DOI:
10.1039/D2QI00125J
(Research Article)
Inorg. Chem. Front., 2022,
9, 3130-3137
Bimetallic sulfide particles incorporated in Fe/Co-based metal–organic framework ultrathin nanosheets toward boosted electrocatalysis of the oxygen evolution reaction†
Received
15th January 2022
, Accepted 29th April 2022
First published on 30th April 2022
Abstract
The development of inexpensive, high-performance, and long-lasting electrocatalysts toward the oxygen evolution reaction (OER) has been proved to be crucial to enhance the efficiency of water splitting to obtain clean and sustainable energy. Herein, Fe/Co-based metal–organic framework (MOF) ultrathin nanosheets doped with (Fe)-Co3S4 metal sulfide (MS) particles (CoFe-MS/MOF) are reported as superior electrocatalysts for the OER. CoFe-MS/MOFs exhibit an outstanding OER performance with a small overpotential of 261 mV and a low Tafel slope of 60.3 mV dec−1 at a current density of 10 mA cm−2 while showing high durability in an alkaline medium. The existence of metal sulfide particles plays a pivotal part in promoting the charge transfer capacity as well as the formation of ultrathin MOF nanosheets. More significantly, they can also modulate the adsorption energy of oxygenated intermediates, thereby significantly enhancing the inherent electrocatalytic activity. This work provides guidance for rationally designing and synthesizing inexpensive and highly active bimetallic sulfide doped MOF electrocatalysts in the electrochemical energy field.
1. Introduction
Due to severe environmental pollution and energy depletion resulting from extensive consumption of fossil energy, numerous researches have been dedicated to developing sustainable and clean energy sources,1–4 among which, water electrolysis for the production of hydrogen is an eco-friendly and economically viable strategy.5–7 Nevertheless, the sluggish OER severely affects the efficiency of overall water splitting. Since OER is a thermodynamically unfavorable process involving the transfer of four proton-coupled electron, it is a bottleneck for water splitting.8–10 Therefore, exploring efficient OER electrocatalysts is a key requirement for accelerating the reaction by reducing the activation energy, and thus achieving an improvement of energy conversion efficiency.11 Although Ir/Ru-based precious metal materials remain the state-of-the-art electrocatalysts toward OER by far, the scarcity, inferior durability and high cost severely limit their large-scale industrial application.12,13 As a consequence, development of efficient, cost-effective, and stable noble-metal-free electrocatalysts toward the OER is of considerable scientific and research significance.14,15
In recent years, a large amount of research has been dedicated to exploiting highly efficient and cost-effective electrocatalysts based on 3d transition metals (Co, Ni, Fe, etc.) for the OER due to their elemental abundance in the Earth's crust and similar valence electron structures of 3d6–84s2.16 Among them, metal–organic frameworks (MOFs) have emerged as promising OER catalysts owing to their intrinsically large surface area and porosity.15,17,18 In electrocatalysis, MOFs are usually utilized as precursors or sacrificial templates for the preparation of different electrocatalysts, such as metal sulfides,19 nitrides20 and carbides21 by pyrolysis under various conditions. However, the attractive structural and compositional characteristics of precursors will be completely destroyed during pyrolysis, causing restricted active sites and blocked reactant transport.22 Still, it is reasonable for MOFs to be used directly as (pre-)electrocatalysts to make the best of their distinctive properties. Nevertheless, the catalytic activity of pristine MOF materials is unsatisfactory, primarily on account of their poor inherent charge/mass transport capability.23–25
The inherently poor conductivity of MOFs limits their electrocatalytic applications. To address this issue, abundant studies have been devoted to enhancing the electrical conductivity of MOFs. The incorporation of metal chalcogenide and oxide nanoparticles into MOFs through a post-synthesis process and the creation of polymetallic MOFs with more open metal sites and an enriched electron environment could effectively enhance the electrical conductivity and electrocatalytic properties of MOFs.26–30 Furthermore, MOFs with high electrical conductivity have also been developed by using ligands with a π-conjugated aromatic structure.31,32 Mass transfer in MOFs is restricted by the mismatched porous size of MOFs for the diffusion of reactants. The large mass resistance results in low exposure and underutilization of the active sites. In this aspect, two-dimensional ultrathin nanosheet-like MOF materials are highly satisfactory and can be successfully developed by liquid–liquid interfacial growth, ultrasound-assisted exfoliation, sacrificial template growth, epitaxial growth, and other methods, which exhibit excellent electrocatalytic properties.25,33,34
Another superior strategy to improve the electrocatalytic activity of MOFs is the introduction of heterogeneous species into pristine MOFs.35 In previous reports, tiny Ni3S2 or CoFeOx nanoparticles embedded in MOFs by post-synthetic plasma treatment were able to enhance the electrical conductivity and electrocatalytic performance of MOFs.27,28 Such heterogeneous species can enhance the surface area and availability of active sites through modulating the nanostructure of MOFs. Moreover, they can also trigger probable synergistic effects between diverse constituents through the generation of interfacial electronic coupling, providing a huge opportunity to optimize the adsorption energy of key intermediates, thereby enhancing the inherent catalytic activity.36–38
Inspired by the above work, we report the CoFe-MS/MOF electrocatalyst formed by embedding metal sulfide particles into ultrathin Fe/Co-based MOF nanosheets using a facile one-step solvothermal method. It exhibits more outstanding OER electrocatalytic activity (10 mA cm−2 at 261 mV for the OER) than CoNi-MS/MOF, CoCu-MS/MOF, CoFe-MOF and (Fe)-Co3S4. It is found that the existence of iron/cobalt sulfide particles is essential to improving the electrocatalytic properties. Embedding sulfide particles in MOFs not only contributes to the formation of ultrathin nanosheets, but also effectively improves the electrical conductivity of the nanosheets and, more significantly, modulates the surface properties and electronic structure of the electrocatalysts, which together enhance the electrocatalytic activity.
2. Results and discussion
As illustrated in Fig. 1, the CoFe-MS/MOF nanosheets were synthesized in the presence of thioacetamide (TAA, a S source) and terephthalic acid (TPA, the MOF ligand) under solvothermal conditions with an input Fe3+/Co2+ molar ratio of 1
:
4. For comparison, we also synthesized CoNi-MS/MOF and CoCu-MS/MOF nanosheets by the same method. The X-ray diffraction (XRD) pattern of the as-synthesized CoFe-MS/MOF (Fig. 2a) displays some characteristic diffraction peaks similar to those of the MOF-71 structure (no. 265 092, Cambridge Crystallographic Data Centre).22,39 Two sharp diffraction peaks at 19.75° and 22.19° correspond to singlet sulfur (S, PDF#42-1278), probably due to the oxidation of sulfide in air. Some diffraction peaks at 16.37°, 32.14°, 38.33°, 47.21°, 50.53°, and 55.51° correspond to cobalt disulphide (Co3S4, PDF#47-1738), respectively.26 The other diffraction peaks correspond to Fe3S4 (PDF#16-0713). This XRD pattern reveals that bimetallic sulfides and MOF-71 type MOFs coexist in the as-prepared CoFe-MS/MOF. As presented in the scanning electron microscopy (SEM) images of Fig. 2b and c, the CoFe-MS/MOF consists of a large number of ultrathin shuttle-shaped nanosheets (≈15–25 nm in thickness), and these ultrathin nanosheets form a large number of empty spaces by interconnecting and intersecting with each other. This distinctive open and layered porous structure facilitates mass transfer and provides highly specific surface areas for accelerating electrochemical reactions.40–42
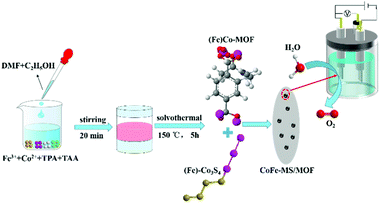 |
| Fig. 1 Schematic diagram of the synthesis procedure for the CoFe-MS/MOF. | |
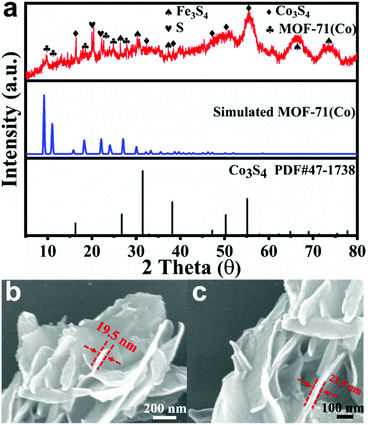 |
| Fig. 2 (a) XRD patterns and (b and c) SEM images of the CoFe-MS/MOF. | |
To further investigate the microstructural characteristics of the CoFe-MS/MOF, transmission electron microscopy (TEM) tests were performed. The CoFe-MS/MOF is composed of various ultrathin nanosheets, as shown in the TEM image in Fig. 3a, which is consistent with the results observed by SEM. Compared to the CoNi-MS/MOF and CoCu-MS/MOF (Fig. S1†), the CoFe-MS/MOF with similar morphologies is significantly thinner. There were some black nanoparticles embedded in the ultrathin nanosheets (Fig. 3b), but no visible cracks, which implies good mechanical and electrical touch between various assemblies. The corresponding high-resolution TEM (HRTEM) image of the CoFe-MS/MOF (Fig. 3c) demonstrates the lattice d-spacing of approximately 0.209 and 0.254 nm, corresponding to the (400) and (422) lattices of Co3S4, agreeing well with the XRD pattern in Fig. 2a. It is noteworthy that no lattice stripes were observed for MOF-71, probably due to the disruption of the crystal structure of the MOF under a high-energy electron flow.28,43
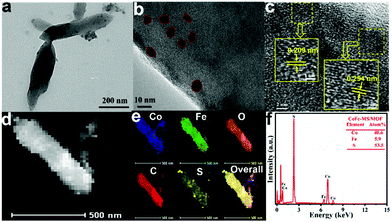 |
| Fig. 3 (a) TEM image, (b and c) HRTEM images, (d) HAADF-STEM image, (e) the corresponding elemental mapping images of Co, Fe, O, C, and S, and (f) the SEM-EDX spectrum of the CoFe-MS/MOF nanosheet. | |
The high-angle annular dark-field scanning TEM (HAADF-STEM) image (Fig. 3d) and corresponding energy-dispersive X-ray (EDX) mapping images (Fig. 3e) of a single CoFe-MS/MOF nanosheet display the even distribution of Co, Fe, O, and C elements and the discrete distribution of the S element throughout the entire nanosheet, indicating that metal sulfide particles are successfully embedded in the Fe/Co-based MOF nanosheet. The EDX spectrum (Fig. 3f) unveils an Fe/Co atomic ratio of approximately 1
:
6.9 in the nanosheet, which is marginally different from the Fe/Co ratio employed for the solvothermal reaction, probably due to the different coordination capabilities of Co2+ and Fe3+ during the MOF growth.44 The EDX data of CoNi-MS/MOF and CoCu-MS/MOF are displayed in Fig. S2.†
To investigate the formation mechanism of this metal sulfide particle inlaid in MOF ultrathin nanosheets, a series of controlled experiments was conducted. In the absence of TAA or TPA, the products are referred to as CoFe-MOF and (Fe)-Co3S4, respectively. The control experiments revealed that the CoFe-MOF displays several characteristic peaks of the MOF-71 MOF without the peaks of metal sulfide, while pure metal sulfide (Fe)-Co3S4 is the opposite (Fig. S3†). In this situation, TAA releases S2− ions as a vulcanization reagent to form bimetallic sulfides with the coexistence of Co3S4 and Fe3S4 under solvothermal conditions.45 Additionally, the diffraction peak intensity of the metal sulfide is positively correlated with the TAA dosage at the same TPA dosage, indicating that the sulfide amount in the nanosheets can be tuned by the TAA content. Notably, both the shuttle-shaped CoFe-MOF and the hollow microspherical (Fe)-Co3S4 are thicker than the CoFe-MS/MOF (Fig. S4†), indicating that the inlaid sulfide in the Fe/Co-based MOF is conducive to the formation of ultrathin nanosheets. In the current situation, the diffraction peaks of the MOF are proved to be rather weak, whereas those of sulfide are proved to be intense for a shorter growth time. Consequently, we assume S2− liberated by TAA first reacts with the metal (Co and Fe) ions to form sulfide nanomicrospheres, and is subsequently converted into metal sulfide particles inlaid in the MOF ultrathin nanosheets in the presence of TPA ligands.46
Among a series of bimetallic and monometallic catalysts, the optimized CoFe-MS/MOF catalyst synthesized with a 1
:
4 Fe/Co ratio after five hours of the solvothermal reaction displayed optimal OER activity in 1 M KOH (Fig. S5b and S6–S8†). As shown by the polarization curves in Fig. 4a, the CoFe-MS/MOF exhibits attractive OER activity as well as the lowest onset potential, which is significantly superior to those of the CoFe-MOF, (Fe)-Co3S4, CoCu-MS/MOF, CoNi-MS/MOF, and RuO2. As shown in Fig. 4b, the CoFe-MS/MOF only demands a low overpotential of 261 mV to deliver a current density of 10 mA cm−2, which is far smaller than those of the CoFe-MOF (312 mV), (Fe)-Co3S4 (306 mV), the CoNi-MS/MOF (305 mV), the CoCu-MS/MOF (299 mV), and commercial RuO2 (313 mV). Meanwhile, the CoFe-MS/MOF electrode also displays a smaller Tafel slope (60.3 mV dec−1) than those of the CoFe-MOF (73.6 mV dec−1), (Fe)-Co3S4 (67.1 mV dec−1), the CoNi-MS/MOF (111.8 mV dec−1), the CoCu-MS/MOF (130.2 mV dec−1), and RuO2 (86.5 mV dec−1), which is shown in Fig. 4c, implying more beneficial OER kinetics. In addition, electrochemical impedance spectra (EIS) were measured to study the charge transport and conductivity of catalysts. As indicated in Fig. 4d, the Nyquist plots can be fitted using an equivalent circuit, and the corresponding fitted results are presented in Table S1.† The terms Rs, CPE, and Rct represent the solution resistance, constant phase elements, and charge-transfer resistance, respectively. Obviously, the charge transfer resistance (Rct) of the CoFe-MS/MOF is only 9.50 Ω, much smaller than those of the CoFe-MOF (51.80 Ω), (Fe)-Co3S4 (10.10 Ω), the CoNi-MS/MOF (72.92 Ω), and the CoCu-MS/MOF (14.60 Ω), suggesting faster OER kinetics of the CoFe-MS/MOF in comparison with that of the other four electrocatalysts. It primarily arises from the synergistic effect between the incorporated sulfide particles and the MOF, which diminishes the charge transfer resistance, and the ultrathin nanosheet structure, which shortens the electron transfer distance.25,47 The above comparisons clearly disclose that embedding sulfide particles into MOF nanosheets can essentially improve the OER activity of the as-prepared CoFe-MS/MOF electrocatalyst. The CoFe-MS/MOF also exhibits excellent OER durability. As shown in the current density over time (i–t) curve in Fig. 4e, the current density exhibits a slow decline. Simultaneously, the chronoamperometric curve at a current density of 10 mA cm−2 reveals that the CoFe-MS/MOF remains quite durable after at least 40 h of continuous electrolysis (Fig. 4f), demonstrating superior durability toward the OER.
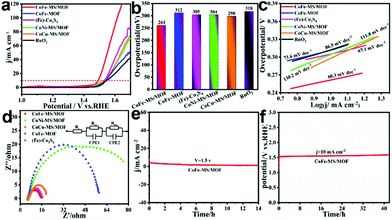 |
| Fig. 4 Electrocatalytic OER activities in 1 M KOH. (a) Polarization curves, (b) histogram of overpotentials, and (c) Tafel plots at a current density of 10 mA cm−2. (d) Nyquist plots. (e) The i–t curve under an overpotential of 270 mV over 14 h and (f) the prolonged chronopotentiometric curve over 40 h for the CoFe-MS/MOF. | |
To investigate the possible origin of the considerably boosted water oxidation activity mentioned above, electrochemical surface areas (ECSAs) of the CoFe-MS/MOF, the CoFe-MOF, (Fe)-Co3S4, the CoNi-MS/MOF, and the CoCu-MS/MOF were compared through their double-layer capacitance (Cdl) (Fig. 5a and Fig. S8†).48 As shown in Fig. 5b, the Cdl value of the CoFe-MS/MOF (7.71 mF cm−2) is 2.6-, 4.7-, 4.0-, and 6.3-fold that of the CoFe-MOF, (Fe)-Co3S4, the CoNi-MS/MOF, and the CoCu-MS/MOF, individually, indicating a larger ECSA of the CoFe-MS/MOF, in accordance with the SEM and TEM observations. The electrocatalytic OER performance of the CoFe-MS/MOF and other comparison samples is listed in Table S2.† Nevertheless, the OER activity of the CoFe-MS/MOF fails to increase by a corresponding multiple, suggesting that it is not only determined by the increased ECSAs, but is also closely associated with the synergistic effect between the MOF nanosheets and the incorporated sulfide particles.
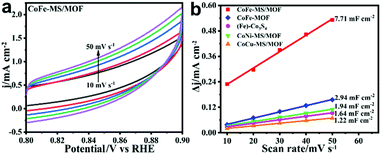 |
| Fig. 5 (a) Cyclic voltammograms of the CoFe-MS/MOF. (b) Double layer currents of different catalysts versus the scan rate. | |
In order to illuminate the probable mechanism underlying such a synergistic effect, the alterations of the CoFe-MS/MOF in the structure and composition after electroactivation were investigated. After electrochemical activation (usually 20 cycles between 1.0 and 1.7 V (vs. RHE) at 10 mV s−1), the diffraction peaks of the metal sulfide show no remarkable difference, while those of the MOF entirely vanish, as displayed by the XRD pattern in Fig. 6a. This indicates that the crystal structure of the MOF nanosheets has undergone a transformation during the electroactivation process, whereas the sulfide particles survive, as evidenced by the Raman spectra (Fig. 6b).30,49 The survival of the sulfide particles is possibly due to the fact that they are sheltered from the phase changes during electroactivation by the surrounding oxyhydroxide substrate. The nanosheet morphology of the CoFe-MS/MOF remains invariant, yet its thickness is significantly enlarged (Fig. 6c and d), indicating the formation of (oxy)hydroxides during electrochemical activation, which act as the actual active sites of the OER.26,50,51
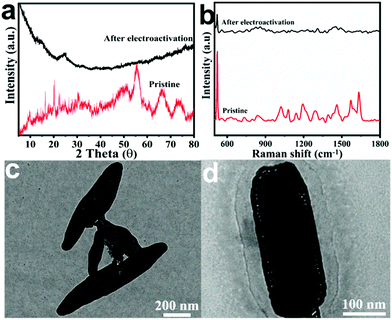 |
| Fig. 6 (a) XRD patterns and (b) Raman spectra of the CoFe-MS/MOF before and after electrochemical activation. (c and d) TEM images of the CoFe-MS/MOF after electrochemical activation. | |
X-ray photoelectron spectroscopy (XPS) was performed to examine the electronic interactions in the CoFe-MS/MOF. The survey spectrum of the CoFe-MS/MOF reveals the existence of Co, Fe, S, O, and C elements, as shown in Fig. 7a. The O 1s spectrum of the CoFe-MS/MOF in Fig. 7b displays three sub-peaks assigned to a M–O bond, a carboxyl (O
C–O–) bond, as well as the absorbed water. After electrochemical activation, the proportion of carboxyl O apparently decreases, whereas the M–O bonds grow, indicating the transformation of the MOF to oxyhydroxide during the OER process. Fig. 7c shows the Co 2p spectra from the CoFe-MS/MOF before and after electroactivation. The binding energies of 781.80 (Co 2p3/2) and 797.70 eV (Co 2p1/2) correspond to the Co2+ species, and 778.73 (Co 2p3/2) and 793.87 eV (Co 2p1/2) are associated with surface-oxidized Co3+, respectively.52 It is notable that the binding energy of Co 2p3/2 for the CoFe-MS/MOF decreases after electrochemical activation (ΔBE = 0.87 eV). The peaks at 711.90 (2p3/2) and 724.83 eV (2p1/2) are indexed to Fe3+ and a resembling binding energy shift is noted in the Fe 2p spectra as well (Fig. 7d).49 This indicates that their electronic structure is apparently altered to an electron-rich state by the metal sites in the CoFe-MS/MOF after electrochemical activation. It is caused by metal sulfide particles conferring electrons from their electron-rich metal atoms to the surface Co and Fe centers.51,53,54
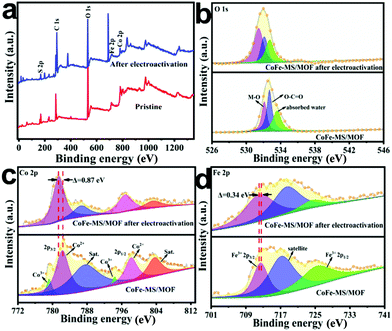 |
| Fig. 7 (a) XPS survey spectra and (b) O 1s, (c) Co 2p, and (d) Fe 2p spectra of the CoFe-MS/MOF before and after electrochemical activation. | |
Recent studies further indicate that the OER activity of Fe/Co-based catalysts can be boosted through enhancing the adsorption of oxygenated intermediates such as *OH and *O on the surface.35,55,56 As shown in Fig. 4c and d, the Fe/Co centers at the electron-rich state favor the enhancement of oxygen-containing intermediates for expedited OER kinetics,16,57 evidenced by the smaller Rct and smaller Tafel slope of the CoFe-MS/MOF in comparison with the CoFe-MOF in this work. Thus, a greater inherent OER activity of the CoFe-MS/MOF stems from the larger electron density at its metal sites, which is caused by the embedded metal sulfide particles.
To estimate the application of the CoFe-MS/MOF as a remarkable OER catalyst in a water-splitting cell, the overall electrocatalytic water decomposition performance of the CoFe-MS/MOF was investigated herein. The CoFe-MS/MOF and the commercial Pt/C catalyst serve as the anodic and cathodic working electrodes (WE) of a double-electrode cell in 1 M KOH, respectively (Fig. 8a). From the polarization curve (Fig. 8b), it is unambiguously discovered that this CoFe-MS/MOF//Pt/C couple entails a smaller cell voltage of 1.54 V in comparison with the RuO2//Pt/C couple to achieve a current density of 10 mA cm−2, reflecting its promising application toward water splitting.
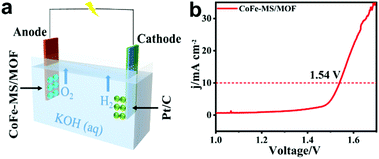 |
| Fig. 8 (a) The schematic diagram of the overall water splitting based on CoFe-MS/MOF//Pt/C electrodes. (b) Polarization curve of the overall water splitting over the CoFe-MS/MOF//Pt/C two-electrode couple. | |
3. Conclusions
To sum up, a range of CoM (M = Fe, Ni, Cu)-MS/MOF, CoFe-MOF, and (Fe)-Co3S4 samples was successfully synthesized through a facile one-step solvothermal method. Among these, the as-prepared CoFe-MS/MOF consisting of interconnected MOF ultrathin nanosheets with metal sulfide nanoparticles exhibits a much lower overpotential, smaller Tafel slope, and excellent stability, which indicate its greatly improved OER activity in alkaline conditions. Furthermore, by coupling with Pt/C, the CoFe-MS/MOF is able to drive water splitting to deliver a current density of 10 mA cm−2 with an ultralow cell voltage of 1.54 V as well, indicating the promising application of the CoFe-MS/MOF for overall water splitting. The boosting mechanism results from the metal sulfide particles embedded in the nanosheets, which facilitates the formation of ultrathin nanosheets to significantly expand the ECSAs, while providing high electrical conductivity but, more significantly, effectively regulates the electronic structure of the catalytically active atomic sites to an electron-rich state through strong electronic interactions, enhancing the adsorption of O-containing intermediates to promote rapid electrochemical reactions. This research offers prospects for the sensible design of high-performance and cost-effective bimetallic sulfide doped MOF materials for electrocatalytic applications.
Conflicts of interest
There are no conflicts to declare.
Acknowledgements
The authors thank the National Natural Science Foundation of China (Grant No. 52073199 and 51873136).
Notes and references
- H. Xu, Y. Zhao, Q. Wang, G. He and H. Chen, Supports promote single-atom catalysts toward advanced electrocatalysis, Coord. Chem. Rev., 2022, 451, 214261–214268 CrossRef CAS
.
- J. Li, C. Wang, H. Shang, Y. Wang, H. You, H. Xu and Y. Du, Metal-modified PtTe2 nanorods: surface reconstruction for efficient methanol oxidation electrocatalysis, Chem. Eng. J., 2021, 424, 130319 CrossRef CAS
.
- H. Xu, Y. Zhao, G. He and H. Chen, Race on engineering noble metal single-atom electrocatalysts for water splitting, Int. J. Hydrogen Energy, 2022, 47, 14257–14279 CrossRef CAS
.
- R. Yu, D. Liu, M. Yuan, Y. Wang, C. Ye, J. Li and Y. Du, Universal MOF-Mediated synthesis of 2D CoNi-based layered triple hydroxides electrocatalyst for efficient oxygen evolution reaction, J. Colloid Interface Sci., 2021, 602, 612–618 CrossRef CAS PubMed
.
- P. Zhai, M. Xia, Y. Wu, G. Zhang, J. Gao, B. Zhang, S. Cao, Y. Zhang, Z. Li, Z. Fan, C. Wang, X. Zhang, J. T. Miller, L. Sun and J. Hou, Engineering single-atomic ruthenium catalytic sites on defective nickel-iron layered double hydroxide for overall water splitting, Nat. Commun., 2021, 12, 1–11 CrossRef PubMed
.
- Q. Mou, Z. Xu, G. Wang, E. Li, J. Liu, P. Zhao, X. Liu, H. Li and G. Cheng, A bimetal hierarchical layer structure MOF grown on Ni foam as a bifunctional catalyst for the OER and HER, Inorg. Chem. Front., 2021, 8, 2889–2899 RSC
.
- H. Xu, H. Shang, C. Wang and Y. Du, Low–dimensional metallic nanomaterials for advanced electrocatalysis, Adv. Funct. Mater., 2020, 30, 2006317 CrossRef CAS
.
- Z. Li, X. Xu, X. Lu, C. He, J. Huang, W. Sun and L. Tian, Synergistic coupling of FeNi3 alloy with graphene carbon dots for advanced oxygen evolution reaction electrocatalysis, J. Colloid Interface Sci., 2022, 615, 273–281 CrossRef CAS PubMed
.
- J. Wang, S.-J. Kim, J. Liu, Y. Gao, S. Choi, J. Han, H. Shin, S. Jo, J. Kim, F. Ciucci, H. Kim, Q. Li, W. Yang, X. Long, S. Yang, S.-P. Cho, K. H. Chae, M. G. Kim, H. Kim and J. Lim, Redirecting dynamic surface restructuring of a layered transition metal oxide catalyst for superior water oxidation, Nat. Catal., 2021, 4, 212–222 CrossRef CAS
.
- Y. Wang, C. Wang, H. Shang, M. Yuan, Z. Wu, J. Li and Y. Du, Self-driven Ru-modified NiFe MOF nanosheet as multifunctional electrocatalyst for boosting water and urea electrolysis, J. Colloid Interface Sci., 2022, 605, 779–789 CrossRef CAS PubMed
.
- C. Wang, H. Shang, Y. Wang, H. Xu, J. Li and Y. Du, Interfacial electronic structure modulation enables CoMoOx/CoOx/RuOx to boost advanced oxygen evolution electrocatalysis, J. Mater. Chem. A, 2021, 9, 14601–14606 RSC
.
- R. K. Hona, S. B. Karki, T. Cao, R. Mishra, G. E. Sterbinsky and F. Ramezanipour, Sustainable oxide electrocatalyst for hydrogen- and oxygen-evolution reactions, ACS Catal., 2021, 11, 14605–14614 CrossRef CAS
.
- C. Cai, S. Han, Q. Wang and M. Gu, Direct observation of yolk-shell transforming to gold single atoms and clusters with superior oxygen evolution reaction efficiency, ACS Nano, 2019, 13, 8865–8871 CrossRef CAS PubMed
.
- J. Qi, Y. P. Lin, D. Chen, T. Zhou, W. Zhang and R. Cao, Autologous cobalt phosphates with modulated coordination sites for electrocatalytic water oxidation, Angew. Chem., Int. Ed., 2020, 59, 8917–8921 CrossRef CAS PubMed
.
- S. Pan, X. Mao, J. Yu, L. Hao, A. Du and B. Li, Remarkably improved oxygen evolution reaction activity of cobalt oxides by an Fe ion solution immersion process, Inorg. Chem. Front., 2020, 7, 3327–3339 RSC
.
- T. Wu, X. Ren, Y. Sun, S. Sun, G. Xian, G. G. Scherer, A. C. Fisher, D. Mandler, J. W. Ager, A. Grimaud, J. Wang, C. Shen, H. Yang, J. Gracia, H. J. Gao and Z. J. Xu, Spin pinning effect to reconstructed oxyhydroxide layer on ferromagnetic oxides for enhanced water oxidation, Nat. Commun., 2021, 12, 3634 CrossRef CAS PubMed
.
- X. Wang, X. Wang, L. Zhao, H. Zhang, M. Liu, C. Zhang and S. Liu, Self-reconstruction of cationic activated Ni-MOFs enhanced the intrinsic activity of electrocatalytic water oxidation, Inorg. Chem. Front., 2022, 9, 179–185 RSC
.
- C. Wang, H. Shang, J. Li, Y. Wang, H. Xu, C. Wang, J. Guo and Y. Du, Ultralow Ru doping induced interface engineering in MOF derived ruthenium-cobalt oxide hollow nanobox for efficient water oxidation electrocatalysis, Chem. Eng. J., 2021, 420, 129805 CrossRef CAS
.
- K. Berijani and A. Morsali, The role of metal–organic porous frameworks in dual catalysis, Inorg. Chem. Front., 2021, 8, 3618–3658 RSC
.
- Y. Gu, S. Chen, J. Ren, Y. A. Jia, C. Chen, S. Komarneni, D. Yang and X. Yao, Electronic structure tuning in Ni3FeN/r-GO aerogel toward bifunctional electrocatalyst for overall water splitting, ACS Nano, 2018, 12, 245–253 CrossRef CAS PubMed
.
- Y. Lv and J. R. Gong, In situ growth of MOF-derived ultrafine molybdenum carbide nanoparticles supported on Ni foam as efficient hydrogen-evolution electrocatalysts, J. Mater. Chem. A, 2021, 9, 15246–15253 RSC
.
- M. Zhao, T. Guo, W. Qian, Z. Wang, X. Zhao, L. Wen and D. He, Fe-incorporated cobalt-based metal-organic framework ultrathin nanosheets for electrocatalytic oxygen evolution, Chem. Eng. J., 2021, 422, 130055 CrossRef CAS
.
- Q. Liang, J. Chen, F. Wang and Y. Li, Transition metal-based metal-organic frameworks for oxygen evolution reaction, Coord. Chem. Rev., 2020, 424, 213488 CrossRef CAS
.
- C. Meng, Y. Cao, Y. Luo, F. Zhang, Q. Kong, A. A. Alshehri, K. A. Alzahrani, T. Li, Q. Liu and X. Sun, A Ni-MOF nanosheet array for efficient oxygen evolution electrocatalysis in alkaline media, Inorg. Chem. Front., 2021, 8, 3007–3011 RSC
.
- Z. Li, C. Li, J. Huang, W. Sun, W. Cheng, C. He and L. Tian, Structure engineering of amorphous P–CoS hollow electrocatalysts for promoted oxygen evolution reaction, Int. J. Hydrogen Energy, 2022, 47, 15189–15197 CrossRef CAS
.
- M. Wang, C.-L. Dong, Y.-C. Huang and S. Shen, Operando spectral and electrochemical investigation into the heterophase stimulated active species transformation in transition-metal sulfides for efficient electrocatalytic oxygen evolution, ACS Catal., 2020, 10, 1855–1864 CrossRef
.
- W. Zhang, Y. Wang, H. Zheng, R. Li, Y. Tang, B. Li, C. Zhu, L. You, M. R. Gao, Z. Liu, S. H. Yu and K. Zhou, Embedding ultrafine metal oxide nanoparticles
in monolayered metal-organic framework nanosheets enables efficient electrocatalytic oxygen evolution, ACS Nano, 2020, 14, 1971–1981 CrossRef CAS PubMed
.
- M. Zhao, W. Li, J. Li, W. Hu and C. M. Li, Strong electronic interaction enhanced electrocatalysis of metal sulfide clusters embedded metal-organic framework ultrathin nanosheets toward highly efficient overall water splitting, Adv. Sci., 2020, 7, 2001965 CrossRef CAS PubMed
.
- H. Su, S. Song, S. Li, Y. Gao, L. Ge, W. Song, T. Ma and J. Liu, High-valent bimetal Ni3S2/Co3S4 induced by Cu doping for bifunctional electrocatalytic water splitting, Appl. Catal., B, 2021, 293, 120225 CrossRef CAS
.
- M. Xie, Y. Ma, D. Lin, C. Xu, F. Xie and W. Zeng, Bimetal-organic framework MIL-53(Co-Fe): an efficient and robust electrocatalyst for the oxygen evolution reaction, Nanoscale, 2020, 12, 67–71 RSC
.
- H. Zhong, K. H. Ly, M. Wang, Y. Krupskaya, X. Han, J. Zhang, J. Zhang, V. Kataev, B. Buchner, I. M. Weidinger, S. Kaskel, P. Liu, M. Chen, R. Dong and X. Feng, A phthalocyanine-based layered two-dimensional conjugated metal-organic framework as a highly efficient electrocatalyst for the oxygen reduction reaction, Angew. Chem., Int. Ed., 2019, 58, 10677–10682 CrossRef CAS PubMed
.
- S. S. Shinde, C. H. Lee, J.-Y. Jung, N. K. Wagh, S.-H. Kim, D.-H. Kim, C. Lin, S. U. Lee and J.-H. Lee, Unveiling dual-linkage 3D hexaiminobenzene metal–organic frameworks towards long-lasting advanced reversible Zn–air batteries, Energy Environ. Sci., 2019, 12, 727–738 RSC
.
- Y. Lin, H. Wan, D. Wu, G. Chen, N. Zhang, X. Liu, J. Li, Y. Cao, G. Qiu and R. Ma, Metal-organic framework hexagonal nanoplates: bottom-up synthesis, topotactic transformation, and efficient oxygen evolution reaction, J. Am. Chem. Soc., 2020, 142, 7317–7321 CrossRef CAS PubMed
.
- Z. Li, D. Liu, X. Lu, M. Du, Z. Chen, J. Teng, R. Sha and L. Tian, Boosting oxygen evolution of layered double hydroxide through electronic coupling with ultralow noble metal doping, Dalton Trans., 2022, 51, 1527–1532 RSC
.
- N. Han, S. Luo, C. Deng, S. Zhu, Q. Xu and Y. Min, Defect-rich FeN0.023/Mo2C heterostructure as a highly efficient bifunctional catalyst for overall water-splitting, ACS Appl. Mater. Interfaces, 2021, 13, 8306–8314 CrossRef CAS PubMed
.
- Q. Zhang, M. Zhang, T. Chen, L. Li, S. Shi and R. Jiang, Unconventional phase engineering of fuel-cell electrocatalysts, J. Electroanal. Chem., 2022, 916, 116363 CrossRef CAS
.
- Y. Huang, G. Wei, J. He, C. An, M. Hu, M. Shu, J. Zhu, S. Yao, W. Xi, R. Si, Z.-M. Zhang and C. An, Interfacial electronic interaction of atomically dispersed IrClx on ultrathin Co(OH)2/CNTs for efficient electrocatalytic water oxidation, Appl. Catal., B, 2020, 279, 119398 CrossRef CAS
.
- H. Xu, B. Huang, Y. Zhao, G. He and H. Chen, Engineering Heterostructured Pd–Bi2Te3 Doughnut/Pd Hollow Nanospheres for Ethylene Glycol Electrooxidation, Inorg. Chem., 2022, 61, 4533–4540 CrossRef CAS PubMed
.
- N. L. Rosi, J. Kim, M. Eddaoudi, B. Chen, M. O'Keeffe and O. M. Yaghi, Rod packings and metal–organic frameworks constructed from rod-shaped secondary building units, J. Am. Chem. Soc., 2005, 127, 1504–1518 CrossRef CAS PubMed
.
- X. Zhu, J. Dai, L. Li, D. Zhao, Z. Wu, Z. Tang, L.-J. Ma and S. Chen, Hierarchical carbon microflowers supported defect-rich Co3S4 nanoparticles: An efficient electrocatalyst for water splitting, Carbon, 2020, 160, 133–144 CrossRef CAS
.
- Z. Ye, Y. Jiang, L. Li, F. Wu and R. Chen, A high-efficiency CoSe electrocatalyst with hierarchical porous polyhedron nanoarchitecture for accelerating polysulfides conversion in Li-S batteries, Adv. Mater., 2020, 32, 2002168 CrossRef CAS PubMed
.
- L. Yang, L. Zeng, H. Liu, Y. Deng, Z. Zhou, J. Yu, H. Liu and W. Zhou, Hierarchical microsphere of MoNi porous nanosheets as electrocatalyst and cocatalyst for hydrogen evolution reaction, Appl. Catal., B, 2019, 249, 98–105 CrossRef CAS
.
- Z. Zou, T. Wang, X. Zhao, W.-J. Jiang, H. Pan, D. Gao and C. Xu, Expediting in-Situ electrochemical activation of two-dimensional metal–organic frameworks for enhanced OER intrinsic activity by iron incorporation, ACS Catal., 2019, 9, 7356–7364 CrossRef CAS
.
- J. Xu, X. Zhu and X. Jia, From low- to high-crystallinity bimetal–organic framework nanosheet with highly exposed boundaries: an efficient and stable electrocatalyst for oxygen evolution reaction, ACS Sustainable Chem. Eng., 2019, 7, 16629–16639 CrossRef CAS
.
- Y. Guo, J. Tang, H. Qian, Z. Wang and Y. Yamauchi, One-pot synthesis of zeolitic imidazolate framework 67-derived hollow Co3S4@MoS2 heterostructures as efficient bifunctional catalysts, Chem. Mater., 2017, 29, 5566–5573 CrossRef CAS
.
- X. Zhang, B. Li, M. Lan, S. Yang, Q. Xie, J. Xiao, F. Xiao and S. Wang, Cation modulation of cobalt sulfide supported by mesopore-rich hydrangea-like carbon nanoflower for oxygen electrocatalysis, ACS Appl. Mater. Interfaces, 2021, 13, 18683–18692 CrossRef CAS PubMed
.
- G. Zhang, Y. Li, X. Xiao, Y. Shan, Y. Bai, H. G. Xue, H. Pang, Z. Tian and Q. Xu, In situ anchoring polymetallic phosphide nanoparticles within porous prussian blue analogue nanocages for boosting oxygen evolution catalysis, Nano Lett., 2021, 21, 3016–3025 CrossRef CAS PubMed
.
- S. Niu, W. J. Jiang, Z. Wei, T. Tang, J. Ma, J. S. Hu and L. J. Wan, Se-doping activates FeOOH for cost-effective and efficient electrochemical water oxidation, J. Am. Chem. Soc., 2019, 141, 7005–7013 CrossRef CAS PubMed
.
- K. Ge, S. Sun, Y. Zhao, K. Yang, S. Wang, Z. Zhang, J. Cao, Y. Yang, Y. Zhang, M. Pan and L. Zhu, Facile synthesis of two-dimensional iron/cobalt metal-organic framework for efficient oxygen evolution electrocatalysis, Angew. Chem., Int. Ed., 2021, 60, 12097–12102 CrossRef CAS PubMed
.
- X. Chen, D.-D. Ma, B. Chen, K. Zhang, R. Zou, X.-T. Wu and Q.-L. Zhu, Metal–organic framework-derived mesoporous carbon nanoframes embedded with atomically dispersed Fe–N active sites for efficient bifunctional oxygen and carbon dioxide electroreduction, Appl. Catal., B, 2020, 267, 118720 CrossRef CAS
.
- T. I. Singh, G. Rajeshkhanna, U. N. Pan, T. Kshetri, H. Lin, N. H. Kim and J. H. Lee, Alkaline water splitting enhancement by MOF-derived Fe-Co-oxide/Co@NC-mNS heterostructure: boosting OER and HER through defect engineering and in situ oxidation, Small, 2021, 17, 2101312 CrossRef CAS PubMed
.
- M. Arif, G. Yasin, L. Luo, W. Ye, M. A. Mushtaq, X. Fang, X. Xiang, S. Ji and D. Yan, Hierarchical hollow nanotubes of NiFeV-layered double hydroxides@CoVP heterostructures towards efficient, pH-universal electrocatalytical nitrogen reduction reaction to ammonia, Appl. Catal., B, 2020, 265, 118559 CrossRef CAS
.
- H. Xu, H. Shang, C. Wang and Y. Du, Surface and interface engineering of noble-metal-free electrocatalysts for efficient overall water splitting, Coord. Chem. Rev., 2020, 418, 213374 CrossRef CAS
.
- C. Wang, L. Jin, H. Shang, H. Xu, Y. Shiraishi and Y. Du, Advances in engineering RuO2 electrocatalysts towards oxygen evolution reaction, Chin. Chem. Lett., 2021, 32, 2108–2116 CrossRef CAS
.
- G. Barati Darband, M. Aliofkhazraei, S. Hyun, A. Sabour Rouhaghdam and S. Shanmugam, Electrodeposition of Ni-Co-Fe mixed sulfide ultrathin nanosheets on Ni nanocones: a low-cost, durable and high performance catalyst for electrochemical water splitting, Nanoscale, 2019, 11, 16621–16634 RSC
.
- C. Wang, H. Xu, Y. Wang, H. Shang, L. Jin, F. Ren, T. Song, J. Guo and Y. Du, Hollow V-doped CoMx (M = P, S, O) nanoboxes as efficient OER electrocatalysts for overall water splitting, Inorg. Chem., 2020, 59, 11814–11822 CrossRef CAS PubMed
.
- W. J. Jiang, T. Tang, Y. Zhang and J. S. Hu, Synergistic modulation of non-precious-metal electrocatalysts for advanced water splitting, Acc. Chem. Res., 2020, 53, 1111–1123 CrossRef CAS PubMed
.
|
This journal is © the Partner Organisations 2022 |