRef CAS.
- R. X. Yao, H. H. Fu, B. Yu and X. M. Zhang, Chiral metal-organic frameworks constructed from four-fold helical chain SBUs for enantioselective recognition of α-hydroxy/amino acids, Inorg. Chem. Front., 2018, 5, 153–159 RSC.
- X. Chen and G. Li, Proton conductive Zr-based MOFs, Inorg. Chem. Front., 2020, 7, 3765–3784 RSC.
- Q. X. Wang and G. Li, Bi(III) MOFs: syntheses, structures and applications, Inorg. Chem. Front., 2021, 8, 572–589 RSC.
- A. L. Li, Q. Gao, J. Xu and X. H. Bu, Proton-conductive metal-organic frameworks: recent advances and perspectives, Coord. Chem. Rev., 2017, 344, 54–82 CrossRef CAS.
- T. Panda, T. Kundu and R. Banerjee, Self-assembled one dimensional functionalized metal-organic nanotubes (MONTs) for proton conduction, Chem. Commun., 2012, 48, 5464–5466 RSC.
- X. X. Xie, Y. C. Yang, B. H. Dou, Z. F. Li and G. Li, Proton conductive carboxylate-based metal–organic frameworks, Coord. Chem. Rev., 2020, 403, 2131001–2131031 CrossRef.
- J. Jiang and O. M. Yaghi, Bronsted acidity in metal-organic frameworks, Chem. Rev., 2015, 115, 6966–6997 CrossRef CAS PubMed.
- S. Chand, S. M. Elahi, A. Pal and M. C. Das, Metal-organic frameworks and other crystalline materials for ultrahigh superprotonic conductivities of 10−2 S cm−1 or higher, Chem, 2019, 25, 6259–6269 CrossRef CAS PubMed.
- S. J. Liu, C. Cao, F. Yang, M. H. Yu, S. L. Yao, T. F. Zheng, W. W. He, H. X. Zhao, T. L. Hu and X. H. Bu, High proton conduction in two CoII and MnII anionic metal–organic frameworks derived from 1,3,5-benzenetricarboxylic acid, Cryst. Growth Des., 2016, 16, 6776–6780 CrossRef CAS.
- S. Horike, D. Umeyama, M. Inukai, T. Itakura and S. Kitagawa, Coordination-network-based ionic plastic crystal for anhydrous proton conductivity, J. Am. Chem. Soc., 2012, 134, 7612–7615 CrossRef CAS PubMed.
- J. Taylor, M. Komatsu, T. Dekura, S. Otsubo, K. M. Takata and H. Kitagawa, The role of a three dimensionally ordered defect sublattice on the acidity of a sulfonated metal-organic framework, J. Am. Chem. Soc., 2015, 137, 11498–11506 CrossRef CAS.
- T. H. N. Lo, M. V. Nguyen and T. N. Tu, An anchoring strategy leads to enhanced proton conductivity in a new metal-organic framework, Inorg. Chem. Front., 2017, 4, 1509–1516 RSC.
- W. J. Phang, H. Jo, W. R. Lee, J. H. Song, K. Yoo, B. S. Kim and C. S. Hong, Superprotonic conductivity of a UiO-66 framework functionalized with sulfonic acid groups by facile postsynthetic oxidation, Angew. Chem., 2015, 127, 5231–5235 CrossRef.
- R. L. Liu, D. Y. Wang, J. R. Shi and G. Li, Proton conductive metal sulfonate frameworks, Coord. Chem. Rev., 2021, 431, 213747–213772 CrossRef CAS.
- H. B. Luo, Q. Ren, P. Wang, J. Zhang, L. Wang and X. M. Ren, High proton conductivity achieved by encapsulation of imidazole molecules into proton-conducting MOF-808, ACS Appl. Mater. Interfaces, 2019, 11, 9164–9171 CrossRef CAS.
- V. G. Ponomareva, K. A. Kovalenko, A. P. Chupakhin, D. N. Dybtsev, E. S. Shutova and V. P. Fedin, Imparting high proton conductivity to a metal-organic framework material by controlled acid impregnation, J. Am. Chem. Soc., 2012, 134, 15640–15643 CrossRef CAS PubMed.
- X. M. Li, L. Z. Dong, S. L. Li, G. Xu, J. Liu, F. M. Zhang, L. S. Lu and Y. Q. Lan, Synergistic conductivity effect in a proton sources-coupled metal-organic framework, ACS Energy Lett., 2017, 2, 2313–2318 CrossRef CAS.
- F. Yang, G. Xu, Y. Dou, B. Wang, H. Zhang, H. Wu, W. Zhou, J. R. Li and B. Chen, A flexible metal-organic framework with a high density of sulfonic acid sites for proton conduction, Nat. Energy, 2017, 2, 877–883 CrossRef CAS.
- S. Kim, B. Joarder, J. A. Hurd, J. Zhang, K. W. Dawson, B. S. Gelfand, N. E. Wong and G. K. H. Shimizu, Achieving superprotonic conduction in metal-organic frameworks through iterative design advances, J. Am. Chem. Soc., 2018, 140, 1077–1082 CrossRef CAS.
- D. W. Lim and H. Kitagawa, Rational strategies for proton-conductive metal-organic frameworks, Chem. Soc. Rev., 2021, 50, 6349–6368 RSC.
- D. W. Lim and H. Kitagawa, Proton transport in metal-organic frameworks, Chem. Rev., 2020, 120, 8416–8467 CrossRef CAS.
- H. N. Wang, H. X. Sun, Y. M. Fu, X. Meng, Y. H. Zou, Y. O. He and R. G. Yang, Varied proton conductivity and photoreduction CO2 performance of isostructural heterometallic cluster based metal-organic frameworks, Inorg. Chem. Front., 2021, 8, 4062–4071 RSC.
- H. Gao, Y. B. He, J. J. Hou and X. M. Zhang, In situ aliovalent nickle substitution and acidic modification of nanowalls promoted proton conductivity in a In-OF with 1-D helical channel, ACS Appl. Mater. Interfaces, 2021, 13, 38289–38295 CrossRef CAS PubMed.
- H. Gao, Y. B. He, J. J. Hou, Q. G. Zhai and X. M. Zhang, Enhanced proton conductivity by aliovalent substitution of cadmium for indiumin dimethylaminium templated metal anilicates, ACS Appl. Mater. Interfaces, 2020, 12, 41605–41612 CrossRef CAS.
- J. M. Soler, E. Artacho, J. D. Gale, A. García, J. Junquera, P. Ordejón and D. Sánchez-Portal, The siesta method for ab Initio order-N materials simulation, J. Phys.: Condens. Matter., 2002, 14, 2745–2779 CrossRef CAS.
- J. P. Perdew, K. Burke and M. Ernzerhof, Generalized gradient approximation made simple, Phys. Rev. Lett., 1996, 77, 3865–3868 CrossRef CAS PubMed.
- N. Troullier and J. L. Martins, Efficient pseudopotentials for plane-wave calculations, Phys. Rev. B: Condens. Matter Mater. Phys., 1991, 43, 1993–2006 CrossRef CAS PubMed.
- S. Nosé, A unified formulation of the constant
temperature molecular dynamics methods, J. Chem. Phys., 1984, 81, 511–519 CrossRef.
- D. Umeyama, S. Horike, M. Inukai, Y. Hijikata and S. Kitagawa, Confinement of mobile histamine in coordination nanochannels for fast proton transfer, Angew. Chem., Int. Ed., 2011, 50, 11706–11709 CrossRef CAS PubMed.
- T. Panda, T. Kundu and R. Banerjee, Structural isomerism leading to variable proton conductivity in Indium(III) isophthalic acid based frameworks, Chem. Commun., 2013, 49, 6197–6199 RSC.
- L. F. Zou, S. Yao, J. Zhao, D. S. Li, G. H. Li, Q. S. Huo and Y. L. Liu, Enhancing proton conductivity in a 3D metal-organic framework by the cooperation of guest [Me2NH2]+ cations, water molecules, and host carboxylates, Cryst. Growth Des., 2017, 17, 3556–3561 CrossRef CAS.
- S. C. Pal, S. Chand, A. G. Kumar, P. G. M. Mileo, I. Silverwood, G. Maurin, S. Banerjee, S. M. Elahi and M. C. Das, , A Co(II)-coordination polymer for ultrahigh superprotonic conduction: an atomistic insight through molecular simulations and QENS experiments, J. Mater. Chem. A, 2020, 8, 7847–7853 RSC.
- M. Wahiduzzaman, S. Wang, J. Schnee, A. Vimont, V. Ortiz, P. G. Yot, R. Retoux, M. Daturi, J. S. Lee, J. S. Chang, C. Serre, G. Maurin and S. Devautour-Vinot, A high proton conductive hydrogen-sulfate decorated titanium carboxylate metal-organic framework, ACS Sustainable Chem. Eng., 2019, 7, 5776–5783 CrossRef CAS.
- D. W. Lim, M. Sadakiyo and H. Kitagawa, Proton transfer in hydrogen-bonded degenerate systems of water and ammonia in metal-organic frameworks, Chem. Sci., 2019, 10, 16–33 RSC.
- E. Eisbein, J. O. Joswig and G. Seifert, Proton conduction in a mil-53(Al) metal-organic framework: confinement versus host/guest interaction, J. Phys. Chem. C, 2014, 118, 13035–13041 CrossRef CAS.
- T. Grancha, J. Ferrando-Soria, J. Cano, P. Amorós, B. Seoane, J. Gascon, M. Bazaga-García, E. R. Losilla, A. Cabeza, D. Armentano and E. Pardo, Insights into the dynamics of grotthuss mechanism in a proton-conducting chiral Biomof, Chem. Mater., 2016, 28, 4608–4615 CrossRef CAS.
Footnotes |
† Electronic supplementary information (ESI) available: Proton-conducting measurement, H2O adsorption, IR, PXRD, TGA, MS, NMR data (PDF). ESI Videos of Cd-BQ-COOH and In-BQ-COOH are showing the proposed conduction mechanism (MP4). See DOI: https://doi.org/10.1039/d2qi00407k |
‡ These authors contribute equally to this work. |
|
This journal is © the Partner Organisations 2022 |