DOI:
10.1039/D3QM01202F
(Review Article)
Mater. Chem. Front., 2024,
8, 1685-1702
Nanozyme-enhanced ferroptosis for cancer treatment
Received
11th November 2023
, Accepted 16th January 2024
First published on 20th January 2024
Abstract
Ferroptosis is a programmed, iron-dependent, oxidative cell death that was discovered recently. It is usually accompanied by iron accumulation and lipid peroxidation during the cell death process. Ferroptosis-inducing factors affect glutathione (GSH) peroxidase directly or indirectly, leading to a decrease in antioxidant capacity and accumulation of lipid reactive oxygen species (ROS). Ferroptosis has garnered much interest in the field of cancer treatment. However, the therapeutic efficacy through the ferroptosis pathway by directly increasing the levels of iron ions at cancer lesion is not ideal due to the inefficient enrichment of iron ions at the lesion site, the uncontrolled Fenton reaction and a single apoptotic pathway. Nanozymes are nanomaterials that can catalyse enzyme substrates into products following enzyme kinetics under physiological conditions. Nanozymes offer advantages such as enhanced stability, simplified preparation, and cost-effectiveness compared to natural enzymes. Notably, nanozymes can serve as self-activated cascade reagents, elevating the therapeutic efficacy of cancer through the ferroptosis pathway by effectively generating reactive ROS and depleting GSH. Furthermore, nanozymes can induce ferroptosis and synergize with other approaches such as photothermal therapy (PTT), photodynamic therapy (PDT), and immunotherapy. Presented in this review are the definition, structure, classification, and features of nanozymes, the fundamental mechanisms of ferroptosis in cancer cells, and the combined strategies employed to combat cancer by leveraging nanozymes to induce or enhance ferroptosis.
1. Introduction
Cancer refers to a group of diseases characterized by the uncontrolled growth and spread of abnormal cells. The Global Cancer Observatory estimated nearly 1.93 billion new cancer cases and approximately 10 million cancer-related deaths worldwide in 2020.1 The most common types of cancer are breast cancer, lung cancer, colorectal cancer, prostate cancer, and stomach cancer.2 These statistics indicated the cruciality to deeply understand cancer and develop suitable diagnosis and treatment options. Traditional methods for cancer treatment include surgery, radiation therapy, and chemotherapy, which have limitations such as high recurrence rates, poor postoperative quality of life, and lack of target specificity.1 Given the current limitations in precision cancer treatment, there is an urgent need for new anticancer approaches.3
Cancer treatment based on ferroptosis can target cancer cells, including those that are resistant to drugs. The iron sensitivity of different types of cancer cells provides the basis for this therapy. By controlling the iron metabolism and lipid peroxidation,4 or inhibiting glutathione (GSH) synthesis or glutathione peroxidase 4 (GPX4) activity, this therapy can selectively trigger the iron dependency of cancer cells without adversely affecting healthy cells.5 Prospects for iron-based inducers are expanding, including erastin, GPX4 inhibitors, and iron chelators.6 Ferroptosis, distinct from traditional forms of cell death such as apoptosis, necrosis, and autophagy, is a regulated form of cell death driven by iron-dependent lipid peroxidation.7 Its essence lies in the depletion of GSH and the decrease in the activity of GPX4, leading to the inability of lipid peroxides (LPO) to be metabolized through the GPX4-catalysed GSH reductase reaction. Subsequently, the oxidation of lipid precursors by ferrous iron produces reactive oxygen species (ROS), thereby triggering ferroptosis. This can serve as a novel anticancer method.
Currently, cancer treatment methods based on iron-induced cell death are actively explored. Non-targeted strategies based on nanoparticle delivery to transport iron, peroxides, and other toxic substances have been proposed to kill cancer cells.8 The existence of various enzymes that regulate ferroptosis makes the development of targeted therapy possible.9 Nanozymes are nanomaterials with enzyme-like characteristics, possess good stability, low cost, and adjustable catalytic activity. Recently, their role in inducing ferroptosis in cancer treatment has attracted much attention.10 For example, nanozymes were designed to encapsulate or carry anticancer drugs or molecules that influence the ferroptosis pathway, and then delivered to specific cancer cells.11 This further enhanced the selectivity of ferroptosis in cancer cells and minimized the damage to healthy cells.
The process of ferroptosis relies on lipid peroxidation, where the Fenton reaction is a key pathway. The Fe2+ ions react with H2O2 to generate hydroxyl radicals, leading to further lipid peroxidation and cell death.12 Nanozymes can effectively catalyse the Fenton reaction or mimic the functions of natural enzymes such as peroxidase (POD), superoxide dismutase (SOD), catalase (CAT), and oxidase (OXD). They regulate the balance between the generation and elimination of ROS, thereby controlling the level of lipid peroxidation to influence the process of ferroptosis (Fig. 1).13
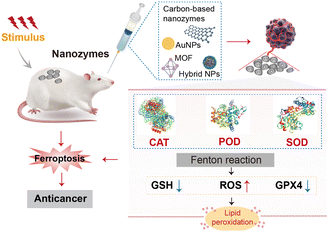 |
| Fig. 1 An overview of synergistic mechanisms of nanozymes and ferroptosis. The diagram demonstrates that nanozymes mimic the catalytic activity of diverse natural enzymes (including structural modeling of CAT/POD/SOD-like enzymes), thus stimulating the Fenton reaction. This stimulation leads to the production of reactive oxygen species, GSH and GPX4 depletion, and lipid peroxidation, ultimately inducing ferroptosis to exert anticancer effects. | |
More and more preclinical studies have shown that many cancer cells have developed resistance to traditional therapies such as chemotherapy14 and radiation.15 However, these conventional mechanisms of drug resistance are often unable to counteract ferroptosis. The employment of nanozymes as a mean to trigger ferroptosis provides an alternative strategy to eradicate previously challenging-to-treat cancer cells. Three main pathways to reverse drug resistance include the canonical GPX4-regulated pathway, iron metabolism pathway, and lipid metabolism pathway. Nanozymes can be designed to achieve precise targeting of specific cells or tissues for precision medicine in cancer treatment. Additionally, nanozymes can be combined with other anticancer drugs or molecules that affect the pathways of ferroptosis, serving as drug delivery systems for cancer treatment. Compared to natural enzymes,16 nanozymes have lower production costs, and their excellent stability and adjustability contribute to their potential benefits and feasibility in future cancer treatments. Thus, nanozyme-triggered ferroptosis to induce cancer cell death shows great potential as an innovative anticancer method.
2. Design and synthesis of nanozymes
2.1 Definition of nanozymes
Nanozymes first appeared in the literature in 2004, gained development in 2007 with Yan's discovery of peroxidase-like activity in magnetic iron oxide (Fe3O4) nanoparticles.17 Nanozymes refer in a broad sense to nanoparticles possessing catalytic functions similar to natural enzymes.18 However, enzymes and nanozymes exhibit significant mechanistic differences. For example, peroxidases confine activated H2O2 to the active center, while iron oxide is likely to exert more ROS-related toxicity (Fig. 2).19 Enzymatic reactions are highly substrate-selective, whereas nanozymes can exhibit broader substrate specificity than their natural counterparts.20
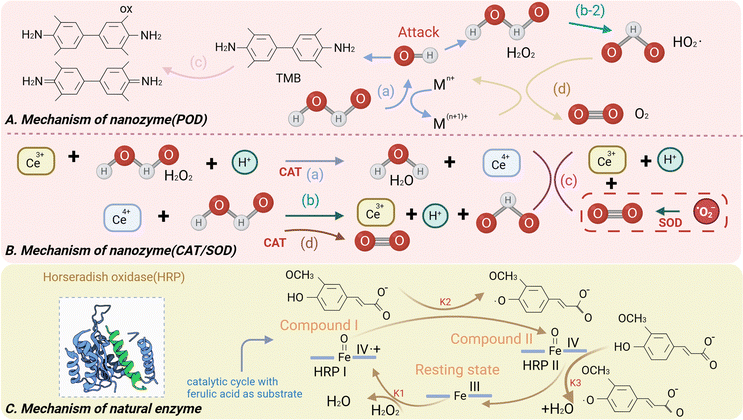 |
| Fig. 2 The catalytic mechanism of (A) POD activity by nanozymes with Fe3O4 as the model; (B) CAT/SOD activity by nanozymes with cerium oxide (CeO2) as the model; and (C) Natural enzyme with horseradish peroxidase (HRP) as the model. | |
Nanozymes are catalytic nanomaterials, but their functionality extends beyond catalysis. In addition to their catalytic activity, nanozymes can possess other properties and functionalities that make them versatile and valuable in various applications, including target recognition,21 specific binding,22 controlled release and so on.23
2.2 Classification of nanozymes
Existing reports on nanozymes are mainly focused on simulating the activity of oxidation–reduction enzymes,24 including SOD activity that generates H2O2 and oxygen (O2) from a superoxide anion (O2−), CAT activity that decomposes H2O2 to produce H2O and O2, POD activity that catalyses the redox of H2O2 substrates, the oxidoreductase activity that directly oxidizes substrates in the presence of O2, some reductase activities, etc.25 Among them, SOD, CAT, and POD, as natural antioxidants in the body, play a vital role in clearing cellular oxygen-free radicals and maintaining the normal oxidative-reduction level of the organism. Additionally, anaerobic glycolysis, also known as the “Warburg effect”,26 is one of the inherent markers of cancer metabolism, characterized by high levels of glucose intake and an increased rate of glucose conversion to lactate. Tumour tissues exhibit an acidic microenvironment and are highly sensitive to fluctuations in glucose content. Inspired by this characteristic, glucose oxidase (GOD) has been employed to serve as the nutritional source of cancer cells in tumour starvation treatments.27 In this section, we summarize the mechanisms and kinetics of typical nanozymes with unique catalytic activities. We analyse the catalytic mechanisms of different types of nanozymes (Table 1).
Table 1 Classification of nanozymes
Nanozymes |
Catalytic activitiesa |
Ref. |
SOD: superoxide dismutase; CAT: catalase; OXD: oxidase; POD: peroxidase.
|
Iron oxide |
POD |
41
|
Gold |
CAT and POD |
42
|
Platinum |
POD, OXD, and SOD |
42
|
CeO2 |
OXD and SOD |
43
|
Copper |
OXD |
44
|
Manganese |
OXD, CAT, and POD |
45
|
Carbon |
POD |
46
|
POD-like activity.
Fe3O4 was the first nanozyme which catalysed the reaction of peroxides, converting H2O2 to O2 and hydroxyl radicals.28 The experimental data suggest that the catalysis mechanism of nano Fe3O4 may follow the “ping-pong reaction mechanism”. As shown in Fig. 2A, Fe3O4 can combine with the first substrate H2O2 to produce the intermediate ˙OH. Then, the generated ˙OH captures an H+ from a hydrogen donor such as 3,3′,5,5′-tetramethylbenzidine. Subsequently, through combined electron spin resonance measurements and free radical inhibition experiments,29 Tang et al. proposed a possible catalytic mechanism for peroxidase analogues based on Fe3O4 nanozymes, and monitored the production of the intermediate ˙OH in the catalytic reaction using electron spin resonance.30 The study showed that Fe3O4 NPs can also produce the intermediate ˙OH, indicating that they have similar properties to peroxides.31
CAT-like and SOD-like activities.
Cerium dioxide (CeO2) NPs, due to their unique chemical and physical properties, are among the most widely studied nanomaterials. They exhibit enzyme-like activity, especially for CAT and SOD.32,33 CeO2 NPs are primarily associated with the oxidation state of their surface, which simultaneously includes Ce3+ and Ce4+ sites, granting them the SOD and CAT activities. Therefore, by manipulating the redox state of cerium, CeO2 NPs can simulate the dual antioxidant enzymes of SOD and CAT (Fig. 2B). Engaging in redox cycling reactions,34 they regenerate the initial oxidation state and regulate ROS levels. A lower Ce3+/Ce4+ ratio favours the simulation of CAT-like activity in CeO2 NPs, reducing Ce4+ to Ce3+ while catalysing the decomposition of H2O2 into O2. After generating hydrogen peroxide, CeO2 NPs can utilize their SOD-like activity to simulate CAT-like activity. Following adsorption onto the CeO2 surface, H2O2 preferentially dissociates, releasing two protons and O2, and simultaneously reducing Ce4+ to Ce3+. Then, another molecule of H2O2 is absorbed and combines with the newly released protons to form two molecules of water, during which Ce3+ is re-oxidized to Ce4+.35
GOD-like activities.
Compared to natural GOD, nanozymes mimicking GOD offer higher catalytic stability, easier modifications, and lower production costs for tumour treatment. Currently, the use of GOD-mimicking nanozymes in cancer therapy primarily includes substances like AuNPs,36 and manganese dioxide (MnO2)37 and carbon nitride (C3N4) nanomaterials. Other materials also include metal–organic frameworks (MOF), covalent-organic frameworks (COF),38 mesoporous silica or hollow black TiO2 nanoparticles.39 Much like their natural GOD counterpart, nanozymes, such as AuNPs, catalyse glucose oxidation by dehydrogenating glucose and reducing O2 through a two-electron mechanism, resulting in the production of gluconate and H2O2.40
2.3 Strategies for improving the catalytic activity and specificity of nanozymes
So far, few nanozymes have matched the effectiveness of their natural enzyme counterparts. Usually, an enzymatic reaction follows a three-step process where a substrate attaches to the enzyme's active site, undergoes transformation into a product and then is released – making way for the next substrate. This mechanism holds true for nanozymes as well, but the reaction happens on the nanomaterial's surface. The substrate binds via adsorption on the nanozyme.47 The key characteristics affecting the catalytic activity of nanozymes include: (1) molecular structure: nanozymes possess a unique and often complex molecular structure, which generally contains active sites or centers that facilitate catalytic activity;35 (2) electron properties: the ways in which electrons are arranged and behave in nanozymes significantly contribute to their catalytic activities;48 and (3) large surface area: the nanoscale size increased surface area allows for more active sites.49 By optimizing each stage of the catalytic cycle, it is possible to narrow the performance gap between nanozymes and natural enzymes.50
Size and shape optimization.
By adjusting the size and shape of nanozymes, one can control the enzymatic activity, stability, and specificity.35 For example, Fan et al. proposed that the GOD-like catalytic activity of AuNPs is size-dependent. By comparing the reaction rates of AuNPs with different particle sizes (13, 20, 30, and 50 nm) under the same conditions, it was found that the catalytic performance of AuNPs decreased with increasing particle size. In addition, shape modification (e.g., nanoflowers) of the nanozymes can endow them with a high density of spikes, good optical properties, and larger surface-to-volume ratios, which can enhance the catalytic activity, adsorption, and loading capacity of the nanozymes.51
Surface modification.
By modifying the surface of nanozymes with specific functional groups or targeting ligands, their catalytic activity and specificity for cancer cells can be increased. For example, the same surface charge of the modified materials can affect the catalytic activity of the nanozymes similar to POD. The positively charged PLL (poly-lysine) and PEI (polyethyleneimine)-modified Fe3O4 nano-enzymes demonstrated higher catalytic activity when anionic 2,2'-azinobis-(3-ethylbenzothiazoline-6-sulphonate) was used as the substrate.52
Environment-responsive design.
Nanozymes are able to modulate the tumour physiological environment, including pH, O2 levels, and immunosuppressive tumour cells, reprogramming the immunosuppressive tumour microenvironment (TME).45 Ideally, nanozymes should also induce immunogenic cell death (ICD) of tumour cells, releasing tumour-associated antigens, which can be trapped, processed, and presented by antigen-presenting cells (APCs), thereby increasing the response rate and therapeutic efficacy of the immune system and immunotherapeutic drugs.53
Composition tweaking.
CeO2 nanozymes exhibit excellent CAT-like activity and O2 production capacity, while AuNPs exhibit more GOD-like activity and detection ability.54 Changing the material compositions of the nanozymes could help in modulating and increasing the catalytic activity.
Hybrid enzyme design.
Designing hybrid nanozymes that combine the properties of different enzymes could offer enhanced catalytic activity or specificity.55 Sun et al. prepared OAm-PEG modified Pt48Pd52-Fe3O4 nanocomposites with a dumbbell-like morphology. The Pt48Pd52-Fe3O4/TMB kit was used for the detection of H2O2 in biological solutions with a detection limit as low as 2 μM, and was successfully used for the quantitative monitoring of extracellular H2O2 produced by neutrophils. Compared with single Pt, Pd and Fe3O4 NPs, the obtained Pt48Pd52-Fe3O4 hybrid nanomaterials have higher peroxidase-like activity compared to single Pt, Pd and Fe3O4 NPs.56
By implementing these strategies, the catalytic activity and specificity of nanozymes can be tuned to achieve the desired therapeutic outcomes in cancer treatment.
3. Mechanisms of nanozyme-induced ferroptosis
Ferroptosis is a prevalent form of cell death that occurs as a result of lipid peroxidation, which is initiated by hydroxyl radicals generated in the iron-catalysed Fenton reaction. Lipid peroxidation involves the oxidative degradation of lipids within the cell membrane, with a particular impact on polyunsaturated fatty acids (PUFAs). The resulting LPO species critically disturb the stability of the cell membrane's structure and functionality, ultimately leading to cellular damage and demise.11
In the cellular environment, an excessive quantity of iron is initially produced, and the entry of Fe3+ into the cell occurs via the transferrin receptor 1 (TFR1) located on the cell membrane. Fe2+ was reduced within the cell, creating an unstable iron pool. Peroxidation of PUFA-containing membrane lipids is facilitated by both an unstable iron pool, which promotes the Fenton reaction and subsequent lipid peroxidation, and enzymes that utilize iron as a cofactor, such as arachidonic acid lipoxygenase (ALOX), which triggers the formation of lipid hydroperoxides, serving as substrates for the Fenton reaction.57
Accumulating evidence regarding the mechanism of LPO and GPX4 enzymology indicates that LPO is triggered by alkoxyl radicals generated through the action of ferrous iron on lipid hydroperoxide derivatives (LOOH), which are unavoidable byproducts of aerobic metabolism. These LOOH molecules are continuously reduced to LOH by GPX4 in the presence of reduced GSH. However, when the activity of GPX4 is limited, such as in the case of GPX4 inhibition or GSH depletion, ferrous iron triggers LPO by catalysing the breakdown of LOOH, ultimately leading to iron-mediated cell death.58 The significance of LPO in ferroptosis is further supported by biological studies. For instance, the presence of LPO products, such as hydroxynonenal and malondialdehyde, has been detected during ferroptosis. Although the precise quantitative evidence for LPO in ferroptosis is still limited, the observed link between these two phenomena is compelling. We emphasize here the concept of thresholds, which are surpassed when the essential constraints of LPO are fulfilled, the activity of GPX4 is insufficient, and ferroptosis develops. LPO has been identified as a reliable indicator of ferroptosis.59
The regulation of ferroptosis is primarily controlled by the GSH/GPX4 pathway. GSH, a crucial intracellular antioxidant, plays a vital role in impeding iron metabolism by effectively eliminating LPO through the catalytic activity of GPX4. GPX4 is selenium-containing protein abundantly present in mammalian cells, serving as an enzyme that neutralizes LPO and providing protection. It efficiently converts the substrate GSH to GSSG while reducing intracellular toxic LPO to non-toxic alcohols (L-OH).61 Additionally, GPX4 effectively converts free H2O2 to water, safeguarding the structural integrity and functionality of cell membranes against peroxides, interference and damage.
Commonly used inducers of ferroptosis, such as erastin or Ras selective lethal small molecule 3 (RSL3, Fig. 3), can inhibit the activity of GPX4, thereby diminishing the antioxidant capacity of cells and triggering lipid reactive oxygen species (ROS), ultimately leading to ferroptosis.62 Furthermore, the mevalonate (MVA) pathway influences GPX4 synthesis by regulating the maturation of selenocysteine tRNA, which in turn controls the onset of ferroptosis.
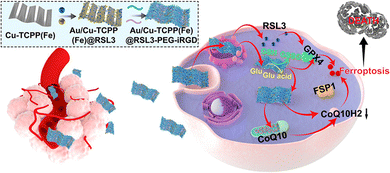 |
| Fig. 3 Schematic illustration of a composite nanosheet designed for tumour targeting, with the aim of sensitizing tumour ferroptosis through the disruption of the GPX4/GSH and FSP1/CoQ10H2 pathways.60 Reproduced with permission from ACS Publications, copyright 2022. | |
Cysteine is closely associated with the glutamate transporter (system Xc−, an essential antioxidant system widely distributed in the phospholipid bilayer), another important mechanism for inducing ferroptosis. System Xc− is a heterodimer composed of two subunits, solute carrier family 7 member 11 (SLC7A11) and SLC3A2. It facilitates the exchange of cystine and glutamate in and out of the cell in a 1
:
1 ratio.63 Cystine, an oxidized form of cysteine, is transported into the cell via system Xc− and subsequently reduced to cysteine. Cysteine plays a crucial role in the synthesis of GSH, an intracellular antioxidant.58 Erastin can inhibit cystine transport causing cells, which leads to the depletion of cysteine (the fundamental component of intracellular GSH) indirectly inducing ferroptosis.59
Recent studies have also shown that immune cells in the tumour microenvironment determine the occurrence of ferroptosis in tumours. Arachidonic acid and interferon-γ (IFN-γ) released by CD8+ T cells have been identified as potential natural triggers of ferroptosis. Cytotoxic T cells (CTL. i.e., CD8+ T cells) downregulate the expression of SLC7A11 and inhibit the uptake of cystine in cancer cells by secreting IFN-γ. Cystine participates in the synthesis of GSH, the main process which involves system Xc− taking extracellular cystine into cells and exporting glutamate outside the cells.64 After entering the cell, cystine is rapidly reduced to cysteine, which subsequently contributes to the formation of GSH. Several studies have confirmed a positive correlation between the occurrence of ferroptosis and immune-related pathways. The findings indicate that higher expression levels of HLA, as well as increased infiltration of CD8+ T cells and tumour infiltrating lymphocyte, are associated with ferroptosis (Fig. 4).66
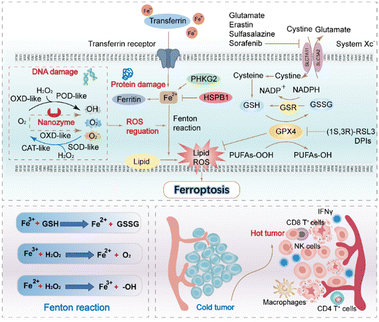 |
| Fig. 4 The classical GPX4 mechanism of ferroptosis and the synergistic role of nanozymes are illustrated in a schematic chart depicting the canonical ferroptosis control axis. This axis involves several key steps, including the uptake of cystine via the cystine-glutamate antiporter, known as system Xc−. Cystine is then reduced to cysteine in a GSH-dependent manner.57 The biosynthesis of GSH and the GPX4-mediated reduction of phospholipid hydroperoxides (PL-OOH) to corresponding alcohols (P-LOH) are also part of this axis. The recycling of oxidized glutathione (GSSG) is achieved through glutathione-disulfide reductase (GSR), utilizing electrons provided by NADPH/H+. The figure also illustrates the regulatory role of nanozymes in the process of ferroptosis, particularly their ability to facilitate Fenton reactions through various classes of enzyme catalytic activities. Additionally, the chart describes the immune effects induced by ferroptosis, such as the activation of various immune cells and the conversion of “cold” tumours to “hot” tumours, among other immune-related phenomena.65 | |
4. Nanozymes play an intrinsic catalytic role in solid tumours
In the treatment of solid tumours such as lung and breast cancers, nanozyme-triggered ferroptosis has been utilized as a new strategy. The reasons for this include: (1) high demand for iron by cancer cells: cancer cells usually require many irons to support their rapid growth and division, which makes them more dependent on iron ions and more susceptible to ferroptosis. Nanozymes can catalyse these iron ions, triggering a more intense ferroptosis effect that kills solid tumour cells.67 (2) Integral compatibility: While causing ferroptosis in cancer cells, the impact on regular healthy cells can be controlled to a certain extent, especially with some highly selective and targeted nanozymes. (3) Resistance to treatment: Some cancers have evolved resistance to therapeutic treatments by way of resistance to conventional programmed cell death (e.g., autophagy).68 Since ferroptosis is an iron-dependent programmed necrosis, it may ignore these resistance mechanisms and still effectively kill cancer cells.69 For some cancer cells that have become resistant to conventional chemotherapy or radiotherapy, nanozyme-triggered ferroptosis may be an effective alternative or adjuvant therapeutic strategy. The cancers currently being treated using nanozyme-triggered ferroptosis are described below.
An et al. prepared a MnO2@HMCu2−xS nanocomposite (HMCMs).70 Mn2+ release based on GSH response can generate ROS via the Fenton reaction, which enhances intracellular oxidative stress and leads to LPO accumulation (Fig. 5). With the introduction of photothermal therapy, the GSH-consuming capacity of HMCM could also be further enhanced to induce iron phagocytosis for efficient tumour ablation.
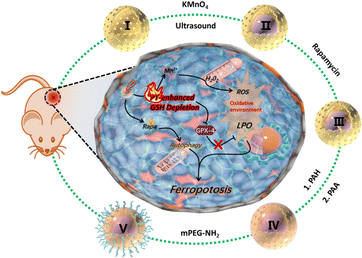 |
| Fig. 5 Sequential synthesis process of HMCMR nanocomposites, depicted in the out ring. The central portion of the figure demonstrates a schematic representation of the mechanism involving photothermal (PT) effects and autophagy enhancement, leading to the promotion of iron mutagenesis facilitated by the HMCMR nanocomposites.70 Reproduced with permission from ACS Publications, copyright 2019. | |
However, the inefficient conversion of Fe3+ to Fe2+ during the Fenton reaction of ferroptosis in tumour environments often leads to the blockage of the whole reaction process and the reduction of catalytic efficiency (Table 2). To improve the catalytic efficiency of the Fenton reaction in ferroptosis, Jiang et al. developed a hybrid semiconducting nanozyme with high photothermal conversion efficiency for photoacoustic imaging-guided second near-infrared photothermal ferrotherapy.78 Such semiconducting polymer NPs composed of highly π-conjugated backbones can act as iron chelators. Their chelating ability is derived from the high binding affinity for ferrous ions of the main chain sulfur and nitrogen atoms, enhancing the Fenton reaction to enhance apoptosis and ferroptosis in A549 cells in vitro and in vivo. To address the hypoxia-limited nature in tumour therapy, some peroxidase-like nanozymes with intrinsic catalytic ability have been widely studied, such as biodegradable boron oxynitride,79 CeO2,80 TiO2,79 and FeS2.81
Table 2 Factors influencing the Fenton reaction
Factors |
Characteristics |
Ref. |
pH |
The optimum pH is usually between 3.0 and 4.0 |
71
|
Concentration of reactants |
Concentrations of iron ions or hydroxide ions shouldn’t be too low or too high |
72
|
Temperature |
Temperature (not too high) is positively correlated with the reaction rate |
73
|
Concentration of H2O2 |
Higher H2O2 concentration promotes hydroxyl radical formation |
74
|
Light |
Light stimulation affects free radical production and activity |
75
|
Electronic field |
Electric field inducing cavities for ROS regeneration |
76
|
X-ray |
Nano-radiosensitizers can enhance energy absorption and deposition for escalating ROS generation |
77
|
Furthermore, nanozymes exhibit the capacity to modulate iron-related proteins and genes within cellular systems, consequently influencing iron metabolism, a pivotal process underlying the occurrence of ferroptosis. Wang et al. highlighted an interesting finding regarding the conserved sequence HEXXH in the fifth structural domain of the Solute Carrier Family 39.82 In this case, the conserved sequence was replaced by EEXXH, which imparts the function of transporting divalent metal ions such as Mn2+, Fe2+, or Zn2+. This transporter is involved in the cellular ferroptosis process by facilitating the transport of Fe2+. Nanozymes have the potential to increase iron accumulation in cells by increasing iron uptake or limiting its export, thereby increasing cellular sensitivity to ferroptosis. Overall, nanozymes in cancer treatment showed that in addition to the transport ability of traditional nanomaterials, they played a catalytic role in the body's own biochemical reactions, which successfully improved the catalytic efficiency of the Fenton reaction in ferroptosis and overcame the deficiencies against hypoxia in chemotherapy, PTT, and PDT, resulting in a significant increase in the efficiency of lung cancer treatment. This feature can also be seen in ferroptosis-based therapy for other types of tumours (Table 3).
Table 3 Nanozymes for treating various types of tumoursa
Tumour type |
Components |
Characteristics |
Ref. |
TCPP(Fe): tetra(4-carboxyphenyl)porphyrin chloride(Fe(III)); MIL: materials of institut lavoisier; SAzyme: single-atom nanozyme; DAzyme: dual-metal atom nanozyme; Lipo: liposome; NAC: N-acetyl-L-cysteine.
|
Lymphoma |
Fe2O3/Au nanozymes |
Catalysing the production of gluconic acid and H2O2 to induce ferroptosis |
83
|
Au/Cu-TCPP(Fe) nanosheets |
Inhibiting GPX4- and FSP1-mediated anti-ferroptosis mechanisms; reducing GSH synthesis and GPX4 expression |
84
|
Pt-MIL-101 (Fe) |
Oxidizing NADPH and subsequent cascade catalytic with nanozymes; generating hydroxyl radicals and blocking GSH regeneration |
85
|
PtN4C-SAzyme |
Self-sufficiency of O2 through multi enzyme-like catalytic activity |
86
|
Manganese-based nanozymes |
Depleting GSH and inhibiting GPX4 activity due to LPO accumulation; activating the cGAS-STING pathway |
87
|
MOF(Fe) |
GPX4 depletion and elevated LPO; enhancing SDMD cleavage and elevating the expression of IL-1β and LDH |
88
|
FeCo/Fe–Co DAzyme |
Activating IFN-γ and targeting arachidonic acid metabolism |
89
|
Glioma |
Au/CeO2 nanoparticles |
Inducing ferroptosis through GSH inhibition and ROS generation; exerting efficient blood–brain barrier permeability and glioblastoma localization |
90
|
Magnetic nanoparticles |
Combining triple actions of dihydroorotic acid dehydrogenase catabolism; GPX4-ferroptosis defense axis with Fe3O4 nanoparticle-mediated Fe2+ release |
91
|
Lung cancer |
Ru nanozymes |
Generating NO to co-activate macrophage M1 polarization |
92
|
CuCP Lipo nanoparticles |
Eliminating GSH from the tumour mass and producing a large amount of LPO; inhibiting tumour antioxidant response through modulation of the tumour microenvironment |
93
|
Colon |
MoS2 nanoparticles |
Self-supply of H2O2; integrating charge-enhanced enzyme activity; dysregulating redox homeostasis via GSH depletion |
94
|
Liver cancer |
Carbon quantum dots |
Promoting cancer cell ferroptosis by perturbating the GPX4-catalysed lipid repair system; activating systemic antitumor immune response |
95
|
Cu-Hemin nanosheets |
Up-regulating HMOX1 expression and down-regulating GPX4 expression |
96
|
Pancreatic cancer |
NAC-RuO2 nanozymes |
Depleting GSH and simultaneous generating ONOO; down-regulating GSH reductase to avoid GSH regeneration |
97
|
5. Synergistic therapies involving nanozymes in cancer treatment
Nanozymes have a broader range of applications in cancer therapy compared to natural enzymes. They possess inherent biological enzyme catalytic properties and can be further enhanced by combining them with natural enzymes or modifying them with enzyme-like active groups on nanomaterials, resulting in improved targeting, stability, and durability. Moreover, nanozymes offer high controllability, convertible enzyme activities, and multiple enzyme activities, leading to enhanced effectiveness in targeted therapy, immunotherapy, radiotherapy, and other cancer treatment modalities (Fig. 6). The following are some combination strategies.
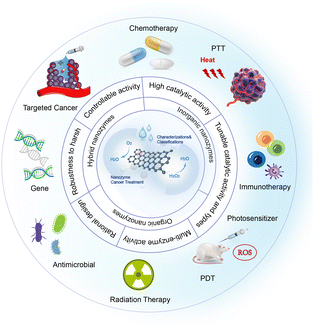 |
| Fig. 6 The figure visually represents the synergistic antitumor effects achieved by integrating nanozymes with diverse therapeutic strategies. Additionally, the figure highlights the advantages and categorization of nanozymes in the context of tumour treatment.102 | |
5.1 Chemotherapy
Conventional chemotherapy is associated with limitations such as inadequate targeting and the development of tumour resistance. Therefore, targeted therapy which can precisely combat cancer has been a promising research direction.98 Nanozymes can be engineered to incorporate specific ligands or antibodies that selectively target molecular markers on cancer cells, thereby improving their specificity and minimizing off-target effects on healthy cells.99
Dong et al. developed a Cu–Ag alloy nanozyme loaded with banoxadone hydrochloride (AQ4N) (Fig. 7).100 The Cu–Ag nanozyme acted as a catalyst, facilitating the reduction of oxygen to cytotoxic superoxide (˙O2−), which could intensify tumour hypoxia and activate AQ4N, a highly selective chemotherapeutic drug for cancer therapy. Additionally, the production of hydroxyl radicals (˙OH) induced by the Cu–Ag nanozyme triggered ferroptosis in tumour cells by promoting LPO accumulation and inhibiting GPX4. More importantly, the experiment did not reveal any off-target toxicity. This study effectively combines principles from starvation, chemotherapy, and ferroptosis, thereby enhancing the specificity of chemotherapy treatment.
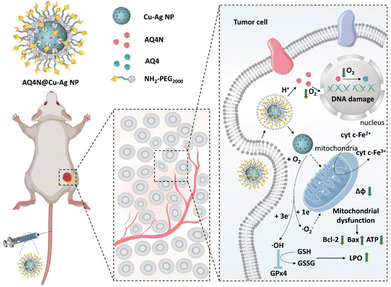 |
| Fig. 7 Utilization of cytochrome c oxidase-like nanozymes, comprising copper–silver alloy nanoparticles (Cu–Ag NPs), as vehicles for delivering chemotherapeutic drugs (AQ4N). The objective is to employ a targeted strategy that combines concurrent starvation therapy, iron-induced cell death, and chemotherapy, with the aim of enhancing the efficacy of anticancer treatment.100 Reproduced with permission from John Wiley and Sons, copyright 2022. | |
Shen et al. conducted a modification of transferrin on a MOF to enhance the targeting of specific sites, thereby promoting iron-induced cell death.101 This modification resulted in an increased anticancer effect through the augmentation of cysteinyl asparagine-1-mediated GSDMD cleavage, as well as the elevation of IL-1β levels and the release of lactate dehydrogenase (LDH).101
In the context of ferroptosis-mediated reversal of chemotherapy resistance, three main pathways have been identified: the canonical GPX4-regulated pathway, iron metabolism pathway, and lipid metabolism pathway. Fu et al. provided evidence that inhibiting Nrf2/Keap1/xCT signalling to induce ferroptosis effectively sensitized cisplatin-resistant gastric cancer cells to cisplatin treatment.99 Similarly, PARP inhibition promotes ferroptosis by inhibiting SLC7A11-mediated GSH synthesis, and synergistically sensitizes xenografts to the PARP, which reverse intrinsic resistance. In addition, CD8+ T cells play a role in inducing cellular focal death by activating caspases, which subsequently cleave GSDMB. The secretion of IFN-γ by CD8+ T cells down-regulates SLC7A11, disrupting the cellular antioxidant system and leading to the accumulation of lipid ROS, thereby inducing ferroptosis.67 Clinically, the induction of ferroptosis has shown promise as a therapeutic approach to effectively inhibit the development of drug resistance, including resistance to drugs such as erlotinib and trametinib. These findings hold promise for the development of novel therapeutics by inducing ferroptosis for overcoming drug resistance in cancer. After sophisticated design based on the structure–activity relationship, nanozymes can exert powerful antitumor effects with ferroptosis as well as chemotherapeutic drugs, not only catalytic effects, but also nanozymes are potentially promising as drug carriers.
Targeted drugs are developed to target oncogenes, which identify characteristic sites on tumour cells determined by genes specific to the tumour cells. The study of different oncogene profiles can help to target tumours more precisely. Ferroptosis genes play important roles in different cancers. Ferroptosis genes are expressed at different levels in different cancers with different expression models and prognosis, including ACSL4, GPX4, SLC7A11, RAS, TP53, etc.
In addition to the classical GPX4 pathway, in 2019 scientists discovered an enzyme catalytic system independent of the classical GPX4 signalling pathway, a pathway based on the ferroptosis suppressor FSP1.103 And the iron sinking mechanism similar to water–oil separation was developed to avoid FSP1 inhibitors from exhibiting off-target effects in vivo. By triggering FSP1 phase separation, subcellular repositioning of FSP1 can be achieved, ultimately leading to the presence of distinct FSP1 agglutinates in tumour tissues, and significantly inhibited tumour growth.104
SLC7A11, as a major acting component of system Xc−, is highly expressed in a variety of solid malignant tumours, such as breast cancer, pancreatic cancer, and glioma, among others. Erastin, a classical ferroptosis promoter, can target SLC7A11, induce ferroptosis and reverse colorectal cancer drug resistance by activating the GPX4 regulatory pathway. Knockdown of SLC7A11 increases ROS levels and decreases GSH levels to promote apoptosis (Fig. 8).105
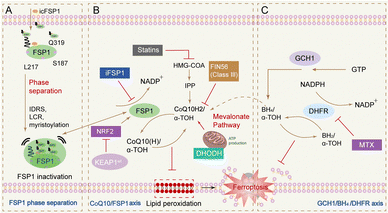 |
| Fig. 8 Ferroptosis pathway independent of GPX4 pathways. (A) Graphical abstract depicting icFSP1-induced FSP1 condensate formation, lipid peroxidation and ferroptosis;104 (B) FSP1/ubiquinone (CoQ10) system has been recently identified to completely protect against ferroptosis induced by pharmacological inhibition or genetic deletion of GPX4. FSP1 prevents lipid peroxidation and associated ferroptosis via reduction of ubiquinol/α-tocopherol on the level of lipid radicals unlike GPX4/GSH;111 (C) alternate ferroptosis-suppressive mechanisms include squalene- and di-/tetrahydrobiopterin (BH2/BH4)-mediated inhibition of lipid peroxidation.112 | |
5.2 Immunotherapy
Tumour immunotherapy aims to enhance the body's immune system to directly or indirectly eliminate and control cancer by mobilizing immune cells capable of recognizing tumours. Tumour cells often possess low immunogenicity as a means to evade immune cell recognition.106 The interaction between ferroptosis and immune cells plays a crucial role, as ferroptosis can impede the immune system's ability to combat pathogenic bacteria by reducing the populations of T cells and B cells. Nanozymes have emerged as effective modulators of the TME, capable of enhancing immunotherapy through diverse mechanisms such as macrophage polarization, antigen presentation, and activation of cellular pathways.107
Zeng et al. proved that polyethylene glycol (PEG)-denatured Cu-doped polypyrrole nanozyme (CuP) effectively reversed the immunosuppressive TME by overcoming tumour hypoxia and polarizing macrophages from a tumour-promoting M2 phenotype to an antitumor M1 phenotype.108 Due to the high temperature enhancement, the catalytic activity can be further enhanced with the addition of a 1064 nm laser, which helps to promote macrophage polarization. The modification of PEG can provide a hydration layer that hinders the formation of protein corona avoiding phagocytosis of the nanozyme.109 Meanwhile, Zhao et al. designed a tumour cell membrane camouflaged multi-enzyme activity mimicking manganese oxide nanozymes (CM@Mn) to provide a biomimetic surface,110 further prolonging the in vivo circulation of the nanoparticles. Through their inherent POD-like and OXD activities, they generate ˙OH and O2− to kill tumour cells and trigger immunogenic cell death. In addition, most nanozyme-based immunotherapies are combined with the anti-PD-1 monoclonal antibody (αPD-1) for precise molecular targeting, which transforms the tumour microenvironment from “cold” to “hot” and generates long-term immune-memory effects to further enhance antitumor activity. The tumour microenvironment will be transformed from “cold” to “hot” and a long-term immune memory effect will be generated to further enhance the antitumor effect.
Interferon gamma secreted by CD8+ T cells down-regulates the levels of SLC3A2 and SLC7A11 in tumour cells, thereby decreasing cystine uptake and ultimately leading to the onset of ferroptosis; knockdown of the GPX4 gene in B cells triggers cellular ferroptosis by inducing lipid peroxidation.113 Liu et al. developed a nanoplatform for the co-expression of six enzymes: Lipoxygenase (LOX) and phospholipase A2 (PLA2)-co-loaded FeCo/Fe–Co bimetallic atom nanozymes (FeCo/Fe–Co DAzyme/PL), which not only induced initial immunogenic tumour desferrioxidation through their own multi-enzyme mimetic activity, but also up-regulated arachidonic acid (AA) expression, which, together with CD8+ T cell-derived IFN-γ, synergistically induces ACSL4-mediated immunogenic tumour desferrioxidation. In this process, FeCo/Fe–Co DAzyme/PL could induce lipid peroxidation by efficiently generating ROS and depleting GSH and GPX4 at the tumour site. This study promotes irreversible cascading immunogenic desferrioxidation through multiple ROS storms, GSH/GPX4 depletion, LOX catalysis, and IFN-γ-mediated ACSL4 activation to achieve a synergistic effect of immunogenic death and ferroptosis, which further enhances the antitumor effect of immunotherapy.114
The immune response is commonly used as an adjunct in tumour treatment. For instance, photothermal therapy (PTT) frequently elicits an immune response. PTT induces immunogenic cell death in tumour cells and enhances the body's immune response by improving antigen presentation of APC cells.115 Carbon nanozymes can activate the tumour immune microenvironment by recruiting a large number of tumour-infiltrating immune cells, including T cells, NK cells, and macrophages, thereby converting “cold” tumours into “hot” tumours and activating systemic antitumor immune responses. Yao et al. discovered that carbon quantum dot nanozymes (ChA CQDs) derived from coffee exhibited notable GSH scavenging activity and followed Michaelis–Menten kinetics. These nanozymes exhibited the ability to convert GSH to GSSG, thereby inducing ferroptosis, and effectively suppressed tumour growth in mice implanted with HepG2 cells. Other nanozymes, such as Fe3O4, can enhance antigen presentation by dendritic cells;116 Fe2O3 can modulate the polarization of tumour-associated macrophages;117 and manganese ion-based nanozymes activate the cGAS-STING pathway to increase tumour-infiltrating CD8+ T cells.87 Shen et al. designed a nano-metal–organic framework (referred to as mFe (SS)/DG) that incorporated glucose oxidase (GOx) and adriamycin. Their study demonstrated that both adriamycin and the MOF could induce immunogenic cell death. Fe3+ and organic ligands containing disulfide bonds within this structure down-regulate GPX4, leading to ferroptosis. Ferroptosis, in turn, amplifies the immune response by releasing “find me” signals such as lipid mediators and high mobility group box 1 (HMGB1), resembling the mechanism observed in vaccination.118
It can be seen that the cell death mechanism induced by immunogenic death can synergistically enhance the efficacy of antibody-based tumour treatments when combined with ferroptosis (Fig. 9). Currently, ICD is induced not only by intracellular pathogens and chemotherapeutic agents, but also by a variety of physical therapies, including PTT, photodynamic therapy (PDT), extracorporeal photochemotherapy (ECP), and various forms of ionizing radiation, which further suggests that the mainstream platform for the next-generation antitumor therapy will be the synergistic interaction between a variety of therapies in order to effectively increase the efficacy of antitumor therapy.119,120 Currently, the study of nanozymes is still in its infancy, and their unique roles such as immunogenicity and enzyme-like activity need to be further investigated.
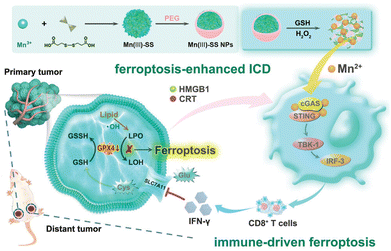 |
| Fig. 9 Illustration of the synthesis process and therapeutic mechanism of TME-responsive Mn(III)-SS NEs, aiming to enhance ferroptosis-induced ICD and immune-driven ferroptosis.121 Reproduced with permission from John Wiley and Sons, copyright 2023. | |
5.3 Photodynamic therapy
Photodynamic therapy (PDT) is an emerging antitumor therapy that incorporates photosensitizers, oxygen molecules, and excitation light to generate ROS to kill cancer cells.122 However, there are still some problems that need to be solved. For example, the hypoxic microenvironment in solid tumours can reduce the therapeutic effect of photodynamic therapy; some cancer cells tend to be resistant to apoptosis and necrotic cell death; not all photosensitizers have the capacity to trigger immunogenic cell death pathways.123 Therefore, a new therapy is needed to improve the efficiency of PDT. Fortunately, PDT can not only induce ferroptosis alone, but also in combination with ferroptosis inducers and metal ions.124 The nanozymes can produce ROS under light irradiation, thus co-regulating the intracellular redox homeostasis leading to cell death, and combining them with PDT can enhance the anticancer effect.
In the realm of nanozymes, MOF-based nanozymes stand out due to their structural similarity to natural enzymes, such as binding and stabilization of the transition state, involvement of functional groups for proton transfer, charge stabilization, metal coordination, and supplying nucleophiles or electrophiles. For example, Ye et al. integrated Pt NPs and the NIR photosensitizer CyI into iron-based MOFs. The MOFs containing photosensitizers can convert oxygen from tumour tissues into monoclinic oxygen.125 The MOFs not only act as delivery carriers for photosensitizers to improve water solubility, but also act as inducers of ferroptosis, which converts O2 from tumour tissues into monoclinic O2.
To provide a location for the multi-enzyme cascade reaction and at the same time avoid the rapid removal of nanoparticles from the blood stream via the mononuclear phagocyte system, pre-coated protein crowns on the surface of nanoparticles were suggested as they can effectively shield plasma proteins from uptake. By using mesoporous silica nanoparticles (MSNs) with high specific surface area and large pore size as substrates, ultrasmall AuNPs with GOx-like activity were grown in situ based on a reduction reaction, and then the photosensitizers cerium chloride (Ce6) and FTn-Ru (Ru nanozymes with cat-like activity) were co-loaded on the surface of the MSN-Au via a simple mixing method by amidation reactions or within the pores.126 The results showed that human ferritin heavy chain nanocage (HTn)-based protein crown-forming and cascade nanozymes consumed glucose and H2O2 in the TME, sustained O2 production, and enhanced starvation therapy and PDT-targeted tumour treatment protocols. This study achieved an enzymatic cascade reaction of two nanozymes on a single nanoplatform, demonstrating that genetically engineered human ferritin heavy chain nanocages can actively bind tumour cells. By constructing Cu-tetrakis(4-carboxyphenyl)porphyrin-based chloroporphyrin (Fe(III)) (Cu-TCPP(Fe)) MOF nanosystems for the efficient doping of AuNPs and RSL3, Kobayashi and Choyke et al. found that it was possible to use these nanocatalytic activities to inhibit both the GPX4/GSH and FSP1/CoQ10H2 pathways.88 In conjunction with the GPX3 inactivation function of RSL4, the nanosystems have the ability to universally inhibit three anti-ferroptosis pathways in tumour cells.
It has been found that photosensitizers such as the dihydroporphyrin (Ce6), which is one of the three elements of photodynamic therapy, can itself potentiate the ferroptosis-activating effect of hemin through both classical and non-classical modes.127 The photosensitizer Aloe emodin induces cellular ferroptosis by specifically inhibiting GSH S-transferase P1 (GSTP1) production.128 By binding to various cell membranes, such as erythrocyte membranes and macrophage membranes, it is possible to specifically target tumours, evade immune clearance or activate T-cells, while achieving the effect of ferroptosis activation/photodynamic/immunotherapy interactions.60
In addition to multiple synergistic therapeutic effects, iron-based compounds often used as nanozymes can be used as magnetic resonance imaging (MRI) contrast agents. Wang et al. doped gadolinium (Gd) into Prussian blue nanozymes (PBzyme), thereby modulating the density of states of Fe in PBzyme, which lowered the Gibbs free energy of the catalytic cycle of catalysis of CAT, resulting in the enhancement of its CAT-like activity (4-fold higher than that of PBzyme). Other metal ions such as Mn2+ and Cu2+ also have excellent performance in enhanced T1-weighted MRI and can improve the relaxation efficiency to realize the integration of nanozymes into diagnostic and therapeutic treatments, which will be a novel platform for the next-generation tumour therapeutics.
5.4 Photothermal therapy
Photothermal therapy (PTT) is an emerging non-invasive and highly targeted modality for cancer treatment. It harnesses the potential of photothermal agents (PTAs) characterized by their high photothermal conversion efficiency.129,130 However, PTT often involves reaching temperatures of 50 °C or higher, resulting in unintended damage to both tumour and normal tissues.131 This excessive heat generation triggers the body's defense mechanism, leading to the production of heat shock proteins (HSPs) that can decrease the sensitivity of tumour cells. The limited solubility, serum instability, and cytotoxicity associated with a traditional HSP inhibitor, including garcinia cambogia, 17-AAG, tretinoin, and siRNA, have hindered their effective utilization in PTT.132 How to inhibit HSPs at relatively mild temperatures to improve the efficiency of PTT is an important issue to be addressed in the future.
Metal nanoparticles can absorb visible light by localized surface plasmon resonance (LSPR) and be used for photocatalytic reactions.133,134 Pd nanozyme has strong light absorption properties and exhibits excellent photothermal conversion performance in the near infrared region (NIR-II) (1000–1400 nm).135 In 2021, Chang et al. prepared single-atom Pd nanozymes based on Pd SAzyme with peroxidase (POD) and glutathione oxidase-like activities and excellent photothermal conversion properties for the enhancement of ferroptosis-based cryogenic PTT antitumor therapies.136 Unlike the conventional iron-dependent ferroptosis approach, in this study the single-atom Pd was dispersed in the metal centres of nanozymes to maximize the atomic utilization of the active centres and the density of the features.137 Thus, the GPX4 pathway is directly modulated to directly resist LPO in biofilms to trigger the ferroptosis-like cell death process. LPO can spontaneously form aldehyde degradation products that cross-link the primary amines of proteins, thereby disrupting the protein structure and function. The study showed a significant reduction in the GPX4 expression, loss of mitochondrial membrane potential and damage under 1064 nm laser irradiation. Compared with the control group, HSP70 was significantly down-regulated in the Pd SAzyme-treated tumour group, and tumour growth was significantly inhibited. The maximum temperature in this study was only 42.68 °C, which shows that Pd SAzyme can promote low temperature PTT with a significant therapeutic effect by depleting HSPs through a large amount of LPO and ROS produced in the process of ferroptosis at a low temperature. In addition, under NIR irradiation, the activity of nanozymes can be increased by 3.5-fold to effectively digest collagen in the tumour extracellular matrix (ECM), thus enhancing the accumulation of nanoparticles in the tumour, and thus improving PTT (Fig. 10).141,142
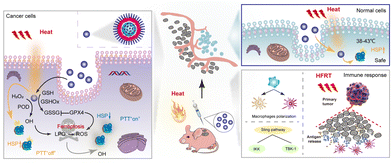 |
| Fig. 10 Nanozymes synergizing with ferroptosis in photothermal therapy, inhibiting HSPs while also triggering an immune response, including macrophage polarization,138 antigen presentation,139 and activation of the STING pathway.140 | |
Copper single-atom nanozymes (Cu SAzyme) have been employed for the inhibition of heat shock proteins (HSPs).143 In addition to its intrinsic enzyme-like activity, the incorporation of LIK066 (a sodium-glucose co-transporter protein inhibitor) into the nanozyme system can disrupt existing HSPs through multiple mechanisms. It inhibits the energy required for HSP synthesis and induces disruption of ROS-active centres, leading to the inhibition of HSP function. The study was conducted through a two-pronged strategy of sodium-dependent glucose-transporting protein (SGLT) inhibitors and ROS centres, thereby inducing photothermal therapy.
Nanozyme-based photothermal therapy demonstrates the potential for enhanced antitumor efficacy when synergistically integrated with a diverse array of complementary anticancer modalities (Fig. 11).144 To achieve more precise tumour targeting, a nanozyme Fe3O4@Cu1.77Se has been designed to release ultra-abundant, ultra-small Fe3O4 and Cu1.77Se nanoparticles in the presence of matrix metalloproteinases, which are overexpressed in many types of cancer.145 The released nanoparticles facilitate the elevation of hydroxyl radicals through the Fenton reaction and depletion of GSH. Due to the unique magnetic properties and high biocompatibility of Fe3O4 nanoparticles, Cu1.77Se has a better photothermal conversion efficiency in the NIR region II. The study demonstrated a significant enhancement of the NMR T2 signal and increased efficiency. This synergistic effect enabled precisely targeted antitumor therapy through NIR photothermal ferroptosis, guided by tumour-enhanced MRI.146
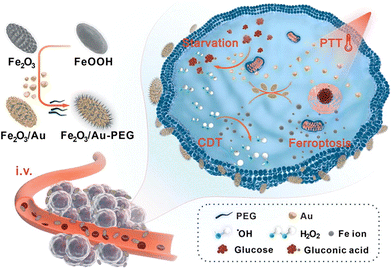 |
| Fig. 11 Using a derivative strategy for fabrication of Fe2O3@Au-PEG hybrid nanozyme that holds peroxidase and glucose oxidase mimicking properties, which realized highly efficient treatment of triple negative breast cancer through starvation/PTT/CDT/ferroptosis concerted effects.144 Reproduced with permission from Elsevier, copyright 2023. | |
5.5 Radiotherapy
The hypoxic environment in tumour tissues plays a crucial role in the development of treatment tolerance and reduced efficacy of radiation therapy. There is a strong association between radiotherapy and ferroptosis.147 Radiotherapy induces multiple changes associated with ferroptosis, including promotion of lipid peroxidation, induction of the expression of key ferroptosis-related proteins such as acyl coenzyme A synthetase long-chain family member 4 (ACSL4), GPX, and the ferroptosis marker gene PTGS2, as well as the reduction of cystine uptake through inhibition of SLC7A11. Additionally, tumour cells treated with radiotherapy exhibit characteristic morphological features of ferroptosis, such as mitochondrial contraction and increased membrane density.148 Moreover, the use of ferroptosis inhibitors can partially restore cell survival following radiotherapy, demonstrating a stronger recovery compared to other regulatory cell death (RCD) inhibitors.149 Notably, Isozaki et al. uncovered that mutations or deletions in the KEAP1 in lung cancer cells upregulate FSP1 expression through NRF2, resulting in resistance to both ferroptosis and radiotherapy. This finding led to the development of the therapeutic strategy for KEAP1-inactivated lung cancer, involving the targeting of the CoQ-FSP1 axis-mediated ferroptosis defense to sensitize the cancer cells to radiotherapy.150 More and more studies are focusing on the promotion of the Fenton effect and enhancement of catalytic activity through the action of external fields, such as temperature gradients, microwaves, and X-rays.151 X-rays can be better used to treat deep-seated tumours as opposed to NIR light. Similar to the traditional photothermal reaction in which UV irradiation reduces Fe3+ ions to Fe2+, high-energy radiation can also promote Fenton reactions.
Nanozyme activity can be further enhanced by the introduction of X-rays, which promote the frequency of FeII/FeIII conversion and sustain the catalytic activity. For example, it has been suggested that the coordination of Fe with N on carbon nanosheets allows for the incorporation of Fe atoms into the carbon nanosheet structure, with N atoms serving as coordinating ligands.152 This coordination creates high atomic utilization efficiency and catalytic activity. The obtained FeN4-SAzyme can initiate a self-cascading enzyme reaction at the site, converting intracellular H2O2 into ˙OH radicals and consuming glutathione (GSH), thereby promoting ferroptosis. Moreover, the loading of glucose oxidase (GOD) enables the generation of additional H2O2 through downstream reactions, further enhancing the catalytic activity of FeN4-SAzyme under X-ray radiation. The treatment with GOD@FeN4-SAzym, in combination with X-rays, resulted in a high total apoptosis rate of 90.40% in 4T1 cells compared to the control group. Additionally, the treatment induced the collapse of mitochondrial membrane potential and significantly reduced the GPX4 expression, confirming the induction of apoptosis and ferroptosis. These findings demonstrated the therapeutic potential of nanozymes in triggering in situ apoptosis and ferroptosis, with the combination of X-rays enhancing the efficacy of the radiation cascade catalytic therapy.
Radiotherapy and nanozymes can enhance tumour treatment by mutually reinforcing effects through the generation of excess ROS, and the ferroptosis pathway.153 In addition to the classical iron-based nanozymes, we envision that this outfield-enhanced SAzyme catalytic model can be extended to a variety of outfields as well as other nanozymes to enhance enzyme-like activity from the inside out.154 For example, cobalt molybdate-phosphomolybdate nanosheets (CPMNSs) have been constructed to induce ferroptosis by generating highly toxic ˙OH through the Fenton-like effect of Co2+.86 In addition, ferroptosis can be triggered by catalyzing H2O2 generation and oxidizing GSH through Pd–C SAzyme as these processes can downregulate the expression of GPX4.155 Furthermore, the noble metal Pd further enhanced the photoelectric effect of FLASH-RT, making it a promising radiosensitizer (Fig. 12).
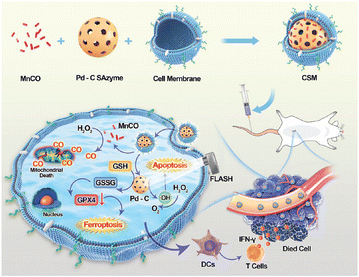 |
| Fig. 12 Multi-pathway enhancement of ferroptosis for radio-immunotherapy by encapsulating a porous Pd-C SAzyme loaded with a carbon monoxide donor within the membrane of 4T1 cancer cells. It exhibited heightened cellular apoptosis, reduced mitochondrial membrane potential, increased ROS production, elevated levels of LPO, and augmented malondialdehyde content, thereby inducing ferroptosis to enhance the anticancer efficacy of radiotherapy.155 Reproduced with permission from John Wiley and Sons, copyright 2023. | |
High-numbered atoms like these are characterized by strong X-ray absorption,156 which can interact with high-energy ionizing radiation to produce radioactive particles such as photons, Compton electrons, positron-negative electron pairs, and oscillating electrons can be used to directly or indirectly cause intracellular DNA damage and achieve external physical radiosensitization.157,158 Ferroptosis is still a relatively new field, and synergistic therapy using nanoparticles with high atomic numbers has great potential for the next generation of antitumor therapy.
Discussion and presepctive
Nanozymes have sparked a promising frontier in cancer therapy by inducing efficient ferroptosis, which could lead to efficient antitumor therapy. In detail, nanosized materials exhibit catalytic properties similar to natural enzymes both in vitro and in vivo. Then, intracellular redox homeostasis is disrupted and cell membrane lipid peroxidation (LPO) is activated, representing the activation of typical ferroptosis. Besides, ferroptosis could synergize with other strategies, such as immunotherapy, photothermal therapy, photodynamic therapy, and radiotherapy, demonstrating highly effective tumour therapeutic effects, even for resistant cancer treatment. Nanozymology and ferroptosis have achieved significant advancements in synergizing with immunotherapy, photothermal therapy, photodynamic therapy, radiotherapy, etc., resulting in improved anticancer effects.
Advances in understanding ferroptosis, including its cellular pathways and metabolic changes, will illuminate new avenues for therapy development. Additionally, monitoring biomarkers for ferroptosis remains challenging, and a more profound understanding of nanozyme mechanisms is required. As the applications of nanozymes continue to expand, bridging the gap between laboratory research and industrial demand is a crucial step. This will involve harnessing the multiple enzyme-like activities exhibited by nanomaterials to maximize their value and resource utilization. Deep neural networks have emerged as powerful tools for accurately predicting the high POD-like and OXD-like activities. By addressing these challenges and conducting comprehensive research, the future looks bright for nanozyme-based ferroptosis therapy, leading to optional options for innovative and effective cancer treatments.
The field of combining nanozymes and ferroptosis holds promising prospects for advancing cancer therapy. By harnessing the catalytic activity of nanozymes and the unique characteristics of ferroptosis, synergistic effects can be achieved to enhance the efficacy of cancer treatment. Future research endeavors may focus on developing novel nanozyme-based platforms that can precisely regulate ferroptotic pathways, optimize drug delivery, and overcome potential challenges such as biocompatibility and stability. Additionally, exploring the synergistic interactions between nanozymes and other therapeutic modalities could further expand the therapeutic potential of this innovative approach. Overall, the integration of nanozymes and ferroptosis represents a promising avenue for the development of more effective and targeted cancer treatments in the future.
Conflicts of interest
The authors declare no competing financial interest.
Acknowledgements
This work was supported by the National Key R&D Program of China (2022YFC2402900), the National Natural Science Foundation of China (22335005, 21925505 and 22305177), the Innovation Program of Shanghai Municipal Education Commission (2023ZKZD28), the International Scientific Collaboration Fund of Science and Technology Commission of Shanghai Municipality (21520710100), the fellowship of China Postdoctoral Science Foundation (2022M720107) and Shanghai “Super Postdoc” Incentive Plan (2022568), the Shanghai Rising-Star Program (Sailing, 23YF1433000), Postdoctoral Fellowship Program of China Postdoctoral Science Foundation (GZB20230517), the Science and Technology Project of Guangzhou City (201802020018), and the Guangdong Science and Technology Program (2019A1515010408). J. D. is the recipient of a 5-year National Science Fund for Distinguished Young Scholars.
Notes and references
- H. Sung, J. Ferlay, R. L. Siegel, M. Laversanne, I. Soerjomataram, A. Jemal and F. Bray, Global cancer statistics 2020: GLOBOCAN estimates of incidence and mortality worldwide for 36 cancers in 185 countries, Ca-Cancer J. Clin., 2021, 71, 209–249 CrossRef PubMed
.
- R. L. Siegel, K. D. Miller, N. S. Wagle and A. Jemal, Cancer statistics, Ca-Cancer J. Clin., 2023, 73, 17–48 CrossRef PubMed
.
- E. Pleasance, A. Bohm, L. M. Williamson, J. M. T. Nelson, Y. Shen, M. Bonakdar, E. Titmuss, V. Csizmok, K. Wee, S. Hosseinzadeh, C. J. Grisdale, C. Reisle, G. A. Taylor, E. Lewis, M. R. Jones, D. Bleile, S. Sadeghi, W. Zhang, A. Davies, B. Pellegrini, T. Wong, R. Bowlby, S. K. Chan, K. L. Mungall, E. Chuah, A. J. Mungall, R. A. Moore, Y. Zhao, B. Deol, A. Fisic, A. Fok, D. A. Regier, D. Weymann, D. F. Schaeffer, S. Young, S. Yip, K. Schrader, N. Levasseur, S. K. Taylor, X. Feng, A. Tinker, K. J. Savage, S. Chia, K. Gelmon, S. Sun, H. Lim, D. J. Renouf, S. J. M. Jones, M. A. Marra and J. Laskin, Whole-genome and transcriptome analysis enhances precision cancer treatment options, Ann. Oncol., 2022, 33, 939–949 CrossRef CAS PubMed
.
- A. N. von Krusenstiern, R. N. Robson, N. Qian, B. Qiu, F. Hu, E. Reznik, N. Smith, F. Zandkarimi, V. M. Estes, M. Dupont, T. Hirschhorn, M. S. Shchepinov, W. Min, K. A. Woerpel and B. R. Stockwell, Identification of essential sites of lipid peroxidation in ferroptosis, Nat. Chem. Biol., 2023, 19, 719–730 CrossRef CAS PubMed
.
- T. M. Seibt, B. Proneth and M. Conrad, Role of GPX4 in ferroptosis and its pharmacological implication, Free Radicals Biol. Med., 2019, 133, 144–152 CrossRef CAS PubMed
.
- W. S. Yang, R. SriRamaratnam, M. E. Welsch, K. Shimada, R. Skouta, V. S. Viswanathan, J. H. Cheah, P. A. Clemons, A. F. Shamji, C. B. Clish, L. M. Brown, A. W. Girotti, V. W. Cornish, S. L. Schreiber and B. R. Stockwell, Regulation of ferroptotic cancer cell death by GPX4, Cell, 2014, 156, 317–331 CrossRef CAS PubMed
.
- B. Hassannia and P. Vandenabeele, and T. Vanden Berghe, Targeting ferroptosis to iron out cancer, Cancer Cell, 2019, 35, 830–849 CrossRef CAS PubMed
.
- Y. Wang, T. Sun and C. Jiang, Nanodrug delivery systems for ferroptosis-based cancer therapy, J. Controlled Release, 2022, 344, 289–301 CrossRef CAS PubMed
.
- J. Kraut, How do enzymes work?, Science, 1988, 242, 533–540 CrossRef CAS PubMed
.
- G. Lei, L. Zhuang and B. Gan, Targeting ferroptosis as a vulnerability in cancer, Nat. Rev. Cancer, 2022, 22, 381–396 CrossRef CAS PubMed
.
- Y. Su, B. Zhao, L. Zhou, Z. Zhang, Y. Shen, H. Lv, L. H. H. AlQudsy and P. Shang, Ferroptosis, a novel pharmacological mechanism of anti-cancer drugs, Cancer Lett., 2020, 483, 127–136 CrossRef CAS PubMed
.
- M. Morales and X. Xue, Targeting iron metabolism in cancer therapy, Theranostics, 2021, 11, 8412–8429 CrossRef CAS PubMed
.
- Z. Tang, P. Zhao, H. Wang, Y. Liu and W. Bu, Biomedicine meets Fenton chemistry, Chem. Rev., 2021, 121, 1981–2019 CrossRef CAS PubMed
.
- M. P. Dias, S. C. Moser, S. Ganesan and J. Jonkers, Understanding and overcoming resistance to PARP inhibitors in cancer therapy, Nat. Rev. Clin. Oncol., 2021, 18, 773–791 CrossRef PubMed
.
- C. Allen, S. Her and D. A. Jaffray, Radiotherapy for cancer: present and future, Adv. Drug Delivery Rev., 2017, 109, 1–2 CrossRef CAS PubMed
.
- B. Hu, X. Zhao, E. Wang, J. Zhou, J. Li, J. Chen and G. Du, Efficient heterologous expression of cytochrome P450 enzymes in microorganisms for the biosynthesis of natural products, Crit. Rev. Biotechnol., 2022, 43, 227–241 CrossRef PubMed
.
- L. Gao, J. Zhuang, L. Nie, J. Zhang, Y. Zhang, N. Gu, T. Wang, J. Feng, D. Yang, S. Perrett and X. Yan, Intrinsic peroxidase-like activity of ferromagnetic nanoparticles, Nat. Nanotechnol., 2007, 2, 577–583 CrossRef CAS PubMed
.
- Y. Wei, J. Wu, Y. Wu, H. Liu, F. Meng, Q. Liu, A. C. Midgley, X. Zhang, T. Qi, H. Kang, R. Chen, D. Kong, J. Zhuang, X. Yan and X. Huang, Prediction and design of nanozymes using explainable machine learning, Adv. Mater., 2022, 34, 2201736 CrossRef CAS PubMed
.
- D. Jiang, D. Ni, Z. T. Rosenkrans, P. Huang, X. Yan and W. Cai, Nanozyme: new horizons for responsive biomedical applications, Chem. Soc. Rev., 2019, 48, 3683–3704 RSC
.
- A. Robert and B. Meunier, How to define a nanozyme, ACS Nano, 2022, 16, 6956–6959 CrossRef CAS PubMed
.
- X. Zhou, M. You, F. Wang, Z. Wang, X. Gao, C. Jing, J. Liu, M. Guo, J. Li, A. Luo, H. Liu, Z. Liu and C. Chen, Multifunctional graphdiyne-Cerium oxide nanozymes facilitate MicroRNA delivery and attenuate tumor hypoxia for highly efficient radiotherapy of esophageal cancer, Adv. Mater., 2021, 33, 2100556 CrossRef CAS PubMed
.
- Q. Liu, J. Tian, J. Liu, M. Zhu, Z. Gao, X. Hu, A. C. Midgley, J. Wu, X. Wang, D. Kong, J. Zhuang, J. Liu, X. Yan and X. Huang, Modular assembly of tumor-penetrating and oligomeric nanozyme based on intrinsically self-assembling protein nanocages, Adv. Mater., 2021, 33, 2103128 CrossRef CAS PubMed
.
- L. Zeng, Y. Han, Z. Chen, K. Jiang, D. Golberg and Q. Weng, Biodegradable and peroxidase-mimetic boron oxynitride nanozyme for breast cancer therapy, Adv. Sci., 2021, 8, 2101184 CrossRef CAS PubMed
.
- X. Ren, D. Chen, Y. Wang, H. Li, Y. Zhang, H. Chen, X. Li and M. Huo, Nanozymes-recent development and biomedical applications, J. Nanobiotechnol., 2022, 20, 92 CrossRef CAS PubMed
.
- S. Guo, X. Yang, S. Guan, J. Lu and S. Zhou, Bioinspired construction of an enzyme-mimetic supramolecular nanoagent for RNS-augmented cascade chemodynamic therapy, ACS Appl. Mater. Interfaces, 2022, 14, 46252–46261 CrossRef CAS PubMed
.
- W. H. Koppenol, P. L. Bounds and C. V. Dang, Otto Warburg's contributions to current concepts of cancer metabolism, Nat. Rev. Cancer, 2011, 11, 325–337 CrossRef CAS PubMed
.
- Y. Cheng, R. Yuan, Y. Chai, H. Niu, Y. Cao, H. Liu, L. Bai and Y. Yuan, Highly sensitive luminol electrochemiluminescence immunosensor based on ZnO nanoparticles and glucose oxidase decorated graphene for cancer biomarker detection, Anal. Chim. Acta, 2012, 745, 137–142 CrossRef CAS PubMed
.
- H. Dong, W. Du, J. Dong, R. Che, F. Kong, W. Cheng, M. Ma, N. Gu and Y. Zhang, Depletable peroxidase-like activity of Fe3O4 nanozymes accompanied with separate migration of electrons and iron ions, Nat. Commun., 2022, 13, 5365 CrossRef CAS PubMed
.
- X. Lin, E. Shuang and X. Chen, Metal-organic framework/3,3’,5,5’-tetramethylbenzidine based multidimensional spectral array platform for sensitive discrimination of protein phosphorylation, J. Colloid Interface Sci., 2021, 602, 513–519 CrossRef CAS PubMed
.
- M. Liang and X. Yan, Nanozymes: from new concepts, mechanisms, and standards to applications, Acc. Chem. Res., 2019, 52, 2190–2200 CrossRef CAS PubMed
.
- N. Wang, L. Zhu, D. Wang, M. Wang, Z. Lin and H. Tang, Sono-assisted preparation of highly-efficient peroxidase-like Fe3O4 magnetic nanoparticles for catalytic removal of organic pollutants with H2O2, Ultrason. Sonochem., 2010, 17, 526–533 CrossRef CAS PubMed
.
- S. Dong, Y. Dong, B. Liu, J. Liu, S. Liu, Z. Zhao, W. Li, B. Tian, R. Zhao, F. He, S. Gai, Y. Xie, P. Yang and Y. Zhao, Guiding transition metal-doped hollow cerium tandem nanozymes with elaborately regulated multi-enzymatic activities for intensive chemodynamic therapy, Adv. Mater., 2022, 34, 2107054 CrossRef CAS PubMed
.
- T. Wang, Y. Li, E. J. Cornel, C. Li and J. Du, Combined antioxidant-antibiotic treatment for effectively healing Infected diabetic wounds based on polymer vesicles, ACS Nano, 2021, 15, 9027–9038 CrossRef CAS PubMed
.
- W. H. Koppenol and R. H. Hider, Iron and redox cycling. Do's and dont's, Free Radicals Biol. Med., 2019, 133, 3–10 CrossRef CAS PubMed
.
- D. Xu, L. Wu, H. Yao and L. Zhao, Catalase-Like nanozymes: classification, catalytic mechanisms, and their applications, Small, 2022, 18, 2203400 CrossRef CAS PubMed
.
- N. Feng, Q. Li, Q. Bai, S. Xu, J. Shi, B. Liu and J. Guo, Development of an Au-anchored Fe single-atom nanozyme for biocatalysis and enhanced tumor photothermal therapy, J. Colloid Interface Sci., 2022, 618, 68–77 CrossRef CAS PubMed
.
- G. Yang, J. Ji and Z. Liu, Multifunctional MnO2 nanoparticles for tumor microenvironment modulation and cancer therapy, Wiley Interdiscip. Rev.: Nanomed. Nanobiotechnol., 2021, 13, 1720 Search PubMed
.
- P. Gao, X. Shen, X. Liu, B. Cui, M. Wang, X. Wan, N. Li and B. Tang, Covalent organic framework-derived carbonous nanoprobes for cancer cell imaging, ACS Appl. Mater. Interfaces, 2021, 13, 41498–41506 CrossRef CAS PubMed
.
- W. J. Ong, L. L. Tan, Y. H. Ng, S. T. Yong and S. P. Chai, Graphitic carbon nitride (g-C3N4)-based photocatalysts for artificial photosynthesis and environmental remediation: are we a step closer to achieving sustainability?, Chem. Rev., 2016, 116, 7159–7329 CrossRef CAS PubMed
.
- S. Min, Q. Yu, J. Ye, P. Hao, J. Ning, Z. Hu and Y. Chong, Nanomaterials with glucose oxidase-mimicking activity for biomedical applications, Molecules, 2023, 28, 4615 CrossRef CAS PubMed
.
- L. Gao, K. Fan and X. Yan, Iron oxide nanozyme: a multifunctional enzyme mimetic for biomedical applications, Theranostics, 2017, 7, 3207–3227 CrossRef CAS PubMed
.
- M. Sharifi, S. H. Hosseinali, P. Yousefvand, A. Salihi, M. S. Shekha, F. M. Aziz, A. JouyaTalaei, A. Hasan and M. Falahati, Gold nanozyme: biosensing and therapeutic activities, Mater. Sci. Eng., C, 2020, 108, 110422 CrossRef CAS PubMed
.
- N. Feng, Y. Liu, X. Dai, Y. Wang, Q. Guo and Q. Li, Advanced applications of cerium oxide based nanozymes in cancer, RSC Adv., 2022, 12, 1486–1493 RSC
.
- P. Xu, W. Huang, J. Yang, X. Fu, W. Jing, Y. Zhou, Y. Cai and Z. Yang, Copper-rich multifunctional Prussian blue nanozymes for infected wound healing, Int. J. Biol. Macromol., 2023, 227, 1258–1270 CrossRef CAS PubMed
.
- M. Tang, Z. Zhang, T. Sun, B. Li and Z. Wu, Manganese-based nanozymes: Preparation, catalytic mechanisms, and biomedical applications, Adv. Healthcare Mater., 2022, 11, e2201733 CrossRef PubMed
.
- H. Sun, Y. Zhou, J. Ren and X. Qu, Carbon nanozymes: Enzymatic properties, catalytic mechanism, and applications, Angew. Chem., Int. Ed., 2018, 57, 9224–9237 CrossRef CAS PubMed
.
- Y. Huang, J. Ren and X. Qu, Nanozymes: Classification, catalytic mechanisms, activity regulation, and applications, Chem. Rev., 2019, 119, 4357–4412 CrossRef CAS PubMed
.
- Z. Tang, Y. Liu, M. He and W. Bu, Chemodynamic therapy: tumour microenvironment-mediated Fenton and Fenton-like reactions, Angew. Chem., Int. Ed., 2019, 58, 946–956 CrossRef CAS PubMed
.
- X. Zhu, J. Wu, R. Liu, H. Xiang, W. Zhang, Q. Chang, S. Wang, R. Jiang, F. Zhao, Q. Li, L. Huang, L. Yan and Y. Zhao, Engineering single-atom iron nanozymes with radiation-enhanced self-cascade catalysis and self-supplied H2O2 for radio-enzymatic therapy, ACS Nano, 2022, 16, 18849–18862 CrossRef CAS PubMed
.
- A. Lin, Q. Liu, Y. Zhang, Q. Wang, S. Li, B. Zhu, L. Miao, Y. Du, S. Zhao and H. Wei, A dopamine-enabled universal assay for catalase and catalase-like nanozymes, Anal. Chem., 2022, 94, 10636–10642 CrossRef CAS PubMed
.
- M. Perwez, S. Y. Lau, D. Hussain, S. Anboo, M. Arshad and P. Thakur, Nanozymes and nanoflower: physiochemical properties, mechanism and biomedical applications, Colloids Surf., B, 2023, 225, 113241 CrossRef CAS PubMed
.
- G. Tang, J. He, J. Liu, X. Yan and K. Fan, Nanozyme for tumor therapy: Surface modification matters, Exploration, 2021, 1, 75–89 CrossRef PubMed
.
- P. Ngoc Man, N. Thanh Loc and J. Kim, Nanozyme-based enhanced cancer immunotherapy, Tissue Eng. Regener. Med., 2022, 19, 237–252 CrossRef PubMed
.
- N. German, A. Ramanaviciene and A. Ramanavicius, Formation and electrochemical evaluation of polyaniline and polypyrrole nanocomposites based on glucose oxidase and gold nanostructures, Polymers, 2020, 12, 3026 CrossRef CAS PubMed
.
- J. Wang, Y. Chu, Z. Zhao, C. Zhang, Q. Chen, H. Ran, Y. Cao and C. Wu, Piezoelectric enhanced sulfur doped graphdiyne nanozymes for synergistic ferroptosis-apoptosis anticancer therapy, J. Nanobiotechnol., 2023, 21, 311 CrossRef CAS PubMed
.
- X. Sun, S. Guo, C.-S. Chung, W. Zhu and S. Sun, A sensitive H2O2 assay based on dumbbell-like PtPd-Fe3O4 nanoparticles, Adv. Mater., 2013, 25, 132–136 CrossRef CAS PubMed
.
- D. Tang, X. Chen, R. Kang and G. Kroemer, Ferroptosis: molecular mechanisms and health implications, Cell Res., 2021, 31, 107–125 CrossRef CAS PubMed
.
- F. Ursini and M. Maiorino, Lipid peroxidation and ferroptosis: The role of GSH and GPx4, Free Radical Biol. Med., 2020, 152, 175–185 CrossRef CAS PubMed
.
- P. Liu, Y. Feng, H. Li, X. Chen, G. Wang, S. Xu, Y. Li and L. Zhao, Ferrostatin-1 alleviates lipopolysaccharide-induced acute lung injury via inhibiting ferroptosis, Cell. Mol. Biol. Lett., 2020, 25, 10 CrossRef CAS PubMed
.
- R. Song, T. Li, J. Ye, F. Sun, B. Hou, M. Saeed, J. Gao, Y. Wang, Q. Zhu, Z. Xu and H. Yu, Acidity-activatable dynamic nanoparticles boosting ferroptotic cell death for immunotherapy of cancer, Adv. Mater., 2021, 33, 2101155 CrossRef CAS PubMed
.
- T. M. Seibt, B. Proneth and M. Conrad, Role of GPX4 in ferroptosis and its pharmacological implication, Free Radicals Biol. Med., 2019, 133, 144–152 CrossRef CAS PubMed
.
- X. Chen, R. Kang, G. Kroemer and D. Tang, Broadening horizons: the role of ferroptosis in cancer, Nat. Rev. Clin. Oncol., 2021, 18, 280–296 CrossRef CAS PubMed
.
- D. Liang, A. M. Minikes and X. Jiang, Ferroptosis at the intersection of lipid metabolism and cellular signaling, Mol. Cell, 2022, 82, 2215–2227 CrossRef CAS PubMed
.
- X. Ma, L. Xiao, L. Liu, L. Ye, P. Su, E. Bi, Q. Wang, M. Yang, J. Qian and Q. Yi, CD36-mediated ferroptosis dampens intratumoral CD8+ T cell effector function and impairs their antitumor ability, Cell Metab., 2021, 33, 1001–1012 CrossRef CAS PubMed
.
- B. R. Stockwell, Ferroptosis turns 10: emerging mechanisms, physiological functions, and therapeutic applications, Cell, 2022, 185, 2401–2421 CrossRef CAS PubMed
.
- B. Tang, R. Yan, J. Zhu, S. Cheng, C. Kong, W. Chen, S. Fang, Y. Wang, Y. Yang, R. Qiu, C. Lu and J. Ji, Integrative analysis of the molecular mechanisms, immunological features and immunotherapy response of ferroptosis regulators across 33 cancer types, Int. J. Biol. Sci., 2022, 18, 180–198 CrossRef CAS PubMed
.
- J. Li, F. Cao, H. L. Yin, Z. J. Huang, Z. T. Lin, N. Mao, B. Sun and G. Wang, Ferroptosis: past, present and future, Cell Death Discovery, 2020, 11, 88 CrossRef PubMed
.
- C. Zhang, X. Liu, S. Jin, Y. Chen and R. Guo, Ferroptosis in cancer therapy: a novel approach to reversing drug resistance, Mol. Cancer, 2022, 21, 47 CrossRef PubMed
.
- A. Mullard, Addressing cancer's grand challenges, Nat. Rev. Drug Discovery, 2020, 19, 825–826 CrossRef CAS PubMed
.
- P. An, Z. Gao, K. Sun, D. Gu, H. Wu, C. You, Y. Li, K. Cheng, Y. Zhang, Z. Wang and B. Sun, Photothermal-enhanced inactivation of glutathione peroxidase for ferroptosis sensitized by an autophagy promotor, ACS Appl. Mater. Interfaces, 2019, 11, 42988–42997 CrossRef CAS PubMed
.
- D. Meyerstein, Re-examining Fenton and Fenton-like reactions, Nat. Rev. Chem., 2021, 5, 595–597 CrossRef CAS PubMed
.
- Z. Tang, Y. Liu, M. He and W. Bu, Chemodynamic therapy: tumour microenvironment-mediated Fenton and Fenton-like reactions, Angew. Chem., Int. Ed., 2019, 58, 946–956 CrossRef CAS PubMed
.
- X. Qian, J. Zhang, Z. Gu and Y. Chen, Nanocatalysts-augmented Fenton chemical reaction for nanocatalytic tumor therapy, Biomaterials, 2019, 211, 1–13 CrossRef CAS PubMed
.
- D. M. Stanbury, The principle of detailed balancing, the iron-catalyzed disproportionation of hydrogen peroxide, and the Fenton reaction, Dalton Trans., 2022, 51, 2135–2157 RSC
.
- Z. Shen, J. Song, B. C. Yung, Z. Zhou, A. Wu and X. Chen, Emerging strategies of cancer therapy based on ferroptosis, Adv. Mater., 2018, 30, 1704007 CrossRef PubMed
.
- T. Chen, Q. Chu, M. Li, G. Han and X. Li, Fe3O4@Pt nanoparticles to enable combinational electrodynamic/chemodynamic therapy, J. Nanobiotechnol., 2021, 19, 206 CrossRef CAS PubMed
.
- Z. Zhou, K. Ni, H. Deng and X. Chen, Dancing with reactive oxygen species generation and elimination in nanotheranostics for disease treatment, Adv. Drug Delivery Rev., 2020, 158, 73–90 CrossRef CAS PubMed
.
- Y. Jiang, P. K. Upputuri, C. Xie, Z. Zeng, A. Sharma, X. Zhen, J. Li, J. Huang, M. Pramanik and K. Pu, Metabolizable semiconducting polymer nanoparticles for second near-infrared photoacoustic imaging, Adv. Mater., 2019, 31, 1808166 CrossRef PubMed
.
- L. Zeng, Y. Han, Z. Chen, K. Jiang, D. Golberg and Q. Weng, Biodegradable and peroxidase-mimetic boron oxynitride nanozyme for breast cancer therapy, Adv. Sci., 2021, 8, 2101184 CrossRef CAS PubMed
.
- J. Wang, W. Liu, G. Luo, Z. Li, C. Zhao, H. Zhang, M. Zhu, Q. Xu, X. Wang, C. Zhao, Y. Qu, Z. Yang, T. Yao, Y. Li, Y. Lin, Y. Wu and Y. Li, Synergistic effect of well-defined dual sites boosting the oxygen reduction reaction, Energy Environ. Sci., 2018, 11, 3375–3379 RSC
.
- X. Meng, D. Li, L. Chen, H. He, Q. Wang, C. Hong, J. He, X. Gao, Y. Yang, B. Jiang, G. Nie, X. Yan, L. Gao and K. Fan, High-performance self-cascade pyrite nanozymes for apoptosis-ferroptos is synergistic tumor therapy, ACS Nano, 2021, 15, 5735–5751 CrossRef CAS PubMed
.
- L. Huang and S. Tepaamorndech, The SLC30 family of zinc transporters-a review of current understanding of their biological and pathophysiological roles, Mol. Aspects Med., 2013, 34, 548–560 CrossRef CAS PubMed
.
- X. Zeng, Y. Ruan, Q. Chen, S. Yan and W. Huang, Biocatalytic cascade in tumor microenvironment with a Fe2O3/Au hybrid nanozyme for synergistic treatment of triple negative breast cancer, Chem. Eng. J., 2023, 452, 138422 CrossRef CAS
.
- K. Li, C. Lin, M. Li, K. Xu, Y. He, Y. Mao, L. Lu, W. Geng, X. Li, Z. Luo and K. Cai, Multienzyme-like reactivity cooperatively impairs glutathione peroxidase 4 and ferroptosis suppressor protein 1 pathways in triple-negative breast cancer for sensitized ferroptosis therapy, ACS Nano, 2022, 16, 2381–2398 CrossRef CAS PubMed
.
- C. Wu, D. Xu, M. Ge, J. Luo, L. Chen, Z. Chen, Y. You, Y.-X. Zhu, H. Lin and J. Shi, Blocking glutathione regeneration: inorganic NADPH oxidase nanozyme catalyst potentiates tumoral ferroptosis, Nano Today, 2022, 46, 101574 CrossRef CAS
.
- Q. Xu, Y. Zhang, Z. Yang, G. Jiang, M. Lv, H. Wang, C. Liu, J. Xie, C. Wang, K. Guo, Z. Gu and Y. Yong, Tumor microenvironment-activated single-atom platinum nanozyme with H2O2 self-supplement and O2-evolving for tumor-specific cascade catalysis chemodynamic and chemoradiotherapy, Theranostics, 2022, 12, 5155–5171 CrossRef CAS PubMed
.
- H. He, L. Du, H. Xue, Y. An, K. Zeng, H. Huang, Y. He, C. Zhang, J. Wu and X. Shuai, Triple tumor microenvironment-responsive ferroptosis pathways induced by manganese-based imageable nanoenzymes for enhanced breast cancer theranostics, Small Methods, 2023, 7, 2300230 CrossRef CAS PubMed
.
- H. Kobayashi and P. L. Choyke, Near-infrared photoimmunotherapy of cancer, Acc. Chem. Res., 2019, 52, 2332–2339 CrossRef CAS PubMed
.
- K. Sun, J. Hu, X. Meng, Y. Lei, X. Zhang, Z. Lu, L. Zhang and Z. Wang, Reinforcing the induction of immunogenic cell death via artificial engineered cascade bioreactor-enhanced chemo-immunotherapy for optimizing cancer immunotherapy, Small, 2021, 17, 2101897 CrossRef CAS PubMed
.
- Y. Cao, S. Zhang, Z. Lv, N. Yin, H. Zhang, P. Song, T. Zhang, Y. Chen, H. Xu, Y. Wang, X. Liu, G. Zhao and H. Zhang, An intelligent nanoplatform for orthotopic glioblastoma therapy by nonferrous ferroptosis, Adv. Funct. Mater., 2022, 32, 2209227 CrossRef CAS
.
- B. Li, X. Chen, W. Qiu, R. Zhao, J. Duan, S. Zhang, Z. Pan, S. Zhao, Q. Guo, Y. Qi, W. Wang, L. Deng, S. Ni, Y. Sang, H. Xue, H. Liu and G. Li, Synchronous disintegration of ferroptosis defense axis via engineered exosome-conjugated magnetic nanoparticles for glioblastoma therapy, Adv. Sci., 2022, 9, 2105451 CrossRef CAS PubMed
.
- X. Chen, Y. Yang, G. Ye, S. Liu and J. Liu, Chiral ruthenium nanozymes with self-cascade reaction driven the NO generation induced macrophage M1 polarization realizing the Lung cancer “Cocktail Therapy”, Small, 2023, 19, 2207823 CrossRef CAS PubMed
.
- H. Yuan, P. Xia, X. Sun, J. Ma, X. Xu, C. Fu, H. Zhou, Y. Guan, Z. Li, S. Zhao, H. Wang, L. Dai, C. Xu, S. Dong, Q. Geng, Z. Li and J. Wang, Photothermal nanozymatic nanoparticles induce ferroptosis and apoptosis through tumor microenvironment manipulation for cancer therapy, Small, 2022, 18, 2202161 CrossRef CAS PubMed
.
- L. Wang, X. Zhang, Z. You, Z. Yang, M. Guo, J. Guo, H. Liu, X. Zhang, Z. Wang, A. Wang, Y. Lv, J. Zhang, X. Yu, J. Liu and C. Chen, A molybdenum disulfide nanozyme with charge-enhanced activity for ultrasound-mediated cascade-catalytic tumor ferroptosis, Angew. Chem., Int. Ed., 2023, 62, 7313–7321 Search PubMed
.
- L. Yao, M. M. Zhao, Q. W. Luo, Y. C. Zhang, T. T. Liu, Z. Yang, M. Liao, P. Tu and K. W. Zeng, Carbon quantum dots-based nanozyme from coffee induces cancer cell ferroptosis to activate antitumor immunity, ACS Nano, 2022, 16, 9228–9239 CrossRef CAS PubMed
.
- K. Li, K. Xu, Y. He, L. Lu, Y. Mao, P. Gao, G. Liu, J. Wu, Y. Zhang, Y. Xiang, Z. Luo and K. Cai, Functionalized tumor-targeting nanosheets exhibiting Fe(II) overloading and GSH consumption for ferroptosis activation in liver tumor, Small, 2021, 17, 2102046 CrossRef CAS PubMed
.
- S. Liu, W. Li, H. Ding, B. Tian, L. Fang, X. Zhao, R. Zhao, B. An, L. Ding, L. Zhong and P. Yang, Biomineralized RuO2 nanozyme with multi-enzyme activity for ultrasound-triggered peroxynitrite-boosted ferroptosis, Small, 2023, 2303057 CrossRef CAS PubMed
.
- S. S. Bashraheel, A. Domling and S. K. Goda, Update on targeted cancer therapies, single or in combination, and their fine tuning for precision medicine, Biomed. Pharmacother., 2020, 125, 110009 CrossRef CAS PubMed
.
- L. Chang, P. Ruiz, T. Ito and W. R. Sellers, Targeting pan-essential genes in cancer: challenges and opportunities, Cancer Cell, 2021, 39, 466–479 CrossRef CAS PubMed
.
- C. Cao, N. Yang, Y. Su, Z. Zhang, C. Wang, X. Song, P. Chen, W. Wang and X. Dong, Starvation, ferroptosis, and prodrug therapy synergistically enabled by a cytochrome c oxidase like nanozyme, Adv. Mater., 2022, 34, 2203236 CrossRef CAS PubMed
.
- R. Xu, J. Yang, Y. Qian, H. Deng, Z. Wang, S. Ma, Y. Wei, N. Yang and Q. Shen, Ferroptosis/pyroptosis dual-inductive combinational anti-cancer therapy achieved by transferrin decorated nanoMOF, Nanoscale Horiz., 2021, 6, 348–356 RSC
.
- X. Zhang, X. Chen and Y. Zhao, Nanozymes: versatile platforms for cancer diagnosis and therapy, Nano-Micro Lett., 2022, 14, 95 CrossRef CAS PubMed
.
- S. Doll, F. P. Freitas, R. Shah, M. Aldrovandi, M. C. da Silva, I. Ingold, A. Goya Grocin, T. N. Xavier da Silva, E. Panzilius, C. H. Scheel, A. Mourão, K. Buday, M. Sato, J. Wanninger, T. Vignane, V. Mohana, M. Rehberg, A. Flatley, A. Schepers, A. Kurz, D. White, M. Sauer, M. Sattler, E. W. Tate, W. Schmitz, A. Schulze, V. O’Donnell, B. Proneth, G. M. Popowicz, D. A. Pratt, J. P. F. Angeli and M. Conrad, FSP1 is a glutathione-independent ferroptosis suppressor, Nature, 2019, 575, 693–698 CrossRef CAS PubMed
.
- T. Nakamura, C. Hipp, A. Santos Dias Mourão, J. Borggräfe, M. Aldrovandi, B. Henkelmann, J. Wanninger, E. Mishima, E. Lytton, D. Emler, B. Proneth, M. Sattler and M. Conrad, Phase separation of FSP1 promotes ferroptosis, Nature, 2023, 619, 371–377 CrossRef CAS PubMed
.
- P. Koppula, L. Zhuang and B. Gan, Cystine transporter SLC7A11/xCT in cancer: ferroptosis, nutrient dependency, and cancer therapy, Protein Cell, 2021, 12, 599–620 CrossRef CAS PubMed
.
- D. F. Quail and J. A. Joyce, Microenvironmental regulation of tumor progression and metastasis, Nat. Med., 2013, 19, 1423–1437 CrossRef CAS PubMed
.
- R. Chen, J. Yang, M. Wu, D. Zhao, Z. Yuan, L. Zeng, J. Hu, X. Zhang, T. Wang, J. Xu and J. Zhang, M2 macrophage hybrid membrane-camouflaged targeted biomimetic nanosomes to reprogram inflammatory microenvironment for enhanced enzyme-thermo-immunotherapy, Adv. Mater., 2023, 35, 2304123 CrossRef CAS PubMed
.
- W. Zeng, M. Yu, T. Chen, Y. Liu, Y. Yi, C. Huang, J. Tang, H. Li, M. Ou, T. Wang, M. Wu and L. Mei, Polypyrrole nanoenzymes as tumor microenvironment modulators to reprogram macrophage and potentiate immunotherapy, Adv. Sci., 2022, 9, 2201703 CrossRef CAS PubMed
.
- A. D’Souza and R. Shegokar, Polyethylene glycol (PEG): a versatile polymer for pharmaceutical applications, Expert Opin. Drug Delivery, 2016, 13, 1257–1275 CrossRef PubMed
.
- Z. Zhao, S. Dong, Y. Liu, J. Wang, L. Ba, C. Zhang, X. Cao, C. Wu and P. Yang, Tumor microenvironment-activable manganese-boosted catalytic immunotherapy combined with PD-1 checkpoint blockade, ACS Nano, 2022, 16, 20400–20418 CrossRef CAS PubMed
.
- K. Bersuker, J. M. Hendricks, Z. Li, L. Magtanong, B. Ford, P. H. Tang, M. A. Roberts, B. Tong, T. J. Maimone, R. Zoncu, M. C. Bassik, D. K. Nomura, S. J. Dixon and J. A. Olzmann, The CoQ oxidoreductase FSP1 acts parallel to GPX4 to inhibit ferroptosis, Nature, 2019, 575, 688–692 CrossRef CAS PubMed
.
- V. A. N. Kraft, C. T. Bezjian, S. Pfeiffer, L. Ringelstetter, C. Müller, F. Zandkarimi, J. Merl-Pham, X. Bao, N. Anastasov, J. Kössl, S. Brandner, J. D. Daniels, P. Schmitt-Kopplin, S. M. Hauck, B. R. Stockwell, K. Hadian and J. A. Schick, GTP cyclohydrolase 1/tetrahydrobiopterin counteract ferroptosis through lipid remodeling, ACS Cent. Sci., 2020, 6, 41–53 CrossRef CAS PubMed
.
- P. Liao, W. Wang, W. Wang, I. Kryczek, X. Li, Y. Bian, A. Sell, S. Wei, S. Grove, J. K. Johnson, P. D. Kennedy, M. Gijón, Y. M. Shah and W. Zou, CD8+ T cells and fatty acids orchestrate tumor ferroptosis and immunity via ACSL4, Cancer Cell, 2022, 40, 365–378 CrossRef CAS PubMed
.
- Y. Liu, R. Niu, R. Deng, S. Song, Y. Wang and H. Zhang, Multi-enzyme co-expressed dual-atom nanozymes induce cascade immunogenic ferroptosis via activating interferon-γ and targeting arachidonic acid metabolism, J. Am. Chem. Soc., 2023, 145, 8965–8978 CrossRef CAS PubMed
.
- Z. Li, X. Lai, S. Fu, L. Ren, H. Cai, H. Zhang, Z. Gu, X. Ma and K. Luo, Immunogenic cell death activates the tumor immune microenvironment to boost the immunotherapy efficiency, Adv. Sci., 2022, 9, 2201734 CrossRef CAS PubMed
.
- S. Wu, L. Xu, C. He, P. Wang, J. Qin, F. Guo and Y. Wang, Lactate efflux inhibition by syrosingopine/LOD co-loaded nanozyme for synergetic self-replenishing catalytic cancer therapy and immune micro environment remodeling, Adv. Sci., 2023, 10, 2300686 CrossRef CAS PubMed
.
- E. J. Park, S. Y. Oh, S. J. Lee, K. Lee, Y. Kim, B. S. Lee and J. S. Kim, Chronic pulmonary accumulation of iron oxide nanoparticles induced Th1-type immune response stimulating the function of antigen-presenting cells, Environ. Res., 2015, 143, 138–147 CrossRef CAS PubMed
.
- J. Zhang, J. Yang, T. Zuo, S. Ma, N. Xokrat, Z. Hu, Z. Wang, R. Xu, Y. Wei and Q. Shen, Heparanase-driven sequential released nanoparticles for ferroptosis and tumor microenvironment modulations synergism in breast cancer therapy, Biomaterials, 2021, 266, 120429 CrossRef CAS PubMed
.
- R. Tang, J. Xu, B. Zhang, J. Liu, C. Liang, J. Hua, Q. Meng, X. Yu and S. Shi, Ferroptosis, necroptosis, and pyroptosis in anticancer immunity, J. Hematol. Oncol., 2020, 13, 110 CrossRef PubMed
.
- B. Ji, M. Wei and B. Yang, Recent advances in nanomedicines for photodynamic therapy (PDT)-driven cancer immunotherapy, Theranostics, 2022, 12, 434–458 CrossRef CAS PubMed
.
- H. He, L. Du, H. Xue, Y. An, K. Zeng, H. Huang, Y. He, C. Zhang, J. Wu and X. Shuai, Triple tumor microenvironment-responsive ferroptosis pathways induced by manganese-based imageable nanoenzymes for enhanced breast cancer theranostics, Small Methods, 2023, 7, 2300230 CrossRef CAS PubMed
.
- J. Xie, Y. Wang, W. Choi, P. Jangili, Y. Ge, Y. Xu, J. Kang, L. Liu, B. Zhang, Z. Xie, J. He, N. Xie, G. Nie, H. Zhang and J. S. Kim, Overcoming barriers in photodynamic therapy harnessing nano-formulation strategies, Chem. Soc. Rev., 2021, 50, 9152–9201 RSC
.
- W. Wu, Y. Pu and J. Shi, Nanomedicine-enabled chemotherapy-based synergetic cancer treatments, J. Nanobiotechnol., 2022, 20, 4 CrossRef CAS PubMed
.
- W. L. Pan, Y. Tan, W. Meng, N. H. Huang, Y. B. Zhao, Z. Q. Yu, Z. Huang, W. H. Zhang, B. Sun and J. X. Chen, Microenvironment-driven sequential ferroptosis, photodynamic therapy, and chemotherapy for targeted breast cancer therapy by a cancer-cell-membrane-coated nanoscale metal-organic framework, Biomaterials, 2022, 283, 121449 CrossRef CAS PubMed
.
- Y. Ye, H. Yu, B. Chen, Y. Zhao, B. Lv, G. Xue, Y. Sun and J. Cao, Engineering nanoenzymes integrating iron-based metal organic framework s with Pt nanoparticles for enhanced Photodynamic-Ferroptosis therapy, J. Colloid Interface Sci., 2023, 645, 882–894 CrossRef CAS PubMed
.
- Z. Liu, Q. Liu, H. Zhang, X. Zhang, J. Wu, Z. Sun, M. Zhu, X. Hu, T. Qi and H. J. A. F. M. Kang, Genetically engineered protein corona-based cascade nanozymes for enhanced tumor therapy, Adv. Funct. Mater., 2022, 32, 2208513 CrossRef CAS
.
- Z. He, H. Zhou, Y. Zhang, X. Du, S. Liu, J. Ji, X. Yang and G. Zhai, Oxygen-boosted biomimetic nanoplatform for synergetic phototherapy/ferroptosis activation and reversal of immune-suppressed tumor microenvironment, Biomaterials, 2022, 290, 121832 CrossRef CAS PubMed
.
- M. Wu, W. Ling, J. Wei, R. Liao, H. Sun, D. Li, Y. Zhao and L. Zhao, Biomimetic photosensitizer nanocrystals trigger enhanced ferroptosis for improving cancer treatment, J. Controlled Release, 2022, 352, 1116–1133 CrossRef CAS PubMed
.
- Y. J. Hou, X. X. Yang, R. Q. Liu, D. Zhao, C. X. Guo, A. C. Zhu, M. N. Wen, Z. Liu, G. F. Qu and H. X. Meng, Pathological mechanism of photodynamic therapy and photothermal therapy based on nanoparticles, Int. J. Nanomed., 2020, 15, 6827–6838 CrossRef CAS PubMed
.
- A. G. Denkova, R. M. de Kruijff and P. Serra-Crespo, Nanocarrier-mediated photochemotherapy and photoradiotherapy, Adv. Healthcare Mater., 2018, 7, 1701211 CrossRef PubMed
.
- D. Zhi, T. Yang, J. O’Hagan, S. Zhang and R. F. Donnelly, Photothermal therapy, J. Controlled Release, 2020, 325, 52–71 CrossRef CAS PubMed
.
- J. Wu, T. Liu, Z. Rios, Q. Mei, X. Lin and S. Cao, Heat shock proteins and cancer, Trends Pharmacol. Sci., 2017, 38, 226–256 CrossRef CAS PubMed
.
- A. Farzin, S. A. Etesami, J. Quint, A. Memic and A. Tamayol, Magnetic nanoparticles in cancer therapy and diagnosis, Adv. Healthcare Mater., 2020, 9, 1901058 CrossRef CAS PubMed
.
- E. Boisselier and D. Astruc, Gold nanoparticles in nanomedicine: preparations, imaging, diagnostics, therapies and toxicity, Chem. Soc. Rev., 2009, 38, 1759–1782 RSC
.
- P. Roszczenko, O. K. Szewczyk, R. Czarnomysy, K. Bielawski and A. Bielawska, Biosynthesized gold, silver, palladium, platinum, copper, and other transition metal nanoparticles, Pharmaceutics, 2022, 14, 2286 CrossRef CAS PubMed
.
- M. Chang, Z. Hou, M. Wang, C. Yang, R. Wang, F. Li, D. Liu, T. Peng, C. Li and J. Lin, Single-atom Pd nanozyme for ferroptosis-boosted mild-temperature photothermal therapy, Angew. Chem., Int. Ed., 2021, 60, 12971–12979 CrossRef CAS PubMed
.
- L. Shen, D. Ye, H. Zhao and J. Zhang, Perspectives for single-atom nanozymes: advanced synthesis, functional mechanisms, and biomedical applications, Anal. Chem., 2021, 93, 1221–1231 CrossRef CAS PubMed
.
- Y. Wei, Z. Wang, J. Yang, R. Xu, H. Deng, S. Ma, T. Fang, J. Zhang and Q. Shen, Reactive oxygen species/photothermal therapy dual-triggered biomimetic gold nanocages nanoplatform for combination cancer therapy via ferroptosis and tumor-associated macrophage repolarization mechanism, J. Colloid Interface Sci., 2022, 606, 1950–1965 CrossRef CAS PubMed
.
- L. Xie, J. Li, G. Wang, W. Sang, M. Xu, W. Li, J. Yan, B. Li, Z. Zhang, Q. Zhao, Z. Yuan, Q. Fan and Y. Dai, Phototheranostic metal-phenolic networks with antiexosomal PD-L1 enhanced ferroptosis for synergistic immunotherapy, J. Am. Chem. Soc., 2022, 144, 787–797 CrossRef CAS PubMed
.
- J. L. Liang, X. K. Jin, S. M. Zhang, Q. X. Huang, P. Ji, X. C. Deng, S. X. Cheng, W. H. Chen and X. Z. Zhang, Specific activation of cGAS-STING pathway by nanotherapeutics-mediated ferroptosis evoked endogenous signaling for boosting systemic tumor immunotherapy, Sci. Bull., 2023, 68, 622–636 CrossRef CAS PubMed
.
- J. Li, C. Xie, J. Huang, Y. Jiang, Q. Miao and K. Pu, Semiconducting polymer nanoenzymes with photothermic activity for enhanced cancer therapy, Angew. Chem., Int. Ed., 2018, 57, 3995–3998 CrossRef CAS PubMed
.
- J. Li and K. Pu, Semiconducting polymer nanomaterials as near-infrared photoactivatable protherapeutics for cancer, Acc. Chem. Res., 2020, 53, 752–762 CrossRef CAS PubMed
.
- M. Chang, Z. Hou, M. Wang, D. Wen, C. Li, Y. Liu, Y. Zhao and J. Lin, Cu single atom nanozyme based high-efficiency mild photothermal therapy through cellular metabolic regulation, Angew. Chem., Int. Ed., 2022, 61, 202209245 CrossRef PubMed
.
- X. Zeng, Y. Ruan, Q. Chen, S. Yan and W. Huang, Biocatalytic cascade in tumor microenvironment with a Fe2O3/Au hybrid nanozyme for synergistic treatment of triple negative breast cancer, Chem. Eng. J., 2023, 452, 138422 CrossRef CAS
.
- H. Wang, Y. Shen, L. Chen, K. Li, Y. Shi, Z. Xu, D. Li, H. Chen, W. Wang and L. Gao, Enhancing catalase-like activity of Prussian blue nanozyme by gadolinium-doping for imaging-guided antitumor amplification via photodynamic therapy and chemotherapy, Mater. Today, NANO, 2023, 22, 100326 CAS
.
- Y. Liu, M. Ji and P. Wang, Recent advances in small copper sulfide nanoparticles for molecular imaging and tumor therapy, Mol. Pharmaceutics, 2019, 16, 3322–3332 CrossRef CAS PubMed
.
- G. Lei, C. Mao, Y. Yan, L. Zhuang and B. Gan, Ferroptosis, radiotherapy, and combination therapeutic strategies, Protein Cell, 2021, 12, 836–857 CrossRef CAS PubMed
.
- M. Gao, J. Yi, J. Zhu, A. M. Minikes, P. Monian, C. B. Thompson and X. Jiang, Role of mitochondria in ferroptosis, Mol. Cell, 2019, 73, 354–363 CrossRef CAS PubMed
.
- W. Gao, X. Wang, Y. Zhou, X. Wang and Y. Yu, Autophagy, ferroptosis, pyroptosis, and necroptosis in tumor immunotherapy, Signal Transduction Targeted Ther., 2022, 7, 196 CrossRef PubMed
.
- H. Isozaki, R. Sakhtemani, A. Abbasi, N. Nikpour, M. Stanzione, S. Oh, A. Langenbucher, S. Monroe, W. Su, H. F. Cabanos, F. M. Siddiqui, N. Phan, P. Jalili, D. Timonina, S. Bilton, M. Gomez-Caraballo, H. L. Archibald, V. Nangia, K. Dionne, A. Riley, M. Lawlor, M. K. Banwait, R. G. Cobb, L. Zou, N. J. Dyson, C. J. Ott, C. Benes, G. Getz, C. S. Chan, A. T. Shaw, J. F. Gainor, J. J. Lin, L. V. Sequist, Z. Piotrowska, B. Y. Yeap, J. A. Engelman, J. J. Lee, Y. E. Maruvka, R. Buisson, M. S. Lawrence and A. N. Hata, Therapy-induced APOBEC3A drives evolution of persistent cancer cells, Nature, 2023, 620, 393–401 CrossRef CAS PubMed
.
- S. Veeranarayanan and T. Maekawa, External stimulus responsive inorganic nanomaterials for cancer theranostics, Adv. Drug Delivery Rev., 2019, 138, 18–40 CrossRef PubMed
.
- G. Lei, L. Zhuang and B. Gan, Targeting ferroptosis as a vulnerability in cancer, Nat. Rev. Cancer, 2022, 22, 381–396 CrossRef CAS PubMed
.
- M. Lyu, D. Zhu, X. Kong, Y. Yang, S. Ding, Y. Zhou, H. Quan, Y. Duo and Z. Bao, Glutathione-depleting nanoenzyme and glucose oxidase combination for hypoxia modulation and radiotherapy enhancement, Adv. Healthcare Mater., 2020, 9, 1901819 CrossRef PubMed
.
- K. Tomita, T. Nagasawa, Y. Kuwahara, S. Torii, K. Igarashi, M. H. Roudkenar, A. M. Roushandeh, A. Kurimasa and T. Sato, MiR-7-5p is involved in ferroptosis signaling and radioresistance thru the generation of ROS in radioresistant HeLa and SAS cell lines, Int. J. Mol. Sci., 2021, 22, 8300 CrossRef CAS PubMed
.
- M. Lyu, M. Luo, J. Li, O. U. Akakuru, X. Fan, Z. Cao, K. Fan and W. Jiang, Personalized Carbon Monoxide-Loaded Biomimetic Single-Atom Nanozyme for Ferroptosis-Enhanced FLASH Radioimmunotherapy, Adv. Funct. Mater., 2023, 33, 2306930 CrossRef
.
- J. Choi, G. Kim, S. B. Cho and H. J. Im, Radiosensitizing high-Z metal nanoparticles for enhanced radiotherapy of glioblastoma multiforme, J. Nanobiotechnol., 2020, 18, 122 CrossRef PubMed
.
- F. Vilotte, R. Jumeau and J. Bourhis, High Z nanoparticles and radiotherapy: a critical view, Lancet Oncol., 2019, 20, 557 CrossRef PubMed
.
- L. Gong, Y. Zhang, C. Liu, M. Zhang and S. Han, Application of radiosensitizers in cancer radiotherapy, Int. J. Nanomed., 2021, 16, 1083–1102 CrossRef PubMed
.
Footnote |
† These authors contributed equally to this work. |
|
This journal is © the Partner Organisations 2024 |