Manganese, nitrogen co-doped porous carbon with high-loading active sites as the oxygen reduction catalyst for Zn–air batteries†
Received
9th June 2024
, Accepted 20th June 2024
First published on 21st June 2024
Abstract
Manganese–nitrogen–carbon (Mn–N–C) materials have been considered ideal catalysts for the oxygen reduction reaction (ORR). However, avoiding metal agglomeration during pyrolysis while ensuring the construction of Mn–Nx active centers is still difficult. Furthermore, the influence of pyrolysis temperature on structures of Mn–N–C is controversial, and needs an in-depth understanding. Herein, we prepare single atom Mn–N–C via a spatial confinement method. This is achieved through the pyrolysis of the ZIF precursor, where single Mn(acac)3 molecules are trapped within its cavities. Compared with 800 °C and 1000 °C, 900 °C is the optimal pyrolysis temperature for the synthesis of ZIF-derived Mn–N–C, which promotes the formation of the porous framework and dense Mn–Nx centers (27.47%). As a result, Mn–N–C-900 possesses an outstanding performance with a half-wave potential (E1/2) of 0.882 V in alkaline media. Impressively, the Zn–air battery using Mn–N–C-900 delivers a high peak power density of 84 mW cm−2.
Introduction
In order to achieve carbon neutrality goals and accelerate the development of an environment-friendly society, the next generation of clean energy devices, including Zn–air batteries (ZABs), has garnered significant attention because of low pollution features.1–12 However, the actual performance of ZABs is limited by the slow ORR kinetics.13–19 As benchmark catalysts for the ORR, Pt-based catalysts are costly and prone to instability, making them unsuitable for widespread commercial applications.20–23 Therefore, there is a pressing demand for the application of non-precious metal materials.24–28
Mn–N–C has emerged as a hopeful alternative to Pt/C.29,30 The atomically dispersed Mn–Nx site is recognized as the main active center, while the porous structure can enhance the accessibility of Mn–Nx centers.31,32 However, the manganese species in precursors are often aggregated into low-activity nanoparticles during pyrolysis, which hinders the construction of Mn–Nx centers.33 Moreover, the influence of pyrolysis temperature on the Mn–Nx center loading and pore framework of Mn–N–C catalysts is still unclear, and needs further study. Hence, exploiting a facile approach to achieve the controllable construction of single atom Mn–Nx sites and studying the correlation between pyrolysis temperature and the structure of catalysts are necessary for the development of Mn–N–C.
Herein, we report a spatial confinement method to prepare Mn–N–C by pyrolyzing the zeolitic imidazolate framework (ZIF) with single Mn(acac)3 molecules trapped within its cavities. The spatial confinement effect of ZIF-8 nanocavities on Mn(acac)3 molecules can avoid the appearance of manganese-based nanoparticles. Compared with 800 and 1000 °C, 900 °C is the optimal pyrolysis temperature for the synthesis of ZIF-derived Mn–N–C. The Mn–N–C-900 synthesized at 900 °C exhibits a more prominent porous structure and a higher loading of Mn–Nx active sites than those of reference samples. As a result, the optimized Mn–N–C-900 possesses outstanding activity and durability with an E1/2 of 0.882 V in an alkaline solution. Impressively, the solid-state ZAB utilizing Mn–N–C-900 reveals an eminent peak power density.
Experimental
Synthesis of the catalysts
Preparation of the ZIF-8@Mn(acac)3 precursor: in a typical procedure, solution A was obtained by dissolving 10 mg Mn(acac)3 and 576 mg Zn(NO3)2·6H2O in 20 mL CH3OH. Simultaneously, solution B was obtained by dissolving 4.5 g 2-methylimidazole (2-MeIM) in 80 mL CH3OH. Finally, solution A was rapidly combined with solution B, and the mixed solution was stirred at 23 °C for 24 h. The precipitate obtained was then washed 3 times with CH3OH and dried at 60 °C for twelve hours.
Preparation of Mn–N–C-900: ZIF-8@Mn(acac)3 was pyrolyzed at 900 °C in an Ar/H2 (95%:5%) atmosphere for 2 h at 5 °C min−1.
To reveal the influence of pyrolysis temperature on Mn–Nx loading and the pore structure, reference Mn–N–C-800 and Mn–N–C-1000 catalysts were prepared using a similar process to Mn–N–C-900, except for choosing 800 °C and 1000 °C as the pyrolysis temperature, respectively.
Physicochemical characterization
A scanning electron microscope (SEM, Zeiss) and transmission electron microscope (TEM, JEOL JEM 2100) were employed to study the morphology. The X-ray diffraction (XRD) patterns and Raman spectra were collected on a Bruker D8 and a HR800, respectively. X-ray photoelectron spectroscopy (XPS) and the N2 adsorption–desorption test were conducted on a Physical Electronics PHI model 5700 instrument and 3H–2000PS1 analyzer, respectively.
Electrochemical measurements
The mass loading of Mn–N–C and Pt/C on the working electrode was 0.459 and 0.255 mg cm−2, respectively. The preparation method of catalyst ink is provided in our previous reports.34
Solid state Zn–air battery test: Zn foil, PVA polymer and nickel foam coated with the catalyst (2 mg cm−2) were chosen as the anode, solid-state electrolyte and cathode, respectively. The preparation method of polyvinyl alcohol (PVA) electrolyte is provided in previous reports.34
Results and discussion
Structural characterization
The synthetic process of the ZIF-8@Mn(acac)3 precursor and its resulting Mn–N–C is exhibited in Scheme 1. Initially, the ZIF-8@Mn(acac)3 precursor is synthesized through the self-assembly of Zn2+, 2-MeIM, and Mn(acac)3 in a methanol solution. The spatial confinement effect of ZIF-8 nanocavities on Mn(acac)3 effectively prevents the aggregation of metal species during the pyrolysis process. After pyrolysis of precursors under H2/Ar at various temperatures (800, 900 and 1000 °C), Mn–N–C materials with different active center loadings and pore structures are synthesized. The final mass yield of Mn–N–C-900 is 0.0721 g (Fig. S1†).
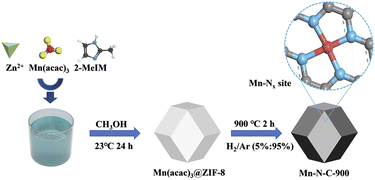 |
| Scheme 1 Synthetic process of Mn–N–C-900. | |
The morphology of the ZIF-8@Mn(acac)3 precursor and the obtained Mn–N–C catalysts are detected by SEM images. As shown in Fig. 1a, ZIF-8@Mn(acac)3 presents a coral-like structure consisting of abundant nano-polyhedra. After the pyrolysis treatment, the surface of the obtained catalysts becomes rougher (Fig. 1b–d), which is due to the decomposition of nitrogen sources. As shown in TEM and HRTEM images, no nanoparticles are observed in Mn–N–C-900 catalysts (Fig. 1e–f), proving that the size of manganese species may be at the atomic level.
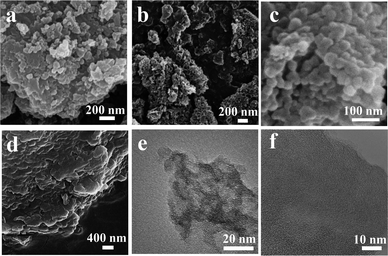 |
| Fig. 1 SEM images of (a) the precursor and the obtained Mn–N–C at (b) 800 °C, (c) 900 °C and (d) 1000 °C; (e) TEM and (f) HRTEM images of Mn–N–C-900. | |
XRD is employed to detect the crystal structure of the catalysts (Fig. 2a). Two peaks corresponding to the C (002) and C (101) planes are detected in all samples.34 No diffraction peaks corresponding to crystalline manganese-based species are detected. The above results indicate that there is no significant metal agglomeration during the pyrolysis process, and the size of Mn species may be at the atomic level. Raman spectra are employed to investigate the influence of pyrolysis temperature on the graphitization degree of the catalysts. As shown in Fig. 2b, two peaks are observed in the synthesized samples, corresponding to the D-band (carbon defects) and G-band (graphitization), respectively.35,36 As the pyrolysis temperature increases from 800 °C to 1000 °C, the ID/IG values of Mn–N–C catalysts decrease gradually from 0.89 to 0.87. This trend indicates that high pyrolysis temperatures can increase the graphitization degree, thereby improving the conductivity of the catalysts. In addition, compared with the reference samples, Mn–N–C-900 possesses higher specific surface area (SBET, 1062 m2 g−1) and pore volume (Vpore, 0.158 cm3 g−1) (Fig. 2c–d), leading to enhanced accessibility of active sites within the catalyst.
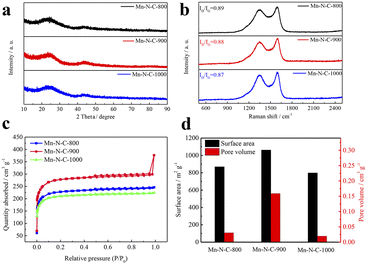 |
| Fig. 2 (a) XRD patterns; (b) Raman spectra; (c) N2 adsorption–desorption curves; and (d) SBET and Vpore. | |
The evaporation of Zn at 900 °C leads to the formation of a large number of micropores,37 so the obtained Mn–N–C-900 exhibits larger SBET and Vpore than those of Mn–N–C-800. Nevertheless, when the temperature is increased to 1000 °C, the pore structure of Mn–N–C-1000 undergoes significant collapse, resulting in poor SBET and Vpore. The above results indicate that 900 °C is the optimal pyrolysis temperature for the synthesis of ZIF-derived Mn–N–C, which is conducive to the construction of a porous structure and large specific surface area.
The influence of pyrolysis temperature on the chemical composition of Mn–N–C is verified by XPS. The C 1s spectra of the obtained catalysts exhibit three peaks, attributed to C–C (284.8 ± 0.3 eV), C–N (285.4 ± 0.3 eV) and C–O (286.5 ± 0.3 eV) (Fig. S3†).15 The presence of a C–N bond proves the introduction of N species into the carbon substrate. In addition, there are three peaks in the Mn 2p spectrum of the Mn–N–C-900 catalyst at 641.6 ± 0.3, 653.6 ± 0.3 and 646.0 ± 0.3 eV, attributed to Mn 2p3/2 and Mn 2p1/2 and the satellite peak, respectively (Fig. S4†).38 The N 1s spectra of the obtained Mn–N–C exhibit five nitrogen species, oxidized-N (402–405 eV), graphitic-N (401.5 ± 0.3 eV), pyrrole-N (400.7 ± 0.3 eV), Mn–N (399.3 ± 0.3 eV) and pyridinic-N (398.4 ± 0.3 eV) (Fig. 3a–c).15 Previous work has demonstrated the importance of pyridinic-N in capturing manganese atoms to construct the Mn–Nx site and enhancing the adsorption of O2 molecules on the catalysts.39 The relative content of pyridinic-N in Mn–N–C-900 (44.8%) is higher than that in Mn–N–C-800 (27.82%) and Mn–N–C-1000 (25.31%) (Fig. 3d and Table S1†). Meanwhile, it is generally accepted that the atomically dispersed Mn–Nx site is the main active center. Compared with the reference samples, Mn–N–C-900 has a higher relative content of Mn–Nx sites (27.47%) (Fig. 3d and Table S1†).
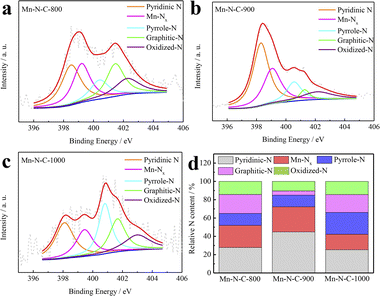 |
| Fig. 3 (a–c) N 1s spectra of Mn–N–C catalysts. (d) The relative content of N species. | |
Obviously, as the temperature increases from 800 °C to 900 °C, more Mn–Nx and pyridinic-N are formed in catalysts. However, at an excessive temperature of 1000 °C, there is a notable decomposition of nitrogen species, resulting in a decrease in the relative content of Mn–Nx and pyridinic-N. The above results indicate that the optimal pyrolysis temperature for the synthesis of ZIF-derived Mn–N–C is 900 °C. 900 °C promotes the formation of pyridinic-N and Mn–Nx active sites, potentially leading to higher catalytic activity compared to reference catalysts. The following electrochemical tests strongly support the above viewpoint.
Oxygen reduction performance evaluation
The performance of Mn–N–C is assessed in 0.1 M KOH. The E1/2 of Mn–N–C-900 reaches 0.882 V (Fig. 4a), which is superior to that of reference Mn–N–C samples, confirming the satisfactory activity of Mn–N–C-900. Moreover, among the synthesized ZIF-derived materials, Mn–N–C-900 demonstrates the highest kinetic current density (Fig. 4b). Furthermore, Mn–N–C-900 possess the lowest Tafel slope of 19.21 mV dec−1, suggesting the outstanding reaction kinetics (Fig. 4c).18,20 On the other hand, the accelerated durability test is employed to assess the stability of Mn–N–C-900. The decay in E1/2 (only 3 mV) for Mn–N–C-900 is almost negligible after 5000 cycles (Fig. 4d). Obviously, the obtained Mn–N–C-900 possesses remarkable activity and stability, which is attributed to abundant Mn–Nx sites and a significant porous structure. 900 °C is the optimal pyrolysis temperature for the preparation of ZIF-derived Mn–N–C.
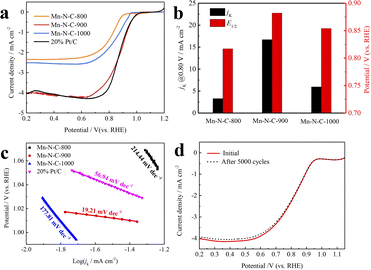 |
| Fig. 4 ORR measurements in 0.1 M KOH: (a) LSV curves; (b) kinetic current density; (c) Tafel plots; and (d) results of the accelerated durability test. | |
Furthermore, we assemble and test the primary ZAB utilizing Mn–N–C-900. The detailed structure of the primary ZAB is shown in Fig. 5a. This study does not involve the installation and testing of rechargeable ZABs. As demonstrated in Fig. 5b, the open circuit voltage of a battery utilizing Mn–N–C-900 is found to be approximately 1.5 V. Additionally, the peak power density of the Mn–N–C-900-based battery (84 mW cm−2) is higher than that of Pt/C (Fig. 5c). Moreover, the Mn–N–C-900 based battery has the capability to power a timer successfully (Fig. 5d). Therefore, the ZABs using Mn–N–C-900 as catalysts exhibit great potential for practical application.
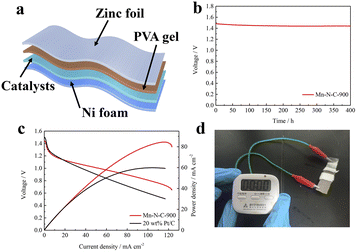 |
| Fig. 5 (a) The structure of the solid-state ZAB. (b) Voltage-time curves. (c) Polarization curves. (d) Photograph of a timer powered by a solid-state battery using Mn–N–C-900. | |
Conclusions
In summary, our work utilizes the spatial confinement method to prepare the single atom Mn–N–C. This is achieved through the pyrolysis of the ZIF precursor, where single Mn(acac)3 molecules are trapped within its cavities. Benefiting from the porous structure and the high loading of Mn-Nx, the resulting Mn–N–C-900, synthesized at 900 °C, shows outstanding catalytic activity and stability with an E1/2 of 0.882 V in 0.1 M KOH, which surpasses those of the reference samples synthesized at 800 °C and 1000 °C. Furthermore, the peak power density of the ZAB utilizing Mn–N–C-900 can reach 84 mW cm−2. Our work provides insights into the synthesis of Mn–N–C, and revealing the impact of pyrolysis temperature on the structure of catalysts.
Data availability
The data supporting this article have been included as part of the ESI.†
Author contributions
Hao Xu: data curation, writing – original draft; Yuxuan Gao: data curation, writing – original draft; Ruopeng Li: conceptualization, writing – review & editing; Weiyan Sun: investigation, software; Xiangyu Lu: writing – review & editing; Jie Bai: investigation, software; Peixia Yang: writing – review & editing.
Conflicts of interest
There are no conflicts to declare.
Acknowledgements
This work was supported by the Shiyanjia Lab (http://www.shiyanjia.com), (GZC20233449) Postdoctoral Fellowship Program of CPSF, (52163029) National Natural Science Foundation of China, and (LBH-Z23156) Postdoctoral Fellowship of Heilongjiang.
References
- Y. Zhu, Y. Jiang, H. Li, D. Zhang, L. Tao, X. Z. Fu, M. Liu and S. Wang, Tip-like Fe-N4 Sites Induced Surface Microenvironments Regulation Boosts the Oxygen Reduction Reaction, Angew. Chem., Int. Ed., 2024, 63, e202319370 CrossRef CAS PubMed.
- Y. Zhang, X. Ma, Z. Cheng, J. Wang, G. Wen, L. Yang and Z. Bai, Fast-Charging Zinc-Air Batteries with Stable Bifunctional Oxygen Electrocatalysis of Mo-Induced Nanodefective Mo-Co/N-C Catalyst, Adv. Funct. Mater., 2024, 2314622, DOI:10.1002/adfm.202314622.
- X. Zhang, F. Yang, S. Sun, K. Wei, H. Liu, G. Li, Y. Sun, X. Li, J. Qian, S. Du, M. Li, Y. Lu, C. Xia, S. Che and Y. Li, Boosting oxygen reduction via MnP nanoparticles encapsulated by N, P-doped carbon to Mn single atoms sites for Zn-air batteries, J. Colloid Interface Sci., 2024, 657, 240–249 CrossRef CAS PubMed.
- P. Zhang, H.-C. Chen, H. Zhu, K. Chen, T. Li, Y. Zhao, J. Li, R. Hu, S. Huang, W. Zhu, Y. Liu and Y. Pan, Inter-site structural heterogeneity induction of single atom Fe catalysts for robust oxygen reduction, Nat. Commun., 2024, 15, 2062 CrossRef CAS PubMed.
- T. Zhang, N. Wu, Y. Zhao, X. Zhang, J. Wu, J. Weng, S. Li, F. Huo and W. Huang, Frontiers and Structural Engineering for Building Flexible Zinc-Air Batteries, Adv. Sci., 2022, 9, 2103954 CrossRef CAS PubMed.
- L. Liu, Y. Wu, H. Wang, X. Yang, W. Zhu, S. Ma, X. Lu, Y. Li, P. Ren, P. Yang and R. Li, Pore-edge graphitic nitride-dominant hierarchically porous carbons for boosting oxygen reduction catalysis, Sustainable Energy Fuels, 2024, 8, 2050–2058 RSC.
- W. Zhou, Y. Liu, D. Wu, L. Zhou, G. Zhang, K. Sun, B. Li and J. Jiang, Biomass chitosan-derived Co-induced N-doped carbon nanotubes to support Mn3O4 as efficient electrocatalysts for rechargeable Zn-air batteries, Sustainable Energy Fuels, 2023, 7, 1698–1706 RSC.
- W. Guo, X. Teng, Q. Zhao, B. Zhang, Q. Yue, W. Tan, H. Du, J. Yu and B. Zhou, Polypyrrole hydrogel as a universal precursor for the target preparation of heteroatom-doped hierarchical carbon with atomically distributed metal sites towards high-efficiency ORR and Zn-air batteries, Sustainable Energy Fuels, 2023, 7, 515–525 RSC.
- W. Shao, R. Yan, M. Zhou, L. Ma, C. Roth, T. Ma, S. Cao, C. Cheng, B. Yin and S. Li, Carbon-Based Electrodes for Advanced Zinc-Air Batteries: Oxygen-Catalytic Site Regulation and Nanostructure Design, Electrochem. Energy Rev., 2023, 6, 11 CrossRef CAS.
- M. Wu, X. Yang, X. Cui, N. Chen, L. Du, M. Cherif, F. Chiang, Y. Wen, A. Hassanpour, F. Vidal, S. Omanovic, Y. Yang, S. Sun and G. Zhang, Engineering Fe-N4 Electronic Structure with Adjacent Co-N2C2 and Co Nanoclusters on Carbon Nanotubes for Efficient Oxygen, Electrocatalysis, Nano-Micro Lett., 2023, 15, 232 CrossRef CAS PubMed.
- M. Wu, F. Dong, Y. Yang, X. Cui, X. Liu, Y. Zhu, D. Li, S. Omanovic, S. Sun and G. Zhang, Emerging Atomically Precise Metal Nanoclusters and Ultrasmall Nanoparticles
for Efficient Electrochemical Energy Catalysis: Synthesis Strategies and Surface/Interface Engineering, Electrochem. Energy Rev., 2024, 7, 10 CrossRef CAS.
- T. Wang, J. Huang, W. Sang, C. Zhou, B. Zhang, W. Zhu, K. Du, Z. Kou and S. Wang, Correlative Mn-Co catalyst excels Pt in oxygen reduction reaction of quasi-state zinc-air batteries, Nano Res., 2024, 17, 4118–4124 CrossRef CAS.
- W. Kang, L. Zeng, X. Liu, H. He, X. Li, W. Zhang, P. S. Lee, Q. Wang and C. Zhang, Insight into Cellulose Nanosizing for Advanced Electrochemical Energy Storage and Conversion: A Review, Electrochem. Energy Rev., 2022, 5, 8 CrossRef CAS.
- W. Wei, X. Deng, K. Zhao, M. Zhang, H. Zhou and W. Wang, Dual-metal M-N-C (M = Mn, Co) as an effective oxygen electrocatalyst for zinc-air battery, J. Alloys Compd., 2023, 968, 172136 CrossRef CAS.
- H. Xu, L. Xiao, P. Yang, X. Lu, L. Liu, D. Wang, J. Zhang and M. An, Solvent environment engineering to synthesize Fe-N-C nanocubes with densely Fe-Nx sites as oxygen reduction catalysts for Zn-air battery, J. Colloid Interface Sci., 2023, 638, 242–251 CrossRef CAS PubMed.
- H. Tian, A. Song, P. Zhang, K. Sun, J. Wang, B. Sun, Q. Fan, G. Shao, C. Chen, H. Liu, Y. Li and G. Wang, High Durability of Fe-N-C Single-Atom Catalysts with Carbon Vacancies toward the Oxygen Reduction Reaction in Alkaline Media, Adv. Mater., 2023, 35, 2210714 CrossRef CAS PubMed.
- M. Shen, J. Liu, J. Li, C. Duan, C. Xiong, W. Zhao, L. Dai, Q. Wang, H. Yang and Y. Ni, Breaking the N-limitation with N-enriched porous submicron carbon spheres anchored Fe single-atom catalyst for superior oxygen reduction reaction and Zn-air batteries, Energy Storage Mater., 2023, 59, 102790 CrossRef.
- H. Liu, L. Jiang, Y. Wang, X. Wang, J. Khan, Y. Zhu, J. Xiao, L. Li and L. Han, Boosting oxygen reduction with coexistence of single-atomic Fe and Cu sites decorated nitrogen-doped porous carbon, Chem. Eng. J., 2023, 452, 138938 CrossRef CAS.
- Z. Li, S. Ji, H. Liu, C. Xu, C. Guo, X. Lu, H. Sun, S. Dou, S. Xin, J. H. Horton and C. He, Constructing Asymmetrical Coordination Microenvironment with Phosphorus-Incorporated Nitrogen-Doped Carbon to Boost Bifunctional Oxygen Electrocatalytic Activity, Adv. Funct. Mater., 2024, 2314444, DOI:10.1002/adfm.202314444.
- X. Xu, X. Li, W. Lu, X. Sun, H. Huang, X. Cui, L. Li, X. Zou, W. Zheng and X. Zhao, Collective Effect in a Multicomponent Ensemble Combining Single Atoms and Nanoparticles for Efficient and Durable Oxygen Reduction, Angew. Chem., Int. Ed., 2024, e202400765 CAS.
- W. Xu, R. Zeng, M. Rebarchik, A. Posada-Borbón, H. Li, C. J. Pollock, M. Mavrikakis and H. D. Abruña, Atomically Dispersed Zn/Co-N-C as ORR Electrocatalysts for Alkaline Fuel Cells, J. Am. Chem. Soc., 2024, 146, 2593–2603 CrossRef CAS PubMed.
- Q. Jin, C. Wang, Y. Guo, Y. Xiao, X. Tan, J. Chen, W. He, Y. Li, H. Cui and C. Wang, Axial Oxygen Ligands Regulating Electronic and Geometric Structure of Zn-N-C Sites to Boost Oxygen Reduction Reaction, Adv. Sci., 2023, 10, 2302152 CrossRef CAS PubMed.
- X. Guo, J. Shi, M. Li, J. Zhang, X. Zheng, Y. Liu, B. Xi, X. An, Z. Duan, Q. Fan, F. Gao and S. Xiong, Modulating Coordination of Iron Atom Clusters on N, P, S Triply-Doped Hollow Carbon Support towards Enhanced Electrocatalytic Oxygen Reduction, Angew. Chem., Int. Ed., 2023, 62, e202314124 CrossRef CAS PubMed.
- Y. Wang, N. Katyal, Y. Tang, H. Li, K. Shin, W. Liu, R. He, M. Xu, G. Henkelman and S. J. Bao, One-Step Pyrolysis Construction of Bimetallic Atom-Cluster Sites for Boosting Bifunctional Catalytic Activity in Zn-Air Batteries, Small, 2023, 20, 2306504 CrossRef PubMed.
- Y. Liu, S. Yuan, C. Sun, C. Wang, X. Liu, Z. Lv, R. Liu, Y. Meng, W. Yang, X. Feng and B. Wang, Optimizing Fe-3d Electron Delocalization by Asymmetric Fe-Cu Diatomic Configurations for Efficient Anion Exchange Membrane Fuel Cells, Adv. Energy Mater., 2023, 13, 2302719 CrossRef CAS.
- L. Cui, J. Hao, Y. Zhang, X. Kang, J. Zhang, X. Z. Fu and J. L. Luo, N and S dual-coordinated Fe single-atoms in hierarchically porous hollow nanocarbon for efficient oxygen reduction, J. Colloid Interface Sci., 2023, 650, 603–612 CrossRef CAS PubMed.
- C. X. Zhao, X. Liu, J. N. Liu, J. Wang, X. Wan, C. Wang, X. Y. Li, J. Shui, L. Song, H. J. Peng, B. Q. Li and Q. Zhang, Molecular Recognition Regulates Coordination Structure of Single-Atom Sites, Angew. Chem., Int. Ed., 2023, 62, e202313028 CrossRef CAS PubMed.
- L. Li, N. Li, J. W. Xia, S. L. Zhou, X. Y. Qian, F. X. Yin, G. H. Dai, G. Y. He and H. Q. Chen, A pH-universal ORR catalyst with atomic Fe-heteroatom (N, S) sites for high-performance Zn-air batteries, Nano Res., 2023, 16, 9416–9425 CrossRef CAS.
- L. Guo, S. Hwang, B. Li, F. Yang, M. Wang, M. Chen, X. Yang, S. G. Karakalos, D. A. Cullen, Z. Feng, G. Wang, G. Wu and H. Xu, Promoting Atomically Dispersed MnN4 Sites via Sulfur Doping for Oxygen Reduction: Unveiling Intrinsic Activity and Degradation in Fuel Cells, ACS Nano, 2021, 15, 6886–6899 CrossRef CAS PubMed.
- X. Xiong, Y. Li, Y. Jia, Y. Meng, K. Sun, L. Zheng, G. Zhang, Y. Li and X. Sun, Ultrathin atomic Mn-decorated formamide-converted N-doped carbon for efficient oxygen reduction reaction, Nanoscale, 2019, 11, 15900–15906 RSC.
- J. Li, M. Chen, D. A. Cullen, S. Hwang, M. Wang, B. Li, K. Liu, S. Karakalos, M. Lucero, H. Zhang, C. Lei, H. Xu, G. E. Sterbinsky, Z. Feng, D. Su, K. L. More, G. Wang, Z. Wang and G. Wu, Atomicallydispersed manganese catalysts for oxygen reduction in proton-exchange membrane fuel cells, Nat. Catal., 2018, 1, 935–945 CrossRef CAS.
- Y. Wang, X. Zhang, S. Xi, X. Xiang, Y. Du, P. Chen, D. Lyu, S. Wang, Z. Q. Tian and P. K. Shen, Rational Design and Synthesis of Hierarchical Porous Mn-N-C Nanoparticles with Atomically Dispersed MnNx Moieties for Highly Efficient Oxygen Reduction Reaction, ACS Sustainable Chem. Eng., 2020, 8, 9367–9376 CrossRef CAS.
- H. Xu, D. Wang, P. Yang, A. Liu, R. Li, Y. Li, L. Xiao, X. Ren, J. Zhang and M. An, Atomically dispersed M-N-C catalysts for the oxygen reduction reaction, J. Mater. Chem. A, 2020, 8, 23187 RSC.
- H. Xu, D. Wang, P. Yang, L. Du, X. Lu, R. Li, L. Liu, J. Zhang and M. An, A hierarchically porous Fe-N-C synthesized by dual melt-salt-mediated template as advanced electrocatalyst for efficient oxygen reduction in zinc-air battery, Appl. Catal., B, 2022, 305, 121040 CrossRef CAS.
- D. Xili, Q. Zhou and L. Zhang, Well-defined Co-N-C catalyst based on ZIF-67 in mixed solvents with low amount of ligands for efficient oxygen reduction reaction, J. Alloys Compd., 2022, 911, 165072 CrossRef CAS.
- L. Peng, J. Yang, Y. Yang, F. Qian, Q. Wang, D. Sun-Waterhouse, L. Shang, T. Zhang and G. I. N. Waterhouse, Mesopore-Rich Fe-N-C Catalyst with FeN4-O-NC Single-Atom Sites Delivers Remarkable Oxygen Reduction Reaction Performance in Alkaline Media, Adv. Mater., 2022, 34, e2202544 CrossRef PubMed.
- X. Lu, H. Xu, P. Yang, L. Xiao, Y. Li, J. Ma, R. Li, L. Liu, A. Liu, V. Kondratiev, O. Levin, J. Zhang and M. An, Zinc-assisted MgO template synthesis of porous carbon-supported Fe-Nx sites for efficient oxygen reduction reaction catalysis in Zn-air batteries, Appl. Catal., B, 2022, 313, 121454 CrossRef CAS.
- G. L. Li, Z. F. Lu, X. Wang, S. Cao and C. Hao, Rational Construction of Atomically Dispersed Mn-Nx Embedded in Mesoporous N-Doped Amorphous Carbon for Efficient Oxygen Reduction Reaction in Zn-Air Batteries, ACS Sustainable Chem. Eng., 2021, 10, 224–233 CrossRef.
- H. Jin, H. Zhou, D. He, Z. Wang, Q. Wu, Q. Liang, S. Liu and S. Mu, MOF-derived 3D Fe-N-S co-doped carbon matrix/nanotube nanocomposites with advanced oxygen reduction activity and stability in both acidic and alkaline media, Appl. Catal., B, 2019, 250, 143–149 CrossRef CAS.
|
This journal is © The Royal Society of Chemistry 2024 |