Construction of a highly efficient DNA nanotube sensor with peroxide-like activity†
Received
28th August 2023
, Accepted 13th November 2023
First published on 12th December 2023
Abstract
The G-quadruplex/heme complexes are special DNA-based artificial metalloenzymes with peroxidase-like activity and are widely used in biosensing and biocatalysis. However, their peroxidase-like activity is not satisfactory. Due to the high programmability and good stability of DNA, DNA as a scaffold material is promising for enhancing the activity of artificial metalloenzymes. In this work, an effective DNA nanotube-based peroxidase was constructed using a self-assembly strategy. To improve the activity of G-quadruplex/heme complexes, a new method for the construction of G-quadruplex/heme complex arrays was proposed in a simple and inexpensive way. By designing the toes of DNA nanotubes as G-quadruplexes, G-quadruplex arrays could be formed on pure DNA nanotubes, and then the G-quadruplex arrays bind to heme to form a nanotube-supported DNAzyme termed as DNTzyme. Agarose gel electrophoresis, circular dichroism, and fluorescence microscopy were used to characterize DNTzyme. What is more, because the loading of DNAzyme on DNA nanotubes can increase their biological stability, a hydrogen peroxide detection sensor was constructed using the enhanced enzymatic activity and excellent stability of DNTzyme. The sensor could accurately and efficiently detect peroxide and show enhanced fluorescence with a detection limit of 49 nM for H2O2 and 1.4 μM for TBHP, and a color development time of about 5 min. This sensor is expected to have applications in bio-detection, biocatalysis, and drug delivery.
1. Introduction
Conventional catalyst synthesis methods require large amounts of hazardous and toxic reagents, as well as dangerous reaction conditions such as high temperatures and pressures. These conditions result in low reaction efficiency, exorbitant cost, and severe pollution problems. The idea of biomimetic catalysis has been proposed to solve these issues. Biomimetic catalysis, which synthesizes artificial catalysts to achieve environmentally friendly, efficient, cost-effective, and highly selective reactions, mimics biological catalysis based on a profound comprehension of catalytic reactions in living organisms.1–3 Among them, artificial metalloenzymes are a significant subject of investigation in the biomimetic catalysis field. They have the catalytic efficiency and specificity of natural enzymes, as well as greater flexibility and controllability.4,5 In comparison to traditional catalysts, artificial metalloenzymes have the capability to catalyze reactions under mild conditions and offer superior application prospects.6 Nonetheless, artificial metalloenzymes present certain problems, including low activity, poor biocompatibility, etc. Therefore, building artificial metalloenzymes with biomolecules can solve these problems efficiently.6,7 At present, there are few reports on artificial metalloenzymes with nucleic acid as the backbone.
In recent years, the study of DNA-based biomimetic catalysts has received much attention because they can mimic the catalytic function of natural enzymes. These catalysts offer several advantages, including high efficiency, high selectivity, easy synthesis, and customizable structure.8 At present, scientists have proficiently employed DNA molecules to fabricate diverse biomimetic catalysts, including DNAzyme,9 DNA metal–organic frameworks,10 and DNA-based artificial metalloenzymes.8 DNA-based artificial metalloenzymes are promising artificial catalysts that combine the advantages of DNA and metal complex catalysts, and they have a wide range of applications in biomedical and chemical synthesis, etc. The DNA backbone has the advantages of high programmability, stability, and structural diversity.8 Moreover, the DNA groove is a unique structure that can elicit a distinctive influence on chemical reactions.11–13 Nevertheless, the accurate controlling of the binding sites of metal complexes to DNA is typically challenging, thereby restricting further research and development in this field.
DNA nanotubes are nanoscale tubes composed of DNA molecules with excellent mechanical properties, chemical stability, and biocompatibility. DNA nanotube preparation methods currently include rolled ring amplification13 and self-assembly.14 Of both these methods, the most prevalent method is self-assembly. DNA nanotubes with different morphologies and functions can be constructed by designing DNA sequences and assembly conditions using the self-assembly between DNA molecules.15,16 However, there are few reports on DNA nanotubes being used to construct artificial metalloenzymes for biomimetic catalysis. We speculate whether DNA nanotubes can be used to mimic enzymatic properties by non-covalent binding to metal complexes. The peroxidase activity of G-quadruplex/iron porphyrin complexes has received much attention.17 Although the G-quadruplex/iron porphyrin complex is expected to serve as an alternative to horseradish peroxidase (HRP) in many fields, its low catalytic activity greatly limits its further application. Achieving a more efficient catalytic system based on G-quadruplex/iron porphyrin complexes remains an important and valuable challenge.18 The G-quadruplex/heme complex is a specialized DNA-based artificial metalloenzyme in which heme binds to the G-quadruplex precisely and non-covalently.19 The G-quadruplex/heme complex is a promising model DNA-based mimetic catalyst, owing to the specific binding of heme to the G-quadruplex. The long-term goal of researchers is to figure out how to improve the peroxidase-like activity of the G-quadruplex/heme complex. However, no reports exist on constructing arrays of G-quadruplex/heme complexes on pure DNA nanotubes for enhancing their activities.
In this paper, an effective and highly active DNA nanotube-like peroxidase was constructed by using a self-assembly strategy. To enhance the activity of G-quadruplex/heme complexes, a novel approach was presented for constructing G-quadruplex/heme complex arrays in a convenient and inexpensive way. G-quadruplex arrays could be created on pure DNA nanotubes by designing the toes of DNA nanotubes as G-quadruplexes, and then the G-quadruplex arrays bind to heme to form G-quadruplex/heme complex arrays. DNTzyme was characterized using agarose gel electrophoresis, circular dichroism, and fluorescence microscopy. And a variety of factors affecting the peroxidase activity were explored to guide the design and optimization of the DNTzyme. Then, the stability of the DNTzyme was compared to that of HRP. Finally, the loading of DNAzyme on DNA nanotubes can increase their biological stability by increasing their resistance to biological enzymes,20,21 and we tried to apply it to the detection of peroxide and to achieve enhanced fluorescence. This study was anticipated to have implications for bioassays, biocatalyses, and drug delivery.
2. Experiments
2.1. Preparation of materials
2.1.1. Preparation of DNA nanotubes.
a. Preparation of blank DNA nanotubes.
Single-stranded DNA (ssDNA) used in the experiments was pre-quantified using a UV spectrophotometer at 260 nm. The total volume was set to 1 mL. Five ssDNAs from S1–S5 (1 or 5 μM each), MgCl2 (12.5 mM), and KCl (100 mM) were added to 1× TAE buffer. The system was heated to 90 °C in a PCR instrument and kept for 5 min, and then slowly cooled down to 4 °C at a rate of −0.1 °C min−1 to produce DNA nanotube samples. The DNA nanotubes were stored at 4 °C.
b. Preparation of DNA nanotubes loaded with G-quadruplex arrays.
Five ssDNAs S1–S5 (1 or 5 μM each), where the S2 was replaced with G4-S2, MgCl2 (12.5 mM), and KCl (100 mM) were added to 1× TAE buffer. The procedure discussed in subsection ‘a’ was repeated.
c. Preparation of fluorescently labelled DNA nanotubes.
Five ssDNAs S1–S5, where the S3 was replaced with fluorescently labelled FAM-S3, MgCl2 (12.5 mM), and KCl (100 mM) were added to 1× TAE buffer. The procedure discussed in subsection ‘a’ was repeated. The nanotubes were characterized by agarose gel electrophoresis.
2.1.2. G-quadruplex processing.
The total volume of the system was 1 mL. ssDNA S1, G4-S2, S3–S5 (1 or 5 μM), MgCl2 (12.5 mM), and KCl (100 mM) were added to 1× TAE buffer. The system was heated to 90 °C for 5 min in the PCR instrument, and then slowly cooled down to 4 °C at a speed of −0.1 °C min−1 to make a G-quadruplex sample. The G-quadruplex was stored at 4 °C.
2.1.3. Preparation of DNTzyme.
The prepared DNA nanotubes loaded with G-quadruplex arrays (2 μM) were mixed with hemin solution (3 μM, dissolved in 1% DMSO) and vortexed for 20 s. The system was then set at room temperature for 30 min to fully integrate the DNA nanotubes with hemin to obtain DNTzyme. The formation of DNTzyme was determined by UV titration.
2.2. Activity determination
The activity of DNTzyme was determined by measuring the efficiency of oxidation of ABTS by DNTzyme in the presence of H2O2. The total volume of the reaction system was set to 1 mL. The DNTzyme (0.5 μM), H2O2 (1 mM), and ABTS (1 mM) were added to buffer A (see Table S1 for details, ESI†). The solution was left at room temperature for 5 min. The absorbance change values were determined at 420 nm using a UV spectrophotometer after reacting the system for 5 min. The initial velocity of the reaction V0 was calculated based on the extinction coefficient of 36
000 M−1 cm−1. Three parallel sets of experiments were performed. Kinetic parameters were determined by varying the concentration of the H2O2 system (0–3 mM), and Km and Vmax were obtained by fitting the kinetic curves. Kcat was obtained using the formula:
2.3. Study on activity factors of DNTzyme
2.3.1. Array loading position.
A series of ssDNAs loaded with the G-quadruplex (denoted as G4-X) at the 3′ or 5′ end were designed and denoted as 3′S2-X or 5′S2-X, respectively (all DNA sequences are detailed in Table S2, ESI†). DNA nanotubes were synthesized using 3′S2-X and 5′S2-X instead of S2 and the corresponding IDNTzyme and ODNTzyme were constructed to determine their catalytic activities.
2.3.2. Spatial spacer sequence.
A series of ssDNAs with different spatial spacing sequences were synthesized and denoted as SP-X(O/I). S2 was replaced with SP-X(O/I) to synthesize DNA nanotubes and determine their catalytic activity.
2.3.3. Single-strand concentration ratio.
The single-strand concentration ratio referred to the concentration ratio of single-stranded S1, S3, S4, and S5 to S2 involved in the formation of DNA nanotubes. The concentration of fixed S2 was 1 μM, and the concentration of S1 was varied to 0, 0.5, 1, 2, and 4 μM, respectively (always keeping the concentrations of S1, S3, S4, and S5 equal). The activity of DNTzyme was determined.
2.3.4. G-quadruplex sequence.
A series of different sequences of G-quadruplex were selected and loaded onto ssDNA S2. DNTzymes were synthesized using them instead of S2 and DNTzyme activity was determined.
2.3.5. Array density.
A mixture of G4-S2 (S2 loaded with G-quadruplex) and NG4-S2 (S2 not loaded with G-quadruplex) in different concentration ratios instead of S2 was used to synthesize DNTzymes with different array densities, and DNTzymes activity was determined.
2.4. Stability experiment
2.4.1. Temperature stability.
The activity of DNTzyme was measured after heat treatment at 20–70 °C for 1 h and at 60 °C for 0–4 h, respectively. DNTzyme and HRP were treated in the same way and their activities were compared. The enzyme activity of each sample before heat treatment was taken as 100% and the relative activity was calculated for each of the samples. Three sets of experiments were performed in parallel.
2.4.2. Metal ion stability.
The activity of DNTzyme was determined after 1 h treatment with different metal ions (Na+, K+, Ca2+ and Mg2+, all ions at a concentration of 50 mM, and the control group had no addition of various ions). DNTzyme and HRP were treated in the same way and their activities were compared. The enzyme activity of the control group was taken as 100% and the relative activity was calculated. Three sets of experiments were performed in parallel.
2.4.3. Organic reagent stability.
The activity of DNTzyme was determined after 1 h treatment with different organic reagents (dimethylsulfoxide, acetone, ethanol and glycerol, all reagents at 10% volume fraction, and the control group had no addition of various organic reagents). DNTzyme and HRP were treated identically and their activities were determined. The enzyme activity of the control group was taken as 100% and the relative activity was calculated. Three sets of experiments were performed in parallel.
2.5. Application of the DNTzyme sensor
DNTzyme-like peroxidase activity was measured by oxidizing ADHP in the presence of H2O2 to produce Resorufin that emitted bright red fluorescence. The total volume of the assay system was set at 1 mL. The fluorescence intensity of Resorufin was evaluated by incubating ADHP (8 μM), DNTzyme (1 μM), and various concentrations of H2O2 in buffer A for 5 min.
3. Results and discussion
3.1. Preparation and characterization of DNTzyme arrays
DNA nanotubes loaded with the G-quadruplex were assembled from five ssDNAs, and then combined with hemin to form a complex DNTzyme. The synthesis process is shown in Scheme 1A and B.
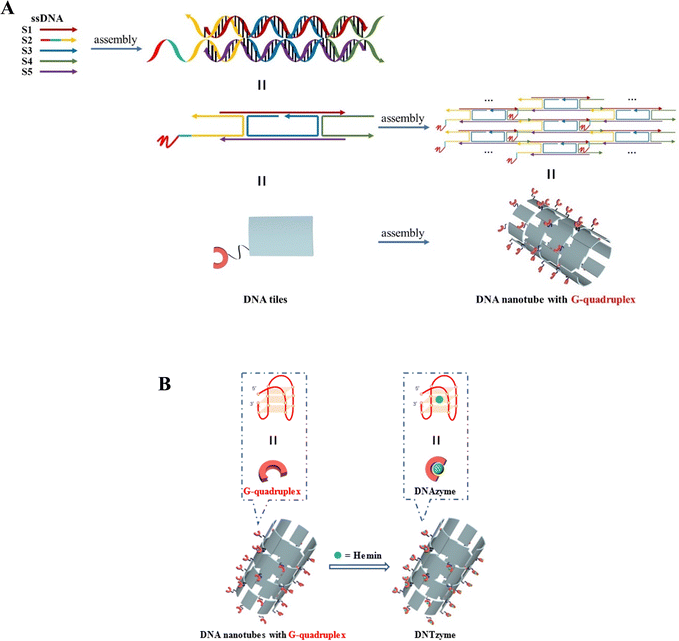 |
| Scheme 1 (A) Schematic diagram of the synthesis of DNA nanotubes loaded with G-quadruplexes; (B) G-quadruplex array combined with hemin to construct DNTzyme. | |
DNA nanotubes were assembled from nanoscale DNA tiles formed by annealing five ssDNAs. The ssDNAs involved in the production of DNA nanotubes were extended in order to establish accurate G-quadruplexes on the DNA nanotubes. After these lengthened ssDNAs were assembled into the DNA nanotubes, the lengthened portions manifested themselves on the DNA nanotubes as regularly aligned branched strands. It was feasible to generate G-quadruplexes with diverse topologies on DNA nanotubes by tailoring the sequences of these branching strands into different G-quadruplex sequences (Scheme 1A). It has been reported that lengthening the 3′-end of ssDNA S2 created a branched strand on the inner surface of DNA nanotubes, whereas lengthening its 5′-end created a branched strand on the outer surface of DNA nanotubes.22 Arrays of G-quadruplexes could thus be formed on the inner or outer surface of DNA nanotubes, termed IDNT and ODNT, respectively. Because the assembly of nanotubes relies on hydrogen bonding between bases, the addition of branched strands could be detrimental to the assembly of nanotubes compared to the absence of branched strands. Therefore, after synthesizing DNA nanotubes with G-quadruplex arrays, the products were analyzed by agarose gel electrophoresis, which confirmed that the addition of branched strands had no substantial negative influence on nanotube synthesis. As demonstrated in Fig. 1A, the mobility of the ODNT and IDNT bands was much lower than that of the ssDNA bands, implying the presence of assembly. Fluorescence labeling was used to visualize the DNA nanotubes. Green lines several tens of microns long could be seen in Fig. 1C and D, indicating the successful synthesis of DNA nanotubes. The TEM image of DNA nanotubes is shown in Fig. S1 (ESI†). To further demonstrate the successful modification of the G-quadruplexes on DNA nanotubes, the CD plots of DNA nanotube samples loaded with the G-quadruplex on the inner or outer surface (IDNT, ODNT) and samples without G-quadruplex (NDNT) were compared. The peaks of the DNA nanotubes loaded with G-quadruplexes became higher at 260–280 nm, as shown in Fig. 2, and this wavelength range corresponded to the peak location of the G-quadruplex standard samples. All these results further verified that the G-quadruplex could be successfully arranged on the DNA nanotubes.
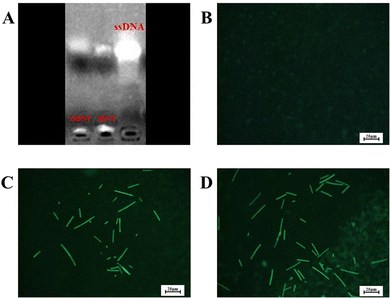 |
| Fig. 1 Electrophoretic analysis of DNTs (A) and fluorescence microscopy images of the blank control (B), ODNT (C) and IDNT (D). | |
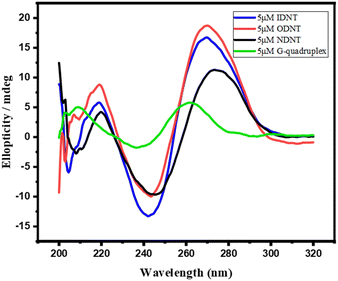 |
| Fig. 2 Circular dichroism of four samples. | |
Subsequently, the binding of DNA nanotubes to hemin was confirmed by UV absorption spectroscopy. The UV absorption spectra of hemin mixtures with various concentrations of the G-quadruplex (ssDNA G4-S2 1–4 μM) or DNAzyme (DNT) are presented in Fig. 3B and C. As the concentration of the G-quadruplex or DNTs increased, the UV absorption of the sample was enhanced, i.e. a colour-enhancing effect occurred. As illustrated in Fig. 3A, the UV absorption of hemin and DNA nanotubes in 1% DMSO solution was weak near 410 nm. However, a strong absorption peak was observed for the mixture of hemin and DNA nanotubes in 1% DMSO solution, indicating successful binding of hemin and DNTs. The binding ratio of hemin to DNA nanotubes was then optimized (Fig. S2, ESI†). When the concentration of hemin was low, the activity of DNTzyme was continuously enhanced by the addition of hemin. When the hemin-to-DNT concentration ratio reached 3
:
1, the addition of hemin no longer significantly increased DNTzyme activity. This result indicated that the binding of hemin to DNTs reached saturation when their concentration ratio reached 3
:
1. Therefore, the hemin-to-DNT concentration ratio was set at 3
:
1 for the synthesis of DNTzyme.
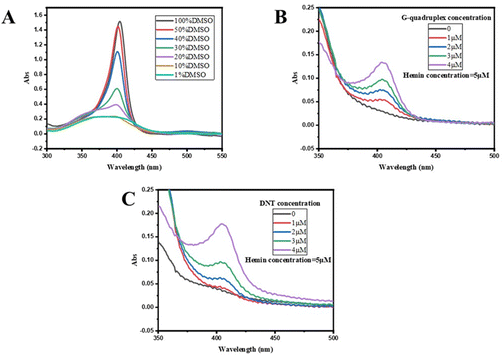 |
| Fig. 3 (A) UV absorption spectra of hemin in DMSO with different concentrations; (B) UV absorption spectra of hemin in the G-quadruplex with different concentrations; and (C) UV absorption spectra of hemin in DNTs with different concentrations. | |
3.2. Factors affecting the catalytic activity of DNTzyme
We investigated the parameters influencing the activity of DNTzyme-like peroxidase after demonstrating the successful synthesis of DNTzyme. The activity was determined by measuring the efficiency of DNTzyme in catalyzing the oxidation of ABTS by H2O2, as illustrated in Scheme 2. We investigated five key factors affecting DNTzyme activity: array loading position (inner or outer surface), spatial spacer sequence, proportion of single-strand concentration, G-quadruplex sequence, and array density.
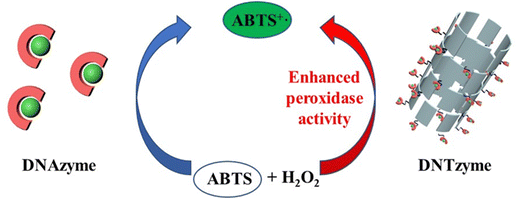 |
| Scheme 2 Schematic diagram of DNAzyme-catalyzed and DNTzyme-catalyzed oxidation of ABTS by H2O2. | |
The peroxidase activity of IDNTzyme and ODNTzyme was compared with that of the free enzymes 3′DNAzyme and 5′DNAzyme, respectively.
3.2.1. Array loading position.
Lengthening the 3′ end of ssDNA S2 produced a branched strand on the inner side of the DNA nanotubes, while extending the 5′ end produced a branched strand on the outer surface of the DNA nanotubes. In this way, G-quadruplexes could be formed on the inner and outer surfaces of DNA nanotubes, and the corresponding constructed DNTzymes were named IDNTzyme and ODNTzyme, respectively (Fig. 4). The activities of IDNTzyme and ODNTzyme were compared. As shown in Fig. 5A, most of the IDNTzyme was more active than the corresponding ODNTzyme. Sequences added to the ends of the G-quadruplex have been shown to impact the peroxidase-like activity of the G-quadruplex/hemin complex.22 Accordingly, it could be hypothesized that the sequences at both ends of the G-quadruplex contributed to the difference in activity between the IDNTzyme and the ODNTzyme. In addition, the activities of IDNTzyme and ODNTzyme's corresponding free DNAzymes (i.e. 3′DNAzyme and 5′DNAzyme) were evaluated and compared. As shown in Fig. 5B, the activity of 3′DNAzyme was generally and significantly better than that of 5′DNAzyme, suggesting that the sequence at both ends of the G-quadruplex had a significant effect on activity.
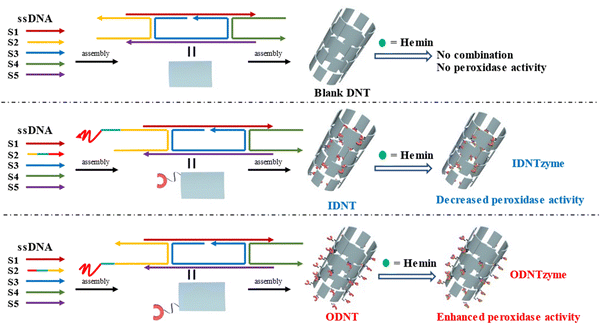 |
| Fig. 4 Schematic diagram of the construction of blank DNTs, IDNTzyme and ODNTzyme. The peroxidase activity of IDNTzyme and ODNTzyme was compared with the free enzymes 3'DNAzyme and 5'DNAzyme, respectively. | |
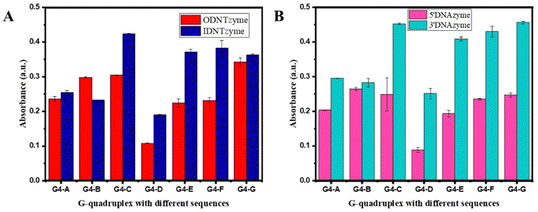 |
| Fig. 5 (A) Comparison of peroxidase-like activities of ODNTzyme and IDNTzyme; (B) comparison of peroxidase-like activities of 5′DNAzyme and 3′DNAzyme. | |
DNTzyme and the equivalent free DNAzyme activity were then compared. As shown in Fig. 6, when the G-quadruplexes were loaded on the outer surface of the DNA nanotubes, the activities of DNTzyme were all higher than those of DNAzyme. However, when G-quadruplexes were loaded on the inner surface of the DNA nanotubes, the activities of DNTzyme were all lower than those of DNAzyme. Loading the G-quadruplexes on the inner or outer surface of the DNA nanotubes resulted in different activity changes. This might be because when the G-quadruplexes were loaded on the outer surface of the DNA nanotubes, the array increased the local concentration of DNAzyme, which in turn increased its activity. When the G-quadruplexes were loaded on the inner surface of the DNA nanotubes, the narrow space restricted G-quadruplex folding and binding to hemin in the DNA nanotubes, thus inhibiting their activity.
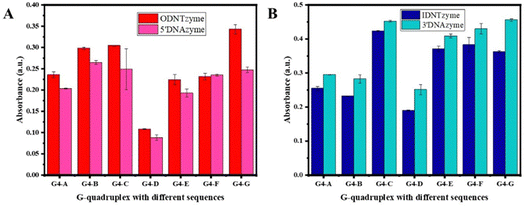 |
| Fig. 6 (A) Comparison of peroxidase-like activities of ODNTzyme and 5′DNAzyme; (B) comparison of peroxidase-like activities of IDNTzyme and 3′DNAzyme. | |
The kinetic parameters of DNTzyme were determined to investigate the mechanism of the changes in the apparent activity of DNTzyme. Table 1 and Fig. S3 (ESI†) reveal that the ODNTzyme exhibited a higher affinity for the substrate and a higher catalytic efficiency than the free DNAzyme. The increased catalytic efficiency was attributed to the high spatiotemporal density on the outside of G-quadruplex/hemin complexes, which increased the affinity of the substrate. In contrast, the catalytic efficiency of IDNTzyme was lower than that of free DNAzyme, although the formation of DNAzyme arrays should have increased its catalytic effectiveness. The lower catalytic efficiency of IDNTzyme compared to free DNAzyme suggested that the limited space prevented contact with the substrates.
Table 1 The kinetic parameters of DNTzyme
|
K
m (mM) |
V
max (nM s−1) |
K
cat (s−1) |
ODNTzyme |
0.672 |
1746 |
1.746 |
5′DNAzyme |
1.021 |
1271 |
1.271 |
IDNTzyme |
1.297 |
1474 |
1.474 |
3′DNAzyme |
1.247 |
1784 |
1.784 |
3.2.2. Space interval sequence.
As mentioned above, the G-quadruplex was attached to ssDNA S2 and thus loaded onto the DNA nanotube. To reduce the negative impacts of the G-quadruplex and the body sequence of S2 on each other, a spacer sequence was added to buffer the spatial resistance between the two (Fig. 7A). The possible adverse effects include: (1) the G-quadruplex preventing the S2 body from participating in the nanotube assembly, (2) the spatial site resistance in the absence of a spacer sequence preventing the nanotube assembly, and (3) normal folding of the G-quadruplex. To avoid the generation of these adverse effects, the spacer sequence was designed to be added between the G-quadruplex and S2, and the sequence was optimized with the metric of obtaining a DNTzyme with the highest possible activity. Four groups of DNTzymes with spacer sequence bp numbers 0, 3, 6, and 16 (including ODNTzyme and IDNTzyme) had corresponding DNAzyme activity (including 5′DNAzyme and 3′DNAzyme). As shown in Fig. 7B, different spacer sequences had a less effect on the activity of IDNTzyme and a more effect on the activity of ODNTzyme. Among them, the activity of ODNTzyme corresponding to 16 bp was higher than other ODNTzyme activities, and Fig. 7C reveals that spacer sequences had less of an effect on DNAzyme. This result suggested that the elevated activity of the 16 bp ODNTzyme was attributable to the buffer region supplied by the larger spacer sequence rather than the sequence itself. The adequate buffer space aided in the spatial arrangement and appropriate folding of the G-quadruplex. This phenomenon was not observed for IDNTzyme because the narrow space inside the DNA nanotubes restricted G-quadruplex alignment and folding, which was consistent with the previous results. Meanwhile, the results again confirmed that the activities of 3′DNAzyme and IDNTzyme, which were constructed by loading the G-quadruplex at the 3′ end, were superior to those of 5′DNAzyme and ODNTzyme (Fig. 7B and C). ODNTzyme activity was superior to that of the equivalent 5′DNAzyme, whereas IDNTzyme activity was lower than that of the corresponding 3′DNAzyme (Fig. 7D and E), and the results were consistent with the previous results.
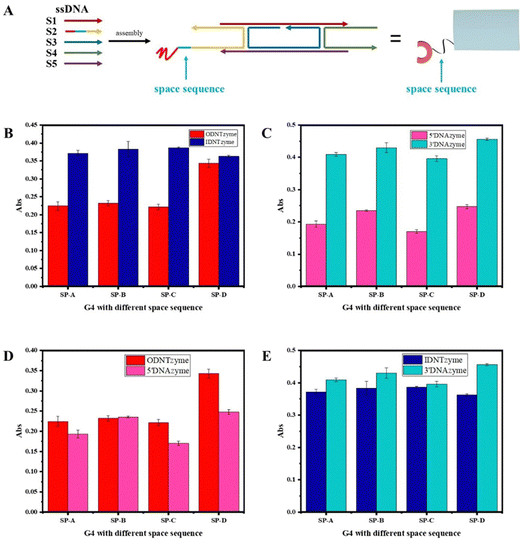 |
| Fig. 7 (A) Schematic diagram of the space sequence; (B) comparison of peroxidase-like activities of ODNTzyme and IDNTzyme; (C) comparison of peroxidase-like activities of 5′DNAzyme and 3′DNAzyme; (D) comparison of peroxidase-like activities of ODNTzyme and 5′DNAzyme; and (E) comparison of peroxidase-like activities of IDNTzyme and 3′DNAzyme. | |
3.2.3. Single-chain concentration ratio.
The methods for synthesising DNA nanotubes published in the literature virtually invariably used the same concentration of five ssDNAs. Furthermore, even at the same concentration of five ssDNAs, not all ssDNAs were fully involved in the creation of nanotubes. However, in this study, ssDNA S2 loaded with G-quadruplexes had a higher “status” than the other four ssDNAs. Increasing the concentration of strands S1, S3, S4, and S5 allowed S2 to fully participate in the production of nanotubes. As shown in Fig. S4 (ESI†), when the concentration of S2 was the same in all systems, the higher the concentration of S1, S3, S4 and S5 in the system, the stronger the ODNTzyme activity and the lower the IDNTzyme activity. This result confirms that the formation of an array was the reason for the change in activity.
3.2.4. G-quadruplex sequence.
The peroxidase-like activity of the DNAzyme produced by distinct sequences of the G-quadruplex coupled to hemin differed significantly. Therefore, the activity of DNTzyme constructed from different sequences of the G-quadruplex was predicted to exhibit large variances. DNTzyme produced from different G-quadruplex sequences was compared to free DNAzyme in studies. As demonstrated in Fig. 8, as expected, the DNTzyme activities corresponding to the different G-quadruplexes varied substantially. The DNTzymes corresponding to PS2.M, PS5.M, T30695, and Hg3 (the topological structures of different G-quadruplex are shown in Fig. S5, ESI†) showed considerable activity increase of up to 106%. However, the degree of increase was not uniform, with the lowest being only 12%. This could be because different G-quadruplex sequences were incompatible with the primary sequence of DNA nanotubes, i.e. the folding of the two may affect each other.
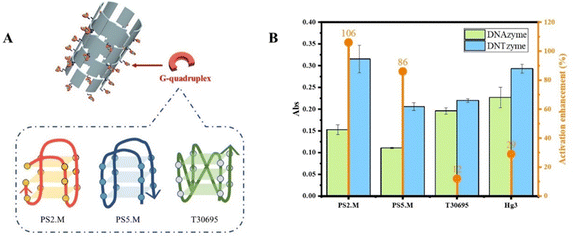 |
| Fig. 8 (A) Schematic diagram of G-quadruplexes with different structures; (B) comparison of the activities of DNTzyme and DNAzyme constructed with G-quadruplexes with different structures. | |
3.2.5. Array density.
Nanotubes with G-quadruplex arrays of different densities were successfully prepared using mixtures of G4-S2 and S2 in different ratios instead of G4-S2, as shown in Fig. S5A (ESI†). To measure the density of G-quadruplex arrays on the nanotubes, DNA nanotubes were synthesized with G4-S2 labeled with the fluorescent molecule FAM and purified by ultrafiltration. The more dense the G-quadruplex arrangement on the nanotubes, the more fluorescent molecules form on the pure nanotubes, and the higher the fluorescence intensity of the solution. Thus, the array density could be indirectly determined by monitoring the fluorescence intensity. However, because the relationship between the fluorescence intensity and the array density was not linear, the degree of array density could only be inferred qualitatively from the fluorescence intensity. As shown in Fig. S6B (ESI†), the peroxidase-like activity of ODNTzymes increased with increasing array density. This clarified the rationale for the increased activity. A high concentration of DNAzyme in the local microenvironment was key to the enhanced activity. Besides, the formation of arrays was essential to increase the local concentration of DNAzyme, i.e. the formation of DNAzyme arrays on DNA nanotubes resulted in increased DNTzyme activity relative to free DNAzyme.
3.3. Stability of DNTzyme
The stability of the catalyst was an essential measure of its excellence. We compared DNTzyme's temperature, metal ions, and organic reagent stability to those of HRP.
The activities of DNTzyme, DNAzyme, and HRP were assessed after heat treatment at 20–70 °C for 1 h. As shown in Fig. S7A (ESI†), the activities of DNTzyme and DNAzyme were almost unchanged after heat treatment at 60 °C or less for 1 h, whereas the activity of HRP decreased significantly after heat treatment at 50 °C for 1 h. The activities of DNTzyme, DNAzyme, and HRP were also determined for heat treatment at 60 °C for 0–4 h. As shown in Fig. S7B (ESI†), the activities of DNTzyme and DNAzyme were almost unchanged, while the activity of HRP was almost completely lost with increasing heat treatment time. These results suggested that DNTzyme had much better thermal stability than HRP.
The activities of DNTzyme, DNAzyme, and HRP were determined after 1 h treatment with various metal ions and organic reagents. Fig. S7C (ESI†) shows that the activities of DNTzyme, DNAzyme, and HRP were all lost to variable degrees after metal ion treatment, because the different salt ions affected the structure of DNA and proteins. The activity of DNTzyme, DNAzyme, and HRP was lost to variable degrees after treatment with various organic reagents (Fig. S7D, ESI†), and the activities of DNTzyme and DNAzyme were lost more. This could be because organic reagents were more destructive to the DNA structure.
3.4. Application of DNTzyme in detecting peroxide explosives
DNAzyme is widely used in biosensors. Recognition elements can be constructed for sensitive detection of target substances using G-quadruplex/hemin complexes and catalysis by H2O2. Based on this, DNTzyme was used to create a simple, quick, and cost-effective sensor for the detection of peroxides and achieving enhanced fluorescence. The principle of peroxide detection was that peroxides oxidize ADHP under the action of DNTzyme to produce resorufin, which could produce a strong red fluorescence (Fig. 9A). The effectiveness of DNTzyme and free DNAzyme in detecting explosives was compared. As shown in Fig. 9B, both DNTzyme and free DNAzyme had good linearity for H2O2 detection in the range of 0–1000 nM. The detection limit of H2O2 for DNTzyme was 49 nM, whereas the detection limit of H2O2 for DNAzyme was 77 nM, which meant that DNTzyme had a better detection effect. The same method was used to detect TBHP. As shown in Fig. 9C, its linearity was still good, and the detection limits of TBHP for DNTzyme and DNAzyme were 1.4 μM and 2.4 μM, respectively. The color development reaction lasted 5 min, which was quite efficient. These results indicated that the constructed peroxide sensor was sensitive, accurate, and efficient.
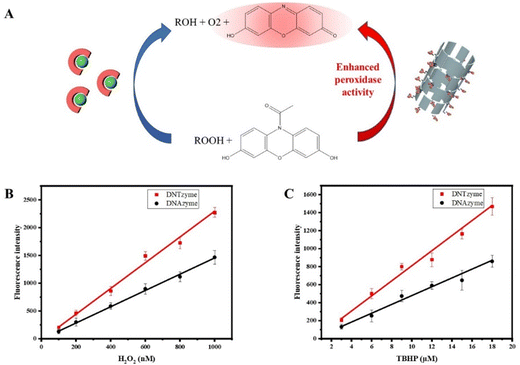 |
| Fig. 9 (A) Schematic diagram of DNTzyme-catalyzed oxidation of ADHP by H2O2. The sensors constructed by DNTzyme and DNAzyme were used to detect H2O2 (B) and TBHP (C). | |
4. Conclusion
We successfully constructed G-quadruplex arrays on DNA nanotubes and confirmed by agarose gel electrophoresis, fluorescence microscopy, and circular dichroism. The binding of G-quadruplex arrays to hemin was confirmed by UV absorption spectroscopy, and the binding ratio of hemin to G-quadruplex arrays was optimized. Some key factors affecting the catalytic activity of DNTzyme were then investigated, including the array loading position, spacer sequence, single-strand concentration ratio, G-quadruplex sequence, and array density. Finally, a peroxide detection sensor was constructed based on the enhanced enzymatic activity and excellent temperature stability of DNTzyme. The sensor can reliably and efficiently detect peroxide and fluorescence enhancement levels with good linearity. The detection limit for H2O2 was 49 nM, the detection limit for TBHP was 1.4 μM, and the color development time was about 5 min. Using the G-quadruplex/hemin complex as an example, this research highlighted the value and potential of DNTs as backbone materials by constructing arrays to effectively enhance the activity of DNAzyme. Because of the functional diversity of G-quadruplexes, G-quadruplex arrays on DNTs were projected to have a higher application value in bio-detection, biocatalysis, and drug delivery.
Author contributions
Ying Zhang: experimental design and planning, performing the experiment, writing the initial draft and revising the manuscript; Lingqi Wu: performing the experiments and drawing of the diagram; Xin Su: ideas, oversight and leadership responsibility for the research activity planning and execution and revised the initial draft; Hao Liang: ideas, oversight and leadership responsibility for the research activity planning and execution, revised the initial draft and funding acquisition.
Conflicts of interest
The authors declare no conflict of interest.
Acknowledgements
This study was funded by the National Natural Science Foundation of China (22078014).
References
- R. J. Hooley, Nat. Chem., 2016, 8, 202–204 CrossRef CAS PubMed.
- W. Xu, L. Jiao, Y. Wu, L. Hu, W. Gu and C. Zhu, Adv. Mater., 2021, 33, 2005172 CrossRef CAS PubMed.
- H. Zhang, S. Li, A. Qu, C. Hao, M. Sun, L. Xu, C. Xu and H. Kuang, Chem. Sci., 2020, 11, 12937–12954 RSC.
- B. Saif and P. Yang, ACS Appl. Bio. Mater., 2021, 4, 1156–1177 CrossRef CAS PubMed.
- T. Sun, S. He, Z. Xu, J. Zuo, Y. Yu and W. Yang, Org. Biomol. Chem., 2021, 19, 3589–3594 RSC.
- B. J. Bloomer, D. S. Clark and J. F. Hartwig, Biochemistry, 2023, 62, 221–228 CrossRef CAS PubMed.
- F. Schwizer, Y. Okamoto, T. Heinisch, Y. Gu, M. M. Pellizzoni, V. Lebrun, R. Reuter, V. Köhler, J. C. Lewis and T. R. Ward, Chem. Rev., 2018, 118, 142–231 CrossRef CAS PubMed.
- J. H. Yum, H. Sugiyama and S. Park, Chem. Rec., 2022, 22, e202100333 CrossRef CAS PubMed.
- J. Dong, X. Qiu, M. Huang, X. Chen and Y. Li, Talanta, 2023, 257, 124384 CrossRef CAS PubMed.
- L. Gao, Y. Deng, H. Liu, K. Solomon, B. Zhang and H. Cai, Biosensors, 2022, 12, 745 CrossRef CAS PubMed.
- S. K. Silverman, Angew. Chem., Int. Ed., 2010, 49, 7180–7201 CrossRef CAS PubMed.
- S. Park and H. Sugiyama, Molecules, 2012, 17, 12792–12803 CrossRef CAS PubMed.
- S. Park and H. Sugiyama, Angew. Chem., Int. Ed., 2010, 49, 3870–3878 CrossRef CAS PubMed.
- O. I. Wilner, R. Orbach, A. Henning, C. Teller, O. Yehezkeli, M. Mertig, D. Harries and I. Willner, Nat. Commun., 2011, 2, 540 CrossRef PubMed.
- P. W. Rothemund, Nature, 2006, 440, 297–302 CrossRef CAS PubMed.
- A. Rangnekar, K. V. Gothelf and T. H. LaBean, Nanotechnology, 2011, 22, 235601 CrossRef PubMed.
- J. H. Yum, S. Park and H. Sugiyama, Org. Biomol. Chem., 2019, 17, 9547–9561 RSC.
- Y. Chen, D. Qiu, X. Zhang, Y. Liu, M. Cheng, J. Lei, J. L. Mergny, H. Ju and J. Zhou, Anal. Chem., 2022, 94, 2212–2219 CrossRef CAS PubMed.
- S. Haldar, Y. Zhang, Y. Xia, B. Islam, S. Liu, F. L. Gervasio, A. J. Mulholland, Z. A. E. Waller, D. Wei and S. Haider, J. Am. Chem. Soc., 2022, 144, 935–950 CrossRef CAS PubMed.
- J. Wang, D.-X. Wang, B. Liu, X. Jing, D.-Y. Chen, A.-N. Tang, Y.-X. Cui and D.-M. Kong, Adv. Mater., 2022, 17, e202101315 CAS.
- D.-X. Wang, J. Wang, Y.-X. Wang, Y.-C. Du, Y. Huang, A.-N. Tang, Y.-X. Cui and D.-M. Kong, Chem. Sci., 2021, 12, 7602–7622 RSC.
- T. Chang, H. Gong, P. Ding, X. Liu, W. Li, T. Bing, Z. Cao and D. Shangguan, Chemistry, 2016, 22, 4015–4021 CrossRef CAS PubMed.
|
This journal is © The Royal Society of Chemistry 2024 |
Click here to see how this site uses Cookies. View our privacy policy here.