DOI:
10.1039/D1QI01610E
(Research Article)
Inorg. Chem. Front., 2022,
9, 1134-1142
Porosity regulation of metal–organic frameworks for high proton conductivity by rational ligand design: mono- versus disulfonyl-4,4′-biphenyldicarboxylic acid†
Received
24th December 2021
, Accepted 28th January 2022
First published on 28th January 2022
Abstract
Porous crystalline metal–organic frameworks (MOFs) bearing sulfonic groups (–SO3H) are receiving increasing attention as solid-state proton conductors because the –SO3H group can not only enhance the proton concentration, but also form hydrogen bonding networks for high proton conductivity. A large number of 1,4-phenyldicarboxylic acids or biphenyldicarboxylic acids bearing two –SO3H groups have been applied for the synthesis of proton-conducting MOFs. Surprisingly, 4,4′-biphenyldicarboxylic acid bearing one –SO3H group has never been explored for the construction of proton-conducting materials. Herein, we first designed and synthesized 2-sulfonyl-4,4′-biphenyldicarboxylic acid (H3L). By applying this ligand to react with lanthanide salts, a series of three-dimensional MOFs, (Me2NH2)2(H3O)[LnL2]·8H2O (Ln = Eu (1), Gd (2), Tb (3)) have been prepared. Due to the presence of the uncoordinated –SO3H group and the encapsulation of high concentrations of dimethylammonium and hydronium cations in the cavity, the MOFs 1–3 show a high proton conductivity (8.83 × 10−3 S cm−1) at 95 °C and 60% relative humidity (RH). More importantly, this high proton conductivity can be maintained over 72 hours without any significant decrease at low RH.
1. Introduction
Solid-state proton conductors (SSPCs) as important components of fuel cells, electrochemical sensors and reactors are attracting great interest.1,2 Compared with traditional materials such as Nafion, porous crystalline MOF materials are considered to be one of the most promising next generation conductors as the crystal structures can be finely tuned by judicious selection of metal ions, ligands and appropriate post-synthesis modifications.3,4 During the past few decades, significant progress in proton conductive MOFs has been achieved with the conductivity already exceeding 10−2 S cm−1.5–7 However, to maintain the conductivity at these high levels, the conductors must remain in a high relative humidity (RH) environment (>90% RH), which poses significant challenges, including the energy expenditure associated with maintaining the high humidity and the loss of fuel cell performance due to the possible flooding of the cathode.8,9 Therefore, the development of novel SSPCs that maintain high proton conductivity at low RH is an urgent issue. There are usually two representative solutions: the first approach is to introduce small protic molecules into the cavity of MOFs, such as phosphonic acids,10,11 imidazoles12 and ionic liquids13–15 and the second approach involves the functionalization of ligands with hydrophilic groups for an enhanced binding ability of the resulting MOFs to water molecules.16,17
Sulfonated species are highly hydrophilic and those bearing free –SO3H groups are strong acids with a relatively low pKa value (generally less than 0).18 Consequently, sulfonated materials can interact with water molecules forming proton clusters in the form of –SO3−⋯H3O+ and significantly increase the proton conductivity in a low RH environment.19 Several methods for anchoring the acidic sulfonic groups to MOF pores have been developed including sulfonation of MOFs via post-synthesis,20–22 sulfonation of organic linkers,23 and coordination of sulfonic acid to metal centres.24,25 Unfortunately, the modified MOFs are seldom examined by single crystal X-ray diffraction analysis; as a result, an in depth understanding of the precise H-bonding networks and proton conducting pathway is difficult. Sulfonic group substituted carboxylic ligands can be regarded as appealing candidates for the construction of proton conductive MOFs and some MOFs have also been characterized by single crystal X-ray diffraction analysis.19,26 Commonly used ligands for the preparation of proton conductive MOFs include dipotassium-3,3′-disulfonyl-4,4′-biphenyldicarboxylic acid (3,3′-DSBPDC),27 3,3′-disulfonyl-benzophenone-4,4′-dicarboxylic acid (DSBODC),28 3,3′-disulfonyl-diphenylsulfone-4,4′-dicarboxylic acid (DSDPSDC),29 disodium 2,2′-disulfonate-4,4′-oxydibenzoic acid (DSOA),30–32 and 2,2′-disulfonyl-4,4′-biphenyldicarboxylic acid (2,2′-DSBPDC).33,34 All these ligands have two sulfonyl groups, which can be easily deprotonated to bind metal centers due to the stronger chelating ability of –SO3− groups to form cyclic coordinated configurations with neighboring –CO2− or –SO3− groups (Scheme 1).19,35 The coordination of the –SO3− group to metal centers will reduce the proton concentration and the porosity of the resulting MOFs, so that the proton conductivity may be greatly decreased.28
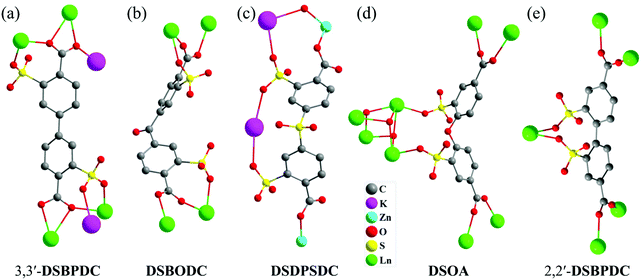 |
| Scheme 1 The coordination modes of some disulfonyl group substituted carboxylic ligands. | |
Our previous work also demonstrated that the MOF (Me2NH2)[Eu(2,2′-DSBPDC)(H2O)] based on a 2,2′-DSBPDC ligand showed a relatively low proton conductivity (4.14 × 10−8 S cm−1) at 25 °C and 95% RH owing to the coordination of two deprotonated sulfonyl groups to the metal center (Scheme 2).33 Therefore, our present strategy for preparing proton conductive MOFs is to design a mono-sulfonated substituted carboxylic ligand: 2-sulfonyl-4,4′-biphenyldicarboxylic acid (H3L) as shown in Scheme 2. We expect that the coordination of the –SO3H group of H3L to metal ions can be avoided so that the group may be completely free in the form of –SO3− or acidic –SO3H. Surprisingly, this kind of seemingly simple ligand is very elusive in the literature and has never been applied for the construction of MOFs and related proton-conducting materials to date.36 Compared to the disulfonyl-substituted one, the mono-sulfonated ligand with only one –SO3H group will possess less steric hindrance and the resulting MOFs can host more protic cations such as dimethylammonium or hydronium, due to the larger porosity.37–39
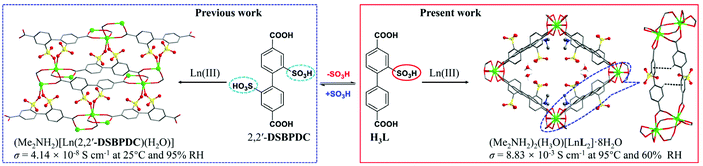 |
| Scheme 2 The comparison of the MOFs built from 2,2′-DSBPDC and H3L. | |
In order to demonstrate our conceptual approach, herein we first synthesized the H3L ligand using a Suzuki C–C coupling reaction between 4-iodo-3-sulfobenzoic acid and 4-carboxyphenylboronic acid (Scheme 3). Using the H3L ligand to react with lanthanide salts, a series of three-dimensional (3D) MOFs, (Me2NH2)2(H3O)[LnL2]·8H2O (Ln = Eu (1), Gd (2), Tb (3)) have been constructed. Single crystal X-ray diffraction analysis revealed that 1–3 all adopted the doubly interpenetrated 3D dia topology. Interestingly, due to the presence of a high concentration of dimethylammonium and, hydronium cations and hanging sulfonic acid groups within the 1D rhombic pore, 1–3 display a high proton conductivity (8.83 × 10−3 S cm−1) at 95 °C and 60% RH (Scheme 2). More importantly, this ultra-high proton conductivity can be maintained over 72 hours without any significant decrease. This work highlights the fact that mono-sulfonated carboxylic acids are a class of attractive ligands for the construction of proton conductive MOF materials.
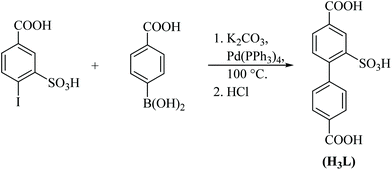 |
| Scheme 3 Synthesis of 2-sulfonyl-4,4′-biphenyldicarboxylic acid (H3L). | |
2. Experimental
2.1 Materials and methods
All chemical reagents were purchased and used as received. 4-Iodo-3-sulfobenzoic acid was synthesized according to the reference protocols.33,341H NMR spectra were recorded on a Bruker AM 400 MHz spectrometer in DMSO-d6 solution, and the chemical shifts are in ppm. FT-IR spectra were recorded on a Nicolet 380 FT-IR instrument (KBr pellets). Powder X-ray diffraction (PXRD) data were collected on a Bruker D8 Advance diffractometer under Cu Kα radiation (λ = 1.5406 Å). C, H, N, and S analyses were conducted using a Thermo Finnigan Flash 1112A elemental analyzer. Thermogravimetric analyses (TGA) were performed on a NETZSCH STA 449C thermal analyzer under a N2 atmosphere with the heating rate of 5 °C min−1.
2.2 Syntheses of the H3L ligand and MOFs 1–3
2.2.1 Synthesis of 2-sulfonyl-4,4′-biphenyldicarboxylic acid (H3L).
4-Iodo-3-sulfobenzoic acid (7.7600 g, 0.0216 mol), 4-carboxyphenylboronic acid (3.4866 g, 0.0216 mol), potassium carbonate (4.9691 g, 0.0360 mol), tetrakis(triphenylphosphine)palladium(0) (830.0 mg, 0.720 mmol), 1,4-dioxane and water (40 mL, 1
:
1) were mixed together in a three-neck flask under N2. The mixture was heated to 100 °C for 24 hours. After cooling to room temperature (RT), the resulting precipitate was filtered and washed with water (3 × 10 mL). The combined filtrate was concentrated to 20 mL and acidified with hydrochloric acid (36% w/w, 5 mL). The white solid was filtered and washed with water (3 × 10 mL) and acetonitrile (3 × 10 mL) and then dried in a vacuum to give the product H3L. Yield 4.2933 g (61.7%). 1H NMR (400 MHz, DMSO-d6, δ ppm): 12.95 (2H, COOH), 8.55 (1H, ArH), 7.93 (1H, ArH), 7.88–7.91 (2H, ArH), 7.67 (2H, ArH), 7.28 (1H, ArH). mp >300 °C. FT-IR (KBr, cm−1): 3478(br, m), 3119(m), 1713(vs), 1605(m), 1404(m), 1225(s), 1189(s), 1041(m), 852(w), 623(m). Anal. calcd for C14H10O7S (%): C 52.17, H 3.13, S 9.95; found: C 52.01, H 3.28, S 9.85.
The H3L ligand is insoluble in common solvents, such as water, methanol, ethanol, acetone, acetonitrile, dichloromethane and chloroform. It is only soluble in N,N-dimethylformamide (DMF) and dimethyl sulfoxide.
2.2.2 Synthesis of (Me2NH2)2(H3O)[EuL2]·8H2O (1).
A mixture of Eu(NO3)3·6H2O (8.9 mg, 0.02 mmol), H3L (15.1 mg, 0.04 mmol), dimethylamine hydrochloride (Me2NH·HCl) (20.0 mg, 0.25 mmol), and DMF (4 mL) was heated in a 25 mL stainless-steel reactor lined with Teflon at 150 °C for 48 h and then cooled to RT within 24 h. Colorless block-shaped crystals of 1 were collected by filtration and washed with ethanol (3 × 5 mL). The yield was 81.0% (16.9 mg) based on Eu(III). FT-IR (KBr, cm−1): 3345(br, m), 1585(m), 1408(vs), 1225(m), 1184(m), 1041(m), 630(m). Anal. calcd for C32H49EuN2O23S2 (%): C 36.75, H 4.72, N 2.68, S 6.13; found: C 36.65, H 4.59, N 2.54, S 6.02.
2.2.3 Synthesis of (Me2NH2)2(H3O)[GdL2]·8H2O (2).
The procedure was the same as that for 1 except for using Gd(NO3)3·6H2O (9.0 mg, 0.02 mmol) instead of Eu(NO3)3·6H2O. The yield of 2 was 83.4% (17.5 mg) based on Gd(III). FT-IR (KBr, cm−1): 3445(m), 1578(m), 1401(vs), 1225(m), 1190(m), 1041(s), 630(m). Anal. calcd for C32H49GdN2O23S2 (%): C 36.57, H 4.70, N 2.67, S 6.10; found: C 36.39, H 4.51, N 2.50, S 6.01.
2.2.4 Synthesis of (Me2NH2)2(H3O)[TbL2]·8H2O (3).
The procedure was the same as that for 1 except for using Tb(NO3)3·6H2O (9.1 mg, 0.02 mmol) instead of Eu(NO3)3·6H2O. The yield of 3 was 80.0% (16.8 mg) based on Tb(III). FT-IR (KBr, cm−1): 3421(m), 1588(m), 1420(vs), 1229(m), 1180(m), 1040(s). Anal. calcd for C32H49TbN2O23S2 (%): C 36.51, H 4.69, N 2.66, S 6.09; found: C 36.37, H 4.57, N 2.51, S 6.18.
2.3 Single crystal X-ray diffraction analysis
Single-crystal X-ray diffraction data for 1–3 were recorded on a Bruker Apex II CCD with a Mo Kα X-ray source (λ = 0.71073 Å) at RT. These structures were determined via direct methods with the SHELXTL-2018 software package.40 The crystallographic data of 1–3 are presented in Table S1.† The main bond distances and angles are summarized in Table S2.† These data (CCDC 2054977–2054979 for 1–3,† respectively) are available.
2.4 Proton conductivity measurement
The alternating current (AC) conductivity measurements for 2 were performed on a pressed cuboid-shaped plate (8 × 8 × 0.80 mm) made under a pressure of ∼15 MPa. The two faces of the plate were coated with silver paste and then the plates were pressed between parallel square titanium electrodes in specially designed porous quartz cells. The AC impedance data were obtained under different environmental conditions by an ordinary quasi-four-probe method, making use of silver wires and paste with a CHI 660E electrochemical workstation in the frequency range of 1 MHz–0.1 Hz with an input voltage amplitude of 100 mV. The temperature and humidity parameters were controlled using the SW/HS-50A temperature and humidity control chamber. The Zview software was used to determine the resistance value from the equivalent circuit fit of the first semi-circle.
Proton conductivity was calculated using the following equation:
|  | (1) |
where
l and
S are the length (cm) and cross-sectional area (cm
2) of the pressed plate respectively, and
R, which was extracted from the Nyquist plots, is the bulk resistance of the sample (Ω). Activation energy (
Ea) for the conductivity of materials was estimated from the following equation:
| 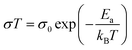 | (2) |
where
σ is the proton conductivity,
σ0 is the preexponential factor,
kB is the Boltzmann constant, and
T is the temperature.
3. Results and discussion
Although the H3L ligand was first mentioned in a US patent in 2012,36 its spectral characterization, yield and related complexes were not reported. Using a Suzuki C–C coupling reaction between 4-iodo-3-sulfobenzoic acid and 4-carboxyphenylboronic acid (Scheme 3), the H3L ligand was successfully prepared in a good yield and characterized by elemental analysis, FT-IR (Fig. S1†) and 1H NMR (Fig. S2†).
The solvothermal reaction of the H3L ligand with lanthanide salts Ln(NO3)3·6H2O (Ln = Eu, Gd, Tb) and Me2NH·HCl in DMF gave the corresponding MOFs 1–3 in high yields. Single-crystal X-ray diffraction analysis shows that all the MOFs 1–3 are isomorphous and crystallize in the same orthorhombic system with a space group of Pnnn (Table S1†). Due to the structural similarity of 1–3, only the structure of 2 is discussed here in detail. The asymmetric unit of 2 contains a Gd(III) cation (the occupancy factor is 0.5), one L3− ligand, one (Me2NH2)+ cation, one H3O+ cation (the occupancy factor is 0.5), and four highly disordered lattice water molecules (Fig. S4†). The presence of the counterions and guest water molecules has been further confirmed by elemental analysis, FT-IR (Fig. S7†) and TGA measurements (Fig. S13†). The Gd(III) center is coordinated by eight carboxylic O atoms from six different L3− ligands. Among the eight O atoms, four O atoms are from two chelating carboxylic groups, while the others are from four bridging carboxylic groups (Fig. 1d). The GdO8 unit exhibits a distorted dodecahedral geometry (Fig. 1a). The Gd–O distances (2.311(4)–2.485(4) Å) are comparable to those usually found in the related Gd-MOFs (Table S2†).41 Each Gd(III) ion is linked to the nearby one through four bridging carboxylic groups to form a [Gd(–COO)4Gd]2+ dimer with a Gd⋯Gd distance of 4.195(4) Å (Fig. 1d). Each dimer is further joined to four adjacent ones by a pair of L3− ligands in a head to tail connection mode (Fig. 1c), which can be regarded as a double-walled linker (Fig. 1d). Notably, the double-walled linker is further supported by two intermolecular edge-to-face C6–H6⋯πv interactions with a distance of 3.943(2) Å and an angle ∠C6–H6⋯πv of 134° between the phenyl rings (Fig. 1c and Table S3†). Therefore, by means of the double-walled linkers connecting to the dimers, a stable 3D framework in 2 can be finally formed (Fig. 1e and f). Topologically, if each double-walled linker is simplified as a straight line (Fig. 1c) and each [Gd(–COO)4Gd]2+ dimer is regarded as a 4-connected node (Fig. 1d), the 3D framework of 2 can be simplified as a dia net topology (Schläfli symbol of 66) as shown in Fig. 1e. The side length of the dia net is 15.831(13) Å, whereas the angles are 114.9(1)° and 127.0(1)°, respectively (Fig. 1e), exhibiting an obvious distortion from the tetrahedral angle of 109.5° observed in an ideal diamond. This distortion may be partially resulted from the two-fold interpenetrated structure found in 2 (Fig. 1e). Moreover, despite the double interpenetration, 2 still has a 57.2% solvent accessible volume calculated by PLATON software42 and possesses 1D rhombic channels along the a axis (Fig. 1f). These channels are filled with (Me2NH2)+ cations, H3O+ cations and lattice water molecules, which form extensive hydrogen-bonded networks with the –SO3− groups hanging on the pore walls (Fig. 1g). In addition, there are strong aliphatic C–H⋯O interactions between the methyl groups of the (Me2NH2)+ cation and the carboxylic O atoms in 2 (Table S3,† C15–H15C⋯O4), which is also manifested by a weak peak at 2800 cm−1 in the FT-IR spectrum of 2 (Fig. S7†).43,44 These abundant hydrogen-bonded networks can be recognized as an important platform for the proton transfer and motion, which will be beneficial for the proton conduction in 2.
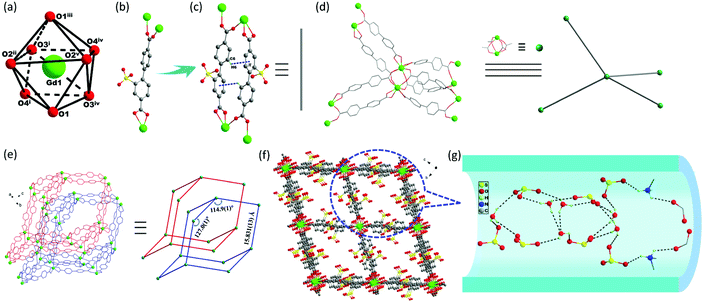 |
| Fig. 1 (a) A distorted dodecahedral geometry of the Gd1 atom. (b) The coordinated modes of L3− ligands. (c) A pair of L3− ligands in a head to tail connection mode supported by two C–H⋯π interactions. (d) A Gd2 dimer connected by four double-walled linkers was simplified as a 4-connected node. H atoms and sulfonic groups are omitted for clarity. (e) A doubly interpenetrated 3D dia net in 2. H atoms and sulfonic groups are omitted for clarity. (f) The 3D structure showing 1D rhombic pores with the sulfonic groups along the a axis. (g) The H-bonding network consisting of dimethylammonium, hydronium, and sulfonic ions in the 1D pore. | |
Since the mono-sulfonated H3L ligand is closely related to the disulfonated ligand 2,2′-DSBPDC,33 it would be interesting to compare the structures of the resulting MOFs derived from the same metal ions but differ only in the sulfonate substituents. Interestingly, in the channels of (Me2NH2)[Eu(2,2′-DSBPDC)(H2O)],33 only one dimethylammonium cation per metal unit can be hosted. In contrast, by eliminating one sulfonate group, the present MOF 2 can encapsulate two dimethylammonium cations as the volume of the pores in 2 is enlarged by the removal of one SO3− group. Most importantly, eight H2O molecules and an additional H3O+ cation per metal unit have also been included in 2 compared with (Me2NH2)[Eu(2,2′-DSBPDC)(H2O)] (only one coordinated water molecule per metal unit).33 In addition, the two deprotonated sulfonic groups in 2,2′-DSBPDC are bound to the same Eu(III) ion in a monodentate mode, forming a nine-membered ring in (Me2NH2)[Eu(2,2′-DSBPDC)(H2O)] (Scheme 1e).33 Consequently, the pores have only 16.7% solvent accessible volume by PLATON analysis.42 In contrast, the mono sulfonic group of H3L is not involved in the coordination with metal ions, giving 57.2% solvent accessible volume in 2. Notably, when the same synthesis conditions as those for (Me2NH2)[Eu(2,2′-DSBPDC)(H2O)] were used except for replacing 2,2′-DSBPDC with H3L, only an unidentified amorphous powder was obtained.
The high concentration of dimethylammonium and, hydronium cations and abundant hydrogen-bonded networks from the hanging sulfonic acid groups within the 1D rhombic pores of 2 encouraged us to investigate the proton conductivity (σ). The Nyquist plots of 2 are shown in Fig. 2. First, the σ value of 2 was evaluated under different RH at RT (Fig. 2a). At 30% RH, the σ value of 2 was 1.06 × 10−5 S cm−1, while at 60% RH the value increased to 2.35 × 10−4 S cm−1 (Table S4†). However, the effective data points failed to be taken because the samples of 2 cannot be equilibrated above 60% RH. Thus, the variable temperature conductivity of 2 was measured from 35 to 95 °C at 60% RH for the same sample. At 60% RH, the σ value (3.80 × 10−4 S cm−1) of 2 at 35 °C increased rapidly to 8.83 × 10−3 S cm−1 at 95 °C (Fig. 2b and Table S4†), making 2 a super proton conductive MOF material under low humidity conditions (<70% RH) (Table 1). The σ value of 2 at 95 °C and 60% RH is only slightly lower than those reported for two strong acid directly doped MOFs, H2SO4@MIL-101 (6.0 × 10−2 S cm−1)45 and CF3SO3H@MIL-101 (5 × 10−2 S cm−1) (Table 1).46 The high proton conductivity of 2 under such low humidity conditions may be attributed to the incorporation of hydrophilic sulfonic groups for enhanced binding capacity to the water molecules that are encapsulated inside the MOF channels. Moreover, the high proton conductivity of 2 can be maintained over 72 hours without any significant decrease (Fig. 2d). The activation energy (Ea) of 2 was calculated from the Arrhenius plot (Fig. 2c) to be 0.52 eV, suggesting a conventional vehicle mechanism.47
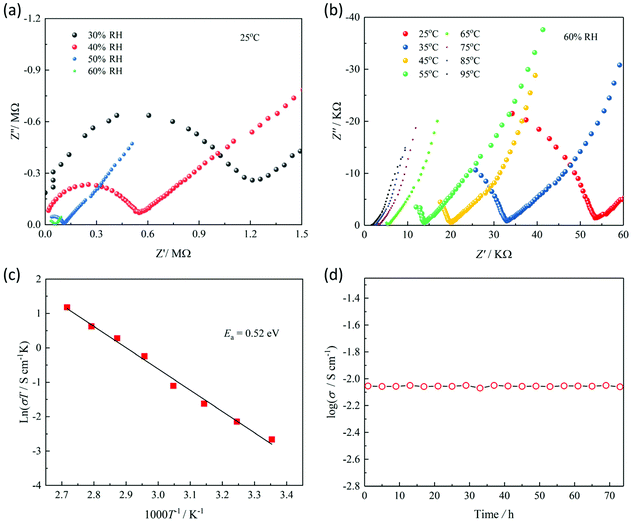 |
| Fig. 2 (a) Impedance spectra of 2 under 30–60% RH at RT. (b) The variable temperature impedance spectra of 2 from 25 to 95 °C at 60% RH. (c) Arrhenius plot. (f). Time-dependent proton conductivity of 2 at 95 °C and 60% RH. | |
Table 1 Proton conductivities of some representative MOFs at low humidity (<70% RH)
MOFs |
σ (S cm−1) |
Conditions |
Ref. |
H2SO4@MIL-101 |
6.0 × 10−2 |
80 °C/20% RH |
45
|
CF3SO3H@MIL-101 |
5 × 10−2 |
25 °C/15% RH |
46
|
IL@MIL-101(SIB-3) |
4.4 × 10−2 |
50 °C/23% RH |
48
|
VNU-15 |
2.9 × 10−2 |
95 °C/60% RH |
17
|
SPEEK/S-UiO-66@GO-10 |
1.66 × 10−2 |
100 °C/40% RH |
49
|
(Me2NH2)2(H3O)[LnL2]·8H2O |
8.83 × 10−3 |
95 °C/60% RH |
This work |
PSM-2 |
4.6 × 10−3 |
80 °C/40% RH |
50
|
{(Me2NH2)2(H2O)3[Ln2L2]}n |
1.1 × 10−3 |
100 °C/68% RH |
51
|
PCMOF-17 |
1.17 × 10−3 |
25 °C/40% RH |
16
|
PCMOF2(Pz) |
3.28 × 10−4 |
25 °C/40% RH |
52
|
[{(tmen)Pd}7(tib)2(ptp)2](NO3)14 |
6.56 × 10−4 |
23 °C/46% RH |
53
|
[Zn(H5-sip)(4,4′-bpy)]·DMF·2H2O |
3.9 × 10−4 |
25 °C/60% RH |
54
|
[Zn(H2O)(H5-sip)(bpel)0.5]·DMF |
3.4 × 10−8 |
25 °C/60% RH |
54
|
[Zn3(5-sip)2(H5-sip)(4,4′-bpy)]·DMF·2DMA |
8.7 × 10−5 |
25 °C/60% RH |
54
|
{[Co3(mClPhIDC)2(H2O)6]·2H2O}n |
8.69 × 10−5 |
100 °C/68% RH |
55
|
{R3N(CH2CO2H)}[MCr(ox)3]·nH2O |
8 × 10−5 |
25 °C/60% RH |
56
|
NH2-MIL-53 |
3 × 10−5 |
80 °C/26% RH |
57
|
At RT, the σ value (2.35 × 10−4 S cm−1) of 2 under low humidity conditions (60% RH) is much higher than that (4.14 × 10−8 S cm−1) of the MOF (Me2NH2)[Eu(2,2′-DSBPDC)(H2O)] at high humidity (95% RH).33 At an elevated temperature, the σ value (8.83 × 10−3 S cm−1 at 95 °C and 60% RH) of 2 is also higher than those found for the disulfonyl-based MOFs at a high RH, JXNU-2 with 3,3′-DSBPDC (1.11 × 10−3 S cm−1 at 80 °C and 98% RH),27 {(H3O)[Ln(DSBODC)(H2O)2]}n (6.57 × 10−4 S cm−1 at 85 °C and 95% RH),28 [K2Zn(DSDPSDC)(H2O)4]n, (1.57 × 10−4 S cm−1 at 85 °C and 95% RH),29 and {[Tb4(OH)4(DSOA)2(H2O)8]·8H2O}n, (1.66 × 10−4 S cm−1 at 100 °C and 98% RH).31 The proton conductivity study of 2 once again confirms the importance of the hanging sulfonic acid groups in the pores of the MOFs in the formation of the proton-mobilizing pathway. By analyzing the structure of 2 and also examining the species encapsulated inside the channels, it is reasonable to assume that the high proton conductivity of 2 at low RH is due to the strong H-bond interactions between the sulfonic groups, the dimethylammonium and hydronium cations. The structural integrity of 2 was further confirmed by the PXRD analysis of the samples before and after the measurements, which show the same patterns (Fig. S10†). It is to note that, unfortunately, MOF 2 cannot maintain the porosity after three days of water immersion (Fig. S11†). The reduced stability of 2 in aqueous media compared to the MOFs from disulfonated ligands is probably due to the enhanced acidity of the MOF caused by the presence of uncoordinated SO3− groups that are more reactive upon water immersion. Similar phenomena have been observed previously.16 Further studies to improve the water stability are in progress and will be reported in due course.
4. Conclusion
In conclusion, we have successfully developed a concept for porosity regulation of MOFs through rational ligand design. By applying asymmetric, mono-sulfonated 2-sulfonyl-4,4′-biphenyldicarboxylic acid as a novel ligand, a series of 3D MOFs containing hanging sulfonic groups have been synthesized and characterized by X-ray crystallography. Due to the elimination of one sulfonate group, coordination of the sulfonate group to metal ions can be avoided and the resulting MOFs have increased porosity and can encapsulate more protic ions. Consequently, an increased proton conductivity (up to 8.83 × 10−3 S cm−1 at 95 °C and 60% RH) has been achieved. Furthermore, this high proton conductivity can be maintained over 72 hours without any significant decrease. We believe that this study will unlock an enormous opportunity in ligand design, especially in the area of asymmetric carboxylic acid based ligands and related MOFs. It is to expect that the strategy of regulating the porosity of MOFs by ligand design will be extended in other areas.
Conflicts of interest
There are no conflicts to declare.
Acknowledgements
This work was supported by the National Natural Science Foundation of China (No. 21476115) and the Natural Science Foundation of Jiangsu Province (No. BK20181374). S. Z. thanks the Postgraduate Research & Practice Innovation Program of Jiangsu Province (KYCX20_1027) and the Cultivation Program for the Excellent Doctoral Dissertation of Nanjing Tech University (2020-03) for financial support.
References
- M. Pan, C. Pan, C. Li and J. Zhao, A review of membranes in proton exchange membrane fuel cells: Transport phenomena, performance and durability, Renewable Sustainable Energy Rev., 2021, 141, 110771 CrossRef CAS.
- X. Meng, H. N. Wang, S. Y. Song and H. J. Zhang, Proton-conducting crystalline porous materials, Chem. Soc. Rev., 2017, 46, 464–480 RSC.
- D.-W. Lim and H. Kitagawa, Proton transport in metal-organic frameworks, Chem. Rev., 2020, 120, 8416–8467 CrossRef CAS PubMed.
- D.-W. Lim, M. Sadakiyo and H. Kitagawa, Proton transfer in hydrogen-bonded degenerate systems of water and ammonia in metal-organic frameworks, Chem. Sci., 2019, 10, 16–33 RSC.
- S. Chand, S. M. Elahi, A. Pal and M. C. Das, Metal-organic frameworks and other crystalline materials for ultrahigh superprotonic conductivities of 10−2 S cm−1 or higher, Chem. – Eur. J., 2019, 25, 6259–6269 CrossRef CAS PubMed.
- S. C. Pal and M. C. Das, Superprotonic conductivity of MOFs and other crystalline platforms beyond 10–1 S cm−1, Adv. Funct. Mater., 2021, 31, 2101584 CrossRef CAS.
- M. K. Sarango-Ramírez, J. Park, J. Kim, Y. Yoshida, D.-W. Lim and H. Kitagawa, Void space versus surface functionalization for proton conduction in metal-organic frameworks, Angew. Chem., Int. Ed., 2021, 60, 20173–20177 CrossRef PubMed.
- Q. Li, R. He, J. O. Jensen and N. J. Bjerrum, Approaches and recent development of polymer electrolyte membranes for fuel cells operating above 100 °C, Chem. Mater., 2003, 15, 4896–4915 CrossRef CAS.
- Y. Chen, M. Thorn, S. Christensen, C. Versek, A. Poe, R. C. Hayward, M. T. Tuominen and S. Thayumanavan, Enhancement of anhydrous proton transport by supramolecular nanochannels in comb polymers, Nat. Chem., 2010, 2, 503–508 CrossRef CAS PubMed.
- Z. Li, G. He, B. Zhang, Y. Cao, H. Wu, Z. Jiang and T. Zhou, Enhanced proton conductivity of Nafion hybrid membrane under different humidities by incorporating metal-organic frameworks with high phytic acid loading, ACS Appl. Mater. Interfaces, 2014, 6, 9799–9807 CrossRef CAS PubMed.
- V. G. Ponomareva, A. M. Cheplakova, K. A. Kovalenko and V. P. Fedin, Exceptionally stable H3PO4@MIL-100 system: A correlation between proton conduction and water adsorption properties, J. Phys. Chem. C, 2020, 124, 23143–23149 CrossRef CAS.
- S. Liu, Z. Yue and Y. Liu, Incorporation of imidazole within the metal-organic framework UiO-67 for enhanced anhydrous proton conductivity, Dalton Trans., 2015, 44, 12976–12980 RSC.
- X. L. Sun, W. H. Deng, H. Chen, H. L. Han, J. M. Taylor, C. Q. Wan and G. Xu, A metal-organic framework impregnated with a binary ionic liquid for safe proton conduction above 100 °C, Chem. – Eur. J., 2017, 23, 1248–1252 CrossRef CAS PubMed.
- W. J. Liu, L. Z. Dong, R. H. Li, Y. J. Chen, S. N. Sun, S. L. Li and Y. Q. Lan, Different protonic species affecting proton conductivity in hollow spherelike polyoxometalates, ACS Appl. Mater. Interfaces, 2019, 11, 7030–7036 CrossRef CAS PubMed.
- P. Chen, S. Liu, Z. Bai and Y. Liu, Enhanced ionic conductivity of ionic liquid confined in UiO-67 membrane at low humidity, Microporous Mesoporous Mater., 2020, 305, 110369 CrossRef CAS.
- B. Joarder, J. B. Lin, Z. Romero and G. K. H. Shimizu, Single crystal proton conduction study of a metal organic framework of modest water stability, J. Am. Chem. Soc., 2017, 139, 7176–7179 CrossRef CAS PubMed.
- T. N. Tu, N. Q. Phan, T. T. Vu, H. L. Nguyen, K. E. Cordova and H. Furukawa, High proton conductivity at low relative humidity in an anionic Fe-based metal-organic framework, J. Mater. Chem. A, 2016, 4, 3638–3641 RSC.
- J. Jiang and O. M. Yaghi, Brønsted acidity in metal-organic frameworks, Chem. Rev., 2015, 115, 6966–6997 CrossRef CAS PubMed.
- R.-L. Liu, D.-Y. Wang, J.-R. Shi and G. Li, Proton conductive metal sulfonate frameworks, Coord. Chem. Rev., 2021, 431, 213747 CrossRef CAS.
- S. Mukhopadhyay, J. Debgupta, C. Singh, R. Sarkar, O. Basu and S. K. Das, Designing UiO-66-based superprotonic conductor with the highest metal-organic framework based proton conductivity, ACS Appl. Mater. Interfaces, 2019, 11, 13423–13432 CrossRef CAS PubMed.
- S. Devautour-Vinot, E. S. Sanil, A. Geneste, V. Ortiz, P. G. Yot, J.-S. Chang and G. Maurin, Guest-assisted proton conduction in the sulfonic mesoporous MIL-101 MOF, Chem. – Asian J., 2019, 14, 3561–3565 CrossRef CAS PubMed.
- W. J. Phang, H. Jo, W. R. Lee, J. H. Song, K. Yoo, B. Kim and C. S. Hong, Superprotonic conductivity of a UiO-66 framework functionalized with sulfonic acid groups by facile postsynthetic oxidation, Angew. Chem., Int. Ed., 2015, 54, 5142–5146 CrossRef CAS PubMed.
- X.-M. Li, L.-Z. Dong, S.-L. Li, G. Xu, J. Liu, F.-M. Zhang, L.-S. Lu and Y.-Q. Lan, Synergistic conductivity effect in a proton sources-coupled metal-organic framework, ACS Energy Lett., 2017, 2, 2313–2318 CrossRef CAS.
- Y. Wang, G. Ye, H. Chen, X. Hu, Z. Niu and S. Ma, Functionalized metal-organic framework as a new platform for efficient and selective removal of cadmium(II) from aqueous solution, J. Mater. Chem. A, 2015, 3, 15292–15298 RSC.
- J. Jiang, F. Gandara, Y.-B. Zhang, K. Na, O. M. Yaghi and W. G. Klemperer, Superacidity in sulfated metal-organic framework-808, J. Am. Chem. Soc., 2014, 136, 12844–12847 CrossRef CAS PubMed.
- L. Zhao, R.-R. Zhu, S. Wang, L. He, L. Du and Q.-H. Zhao, Multiple strategies to fabricate a highly stable 2D CuIICuI-organic framework with high proton conductivity, Inorg. Chem., 2021, 21, 16474–16483 CrossRef PubMed.
- L. J. Zhou, W. H. Deng, Y. L. Wang, G. Xu, S. G. Yin and Q. Y. Liu, Lanthanide-potassium biphenyl-3,3′-disulfonyl-4,4′-dicarboxylate frameworks: gas sorption, proton conductivity, and luminescent sensing of metal ions, Inorg. Chem., 2016, 55, 6271–6277 CrossRef CAS PubMed.
- W. W. Zhang, Y. L. Wang, Q. Liu and Q. Y. Liu, Lanthanide-benzophenone-3,3′-disulfonyl-4,4′-dicarboxylate frameworks: Temperature and 1-hydroxypyren luminescence sensing and proton conduction, Inorg. Chem., 2018, 57, 7805–7814 CrossRef CAS PubMed.
- X.-Y. Zeng, Y.-L. Wang, Z.-T. Lin and Q.-Y. Liu, Proton-conductive coordination polymers based on diphenylsulfone-3,3′-disulfo-4,4′-dicarboxylate with well-defined hydrogen bonding networks, Inorg. Chem., 2020, 59, 12314–12321 CrossRef CAS PubMed.
- X.-Y. Dong, R. Wang, J.-B. Li, S.-Q. Zang, H.-W. Hou and T. C. W. Mak, A tetranuclear Cu4(μ3-OH)2-based metal-organic framework (MOF) with sulfonate-carboxylate ligands for proton conduction, Chem. Commun., 2013, 49, 10590–10592 RSC.
- X.-Y. Dong, R. Wang, J.-Z. Wang, S.-Q. Zang and T. C. W. Mak, Highly selective Fe3+ sensing and proton conduction in a water-stable sulfonate-carboxylate Tb-organic-framework, J. Mater. Chem. A, 2015, 3, 641–647 RSC.
- S. P. Bera, A. Mondal, S. Roy, B. Dey, A. Santra and S. Konar, 3D isomorphous lanthanide coordination polymers displaying magnetic refrigeration, slow magnetic relaxation and tunable proton conduction, Dalton Trans., 2018, 47, 15405–15415 RSC.
- J. Zhao, X. He, Y. Zhang, J. Zhu, X. Shen and D. Zhu, Highly water stable lanthanide metal-organic frameworks constructed from 2,2′-disulfonyl-4,4′-biphenyldicarboxylic acid: Syntheses, structures, and properties, Cryst. Growth Des., 2017, 17, 5524–5532 CrossRef CAS.
- S. Zhang, S. Gao, X. Wang, X. He, J. Zhao and D. Zhu, Two topologically different 3D CuII metal-organic frameworks assembled from the same ligands: control of reaction conditions, Acta Crystallogr., Sect. B: Struct. Sci., Cryst. Eng. Mater., 2019, 75, 1060–1068 CrossRef CAS PubMed.
- M. Furtmair, J. Timm and R. Marschall, Sulfonation of porous materials and their proton conductivity, Microporous Mesoporous Mater., 2021, 312, 110745 CrossRef CAS.
-
P. I. Lazarev, US PatentUS20120099052A1, 2012 Search PubMed.
- B. Joarder, J.-B. Lin, Z. Romero and G. K. H. Shimizu, Single crystal proton conduction study of a metal organic framework of modest water stability, J. Am. Chem. Soc., 2017, 139, 7176–7179 CrossRef CAS PubMed.
- T.-T. Guo, D.-M. Cheng, J. Yang, X. Xu and J.-F. Ma, Calix[4]resorcinarene-based [Co16] coordination cages mediated by isomorphous auxiliary ligands for enhanced proton conduction, Chem. Commun., 2019, 55, 6277–6280 RSC.
- F. Yang, G. Xu, Y. Dou, B. Wang, H. Zhang, H. Wu, W. Zhou, J.-R. Li and B. Chen, A flexible metal-organic framework with a high density of sulfonic acid sites for proton conduction, Nat. Energy, 2017, 2, 877–883 CrossRef CAS.
- G. Sheldrick, Crystal structure refinement with SHELXL, Acta Crystallogr., Sect. C: Struct. Chem., 2015, 71, 3–8 Search PubMed.
- X. Wang, J. Zhao, Y. Zhao, H. Xu, X. Shen, D. R. Zhu and S. Jing, Three sra topological lanthanide-organic frameworks built from 2,2′-dimethoxy-4,4′-biphenyldicarboxylic acid, Dalton Trans., 2015, 44, 9281–9288 RSC.
- A. Spek, Single-crystal structure validation with the program PLATON, J. Appl. Crystallogr., 2003, 36, 7–13 CrossRef CAS.
- A. Elaiwi, P. B. Hitchcock, K. R. Seddon, N. Srinivasan, Y.-M. Tan, T. Welton and J. A. Zora, Hydrogen bonding in imidazolium salts and its implications for ambient-temperature halogenoaluminate(III) ionic liquids, J. Chem. Soc., Dalton Trans., 1995, 21, 3467–3472 RSC.
- Z. Fei, D. Zhao, T. J. Geldbach, R. Scopelliti and P. J. Dyson, Brønsted acidic ionic liquids and their zwitterions: synthesis, characterization and pKa determination, Chem. – Eur. J., 2004, 10, 4886–4893 CrossRef CAS PubMed.
- V. G. Ponomareva, K. A. Kovalenko, A. P. Chupakhin, D. N. Dybtsev, E. S. Shutova and V. P. Fedin, Imparting high proton conductivity to a metal-organic framework material by controlled acid impregnation, J. Am. Chem. Soc., 2012, 134, 15640–15643 CrossRef CAS PubMed.
- D. N. Dybtsev, V. G. Ponomareva, S. B. Aliev, A. P. Chupakhin, M. R. Gallyamov, N. K. Moroz, B. A. Kolesov, K. A. Kovalenko, E. S. Shutova and V. P. Fedin, High proton conductivity and spectroscopic investigations of metal-organic framework materials impregnated by strong acids, ACS Appl. Mater. Interfaces, 2014, 6, 5161–5167 CrossRef CAS PubMed.
- X. Wang, T. Qin, S.-S. Bao, Y.-C. Zhang, X. Shen, L.-M. Zheng and D. Zhu, Facile synthesis of a water stable 3D Eu-MOF showing high proton conductivity and its application as a sensitive luminescent sensor for Cu2+ ions, J. Mater. Chem. A, 2016, 4, 16484–16489 RSC.
- J. Du, G. Yu, H. Lin, P. Jie, F. Zhang, F. Qu, C. Wen, L. Feng and X. Liang, Enhanced proton conductivity of metal organic framework at low humidity by improvement in water retention, J. Colloid Interface Sci., 2020, 573, 360–369 CrossRef CAS PubMed.
- H. Sun, B. Tang and P. Wu, Rational design of S-UiO-66@GO hybrid nanosheets for proton exchange membranes with significantly enhanced transport performance, ACS Appl. Mater. Interfaces, 2017, 9, 26077–26087 CrossRef CAS PubMed.
- S. Mukhopadhyay, J. Debgupta, C. Singh, R. Sarkar, O. Basu and S. K. Das, Designing UiO-66-based superprotonic conductor with the highest metal-organic framework based proton conductivity, ACS Appl. Mater. Interfaces, 2019, 11, 13423–13432 CrossRef CAS PubMed.
- R. Wang, X.-Y. Dong, H. Xu, R.-B. Pei, M.-L. Ma, S.-Q. Zang, H.-W. Hou and T. C. W. Mak, A super water-stable europium-organic framework: Guests inducing low-humidity proton conduction and sensing of metal ions, Chem. Commun., 2014, 50, 9153–9156 RSC.
- S. Kim, B. Joarder, J. A. Hurd, J. Zhang, K. W. Dawson, B. S. Gelfand, N. E. Wong and G. K. H. Shimizu, Achieving superprotonic conduction in metal-organic frameworks through iterative design advances, J. Am. Chem. Soc., 2018, 140, 1077–1082 CrossRef CAS PubMed.
- D. Samanta and P. S. Mukherjee, Structural diversity in multinuclear Pd(II) assemblies that show low-humidity proton conduction, Chem. – Eur. J., 2014, 20, 5649–5656 CrossRef CAS PubMed.
- P. Ramaswamy, R. Matsuda, W. Kosaka, G. Akiyama, H. Joon Jeon and S. Kitagawa, Highly proton conductive nanoporous coordination polymers with sulfonic acid groups on the pore surface, Chem. Commun., 2014, 50, 1144–1146 RSC.
- X. Liang, B. Li, M. Wang, J. Wang, R. Liu and G. Li, Effective approach to promoting the proton conductivity of metal-organic frameworks by exposure to aqua-ammonia vapor, ACS Appl. Mater. Interfaces, 2017, 9, 25082–25086 CrossRef CAS PubMed.
- M. Sadakiyo, H. Ōkawa, A. Shigematsu, M. Ohba, T. Yamada and H. Kitagawa, Promotion of low-humidity proton conduction by controlling hydrophilicity in layered metal-organic frameworks, J. Am. Chem. Soc., 2012, 134, 5472–5475 CrossRef CAS PubMed.
- J. Liu, X. Zou, C. Liu, K. Cai, N. Zhao, W. Zheng and G. Zhu, Ionothermal synthesis and proton-conductive properties of NH2-MIL-53 MOF nanomaterials, CrystEngComm, 2016, 18, 525–528 RSC.
Footnote |
† Electronic supplementary information (ESI) available: FT-IR and 1H NMR spectra of H3L, the molecular structures, FT-IR spectra, PXRD patterns, and TGA curves of 1–3, and the proton conductivity measurement of 2. CCDC 2054977–2054979 for 1–3. For ESI and crystallographic data in CIF or other electronic format see DOI: 10.1039/d1qi01610e |
|
This journal is © the Partner Organisations 2022 |