DOI:
10.1039/D3QM00638G
(Review Article)
Mater. Chem. Front., 2024,
8, 179-191
High-entropy catalysts: new opportunities toward excellent catalytic activities
Received
5th June 2023
, Accepted 31st August 2023
First published on 6th September 2023
Abstract
High-entropy catalysts (HECs) have been increasingly used in various applications in recent years. Owing to their multi-element composition and high-entropy mixing structure, HECs exhibit excellent catalytic performance in thermocatalysis, electrocatalysis, and photocatalysis. This comprehensive review focuses on the synthesis and catalytic applications of high-entropy alloys (HEAs), high-entropy oxides (HEOs), high-entropy sulfides (HESs), and other high-entropy catalytic systems. In this review, we point out several issues that require further exploration, including the controllable synthesis of HECs, identification of active centers, and regulation of elemental composition and structure of HECs. We hope that this review can help to further advance the development of HECs, in terms of optimizing the catalytic activities of HECs. Moreover, the “high-entropy” design concept behind these materials may also provide insights and solutions for addressing the challenges encountered in traditional simple systems.
1. Introduction
In recent years, high-entropy catalysts (HECs) have garnered enormous attention as a novel class of catalysts. High-entropy materials are flexible and inclusive, typically encompassing solid solutions with five or more elements that are uniformly mixed.1–7 The design strategy of these materials emphasizes the uniform and chaotic mixing of all constituent elements, without any dominant elemental species. This arrangement and combination of different elements provides ample opportunities for the development and design of new materials, performance optimization, and advanced applications. For instance, the flexibility of high-entropy material composition allows easy alteration of the geometric and electronic structures of the catalysts, ultimately regulating catalytic activity and product selectivity.8 In addition, the synergistic effect of multiple elements provides different adsorption sites, enabling optimal adsorption of reaction intermediates and achieving ideal reaction rates.9 Furthermore, high-entropy materials also exhibit excellent catalytic stability, thanks to the effects of high-entropy and hysteresis diffusion, rendering them more resilient under extreme reaction conditions including a high/low temperature environment and a strong acidic/alkaline solution.10 Early reports on high-entropy alloys (HEAs) demonstrated their unique advantages in various application environments but did not provide a universal synthesis strategy or a detailed structural understanding of these diverse materials with five or more elements. As the number of elements increases, the phenomenon of immiscibility becomes more pronounced, making it easier to form heterogeneous structures with phase separation. Hence, a higher operating temperature is required to overcome the thermodynamic barriers for achieving a uniform mixing of different elements.11,12 To overcome this challenge, a thermal shock strategy has been employed, which induces high-temperature mixing of multiple elements, allowing even immiscible elements to form a uniformly mixed solid solution structure. This ultra-fast heating–cooling method overcomes the thermodynamic barriers for the formation of nanoparticles with high-entropy mixing, rather than heterogeneous structures with phase separation. Initially applied for the synthesis of HEAs, this “impact” method has been successfully employed for the synthesis of various high-entropy materials, including oxides,1,13,14 sulfides,15–17 carbides,18 diboride and halides.19,20
Despite being relatively new materials, high-entropy materials have shown significant potential in a myriad of energy and environmental applications, particularly in catalysis.12,21–27 For instance, AlFeMnTiM (M = Cr, Co, and Ni) HECs have shown exceptional degradation efficiency for azo dyes, in particular the AlFeMnTiCr catalyst demonstrated a significant 19-fold and 100-fold enhancement over the commercial Fe–Si–B amorphous alloy catalysts and the commercial zero valent iron catalysts, respectively. These findings are fascinating as the degradation of azo dyes is one of the most important reactions in the treatment of industrial wastewater and environmental pollution.28,29 Our recent work has also demonstrated that high-entropy sulfides (CdZnCuCoFe)Sx, a new class of photocatalysts, can photocatalytically convert biomass polysaccharides (cellulose, starch, and guar gum) into carbon monoxide (CO), an important component of syngas, under mild reaction conditions with a product selectivity of up to 99%.15 This study provides new insights into the development of novel photocatalysts for selective conversion of low value biomass into a valuable platform chemical. Besides, other studies have explored the origin and active centers of high-entropy systems that render their excellent catalytic performance. Based on the literature, FeCoNiXRu (X: Cu, Cr, and Mn) HEAs possess abundant active sites with different adsorption and dissociation energies for various intermediates, overcoming the limitations of single-element catalysts.30 Besides, the stark difference in the electronegativity of different elements in the system induced significant charge redistribution, leading to highly active Co and Ru sites that greatly improved the efficiency of water dissociation under alkaline conditions. In a separate study, Hu et al. reported on the synthesis of single-phase high-entropy oxide (HEO) nanoparticles using conductive carbon nanofibers as carriers through rapid high-temperature heating.31 Through this method, the elements were uniformly mixed without significant element segregation or phase separation. In comparison with nanoparticles that consist of fewer elements, oxide nanoparticles that are made up of ten types of metal elements can resolve the issue of catalyst deactivation in methane combustion. These examples illustrate the promising prospects of high-entropy materials in catalysis.
Compared to materials with relatively simple compositions, high-entropy materials consist of a large number of components, resulting in a vast number of atomic arrangement patterns and expanding the possibilities for material development and utilization. Moreover, the four core effects of high-entropy materials – the synergistic effect of multiple elements, lattice distortion effect, hysteresis diffusion effect, and high-entropy effect – confer them with excellent mechanical stability, excellent thermal stability, acid and alkali resistance, high temperature resistance, corrosion resistance, and high hardness. These inherent properties surpass those of traditional metal materials in many aspects.5,7,23,32–38 Moreover, the design concept of “high-entropy” holds promise for the discovery and design of other high-performance and cost-effective catalytic materials, with potential applications across diverse material fields. However, the multi-element composition and complex atomic arrangement of high-entropy materials also pose challenges for material screening and characterization. Therefore, this review focuses on introducing HEAs, HEOs, HESs, and other novel high-entropy catalytic systems. In addition to providing an overview of these materials, this review delves into the opportunities, challenges, and prospects associated with this emerging and highly promising field.
2. Synthesis of high-entropy catalysts
With the development of nanoscience and nanotechnology in recent years, many research efforts have been devoted to developing different synthesis strategies for the preparation of nanostructured high-entropy materials, and these approaches can be systematically categorized into the thermal shock method, wet chemical method, and cation exchange method.23,39 The first type is a kinetically driven process that requires harsh reaction conditions, such as high temperature, high pressure, and inert atmosphere protection. The most typical example of this method is the carbon thermal shock method, which was first proposed by Hu et al. (Fig. 1A).12,31,40 The synthesis of HECs involves two consecutive simple steps:1 complete dissolving of the required metal salt precursor in solution, followed by accurate distribution of the droplets in the carbon base pore groove using a syringe;2 conducting thermal shock using ultrafast heating and cooling rates at extremely high temperature to shorten the reaction time. Hence, this method serves as a potential generic approach for rapid and controllable synthesis of high-entropy nanoparticles. In the non-equilibrium state of ultrafast heating and cooling, the formation of high-entropy nanoparticles is ensured within a growth time of seconds or on a shorter scale (Fig. 1B). As shown in Fig. 1C, HEA (PtPdCoNiFeCuAuSn) nanoparticles composed of up to eight metal elements have been successfully prepared. Initially, carbon thermal shock was employed to prepare HEA nanoparticles, but now it has been extended to the synthesis of HEO and HES nanoparticles. Similar to the “impact” concept, researchers have developed various methods for achieving controllable synthesis of high-entropy nanoparticles, including the pulse laser scanning method and the nickel foil Joule heating strategy.41,42 When synthesizing HEOs using carbon nanofibers as the carrier for carbon thermal shock, it is worth noting that many metal oxides tend to be reduced by carbon nanofibers at high temperature in an argon atmosphere. To address this issue, nickel foil can be chosen as the carrier for Joule heating (Fig. 1D). Nickel, an inexpensive metal with moderate conductivity, plasticity, and melting point, can be transformed into a desired shape under specific conditions to sustain high temperature. Furthermore, nickel exhibits lower reactivity among metals, thereby preventing the reduction of metal oxides at high temperatures. Building upon this Joule heating technology, a series of HEO systems, including rock salt oxides, spinel oxides, and perovskite oxides, have been developed.
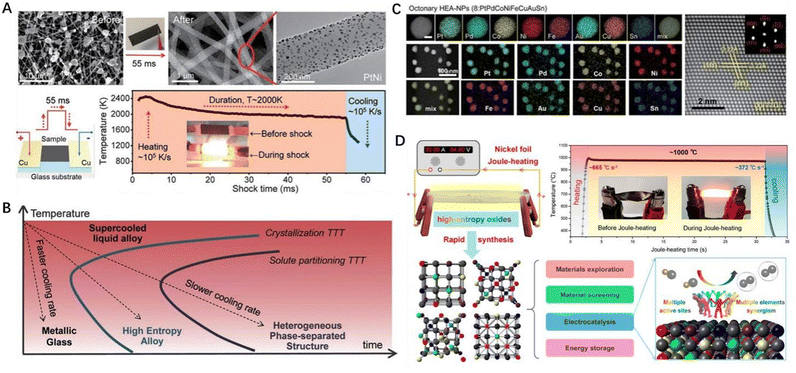 |
| Fig. 1 (A) The process of preparing HEA nanoparticles using the carbon thermal shock method and microscopic images of the samples before and after carbon thermal shock. (B) Dynamic control of the formation of HEA nanoparticles. (C) Single and low magnification elemental maps as well as high-resolution HAADF-STEM images showing the Fcc structure of HEA nanoparticles. (A) to (C) are reproduced with permission from the American Association for the Advancement of Science.19 (D) Schematic diagram to show the presence of multiple active sites on HEOs prepared by Joule heating and the principle diagram of synergistic catalysis of HEOs. Reproduced with permission from the American Chemical Society.41 | |
The second type, the wet chemical method, focuses on the creation of local extreme reaction conditions through a hydrothermal/solvothermal process or ball milling methods.43–45 While these methods facilitate uniform mixing of different elements at high temperatures, studies have shown that high-entropy nanoparticles can also be formed at lower temperatures. The mechanical ball milling method allows for uniform mixing of precursors, without the need for solvents or with only a minimal amount of solvent added. Fig. 2C shows that low-temperature solvothermal synthesis can also induce the formation of high-entropy nanoparticles. The successful synthesis of high-entropy nanoparticles at low temperature was divulged by high angle annular dark field transmission electron microscopy (HAADF-STEM), in which various elements including Cd, Zn, Cu, Co, Fe, and S are uniformly distributed in (CdZnCuCoFe)Sx (Fig. 2D). These methods offer mild reaction conditions, simple operation, and scalability. However, due to the significant differences in the physical and chemical properties among different elements, the use of these methods to synthesize high-entropy nanoparticles typically results in phase separation structures. Only elements with similar properties can form a single-phase solid solution structure, limiting the development and design of multi-element nanoparticles. In contrast, by utilizing carbon thermal shock and high temperature thermal shock, multi-element nanoparticles with a wide range of elements can be synthesized.
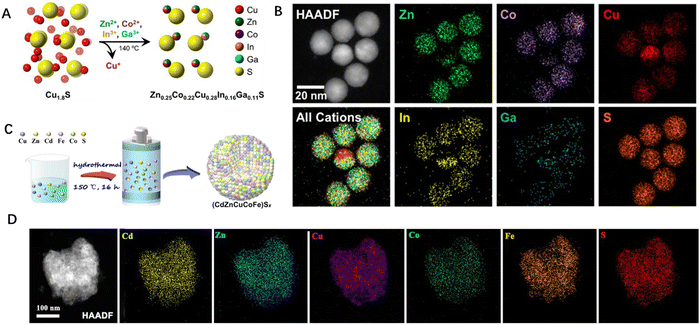 |
| Fig. 2 (A) Schematic diagram to illustrate the formation of Zn0.25Co0.22Cu0.28In0.16Ga0.11S. (B) HAADF-STEM and the corresponding STEM-EDS elemental diagram for the colloidal nanoparticles after one minute of the cation exchange reaction before quenching. (C) Schematic illustration for the synthesis of (CdZnCuCoFe)Sx. (D) HAADF-STEM image of (CdZnCuCoFe)Sx. Reproduced with permission from the Royal Society of Chemistry.15 Reproduced with permission from the American Chemical Society.16 | |
The third type, the cation exchange method, involves soft–hard acid–base interactions between metal cation species and molecular ligands, such as the colloidal nanoparticle cation exchange method (Fig. 2A).16,21 This method enables the synthesis of high-entropy colloidal nanoparticles at low temperatures, and the stoichiometry of the product can be adjusted by varying the proportion of cation exchange in the solution. The cation exchange process for forming HESs is considered as a powerful reaction in terms of entropy and enthalpy.46,47 Initially, the simultaneous cation exchange method was primarily used for the synthesis of high-entropy metal sulfide colloidal nanoparticles. Previous research studies have shown that this method also holds promise for extension to other high-entropy systems, including metal selenides, metal phosphides, metal tellurides, and metal halides.48–51 As depicted in Fig. 2B, Schaak and co-workers separated the HAADF-STEM image and STEM-EDS elemental diagram of the particles quenched in one minute after the reaction in order to better understand the formation process of high-entropy metal sulfide nanoparticles. Even at this early stage, most particles appeared to be high-entropy metal sulfides, indicating that the simultaneous cation exchange reaction occurs nearly instantaneously and is almost complete.
3. High-entropy alloys for catalysis
In comparison to the traditional single component metal and multi-component metal alloys with less than five elements, HEA catalysts have recently received extensive attention due to their merits of strong catalytic activity, high product selectivity, and good durability.10,52,53 This section focuses on the latest research progress of nanostructured HEAs in the fields of thermal catalysis and electrocatalysis.
3.1 Thermocatalysis
Ruthenium (Ru), a noble metal, has been considered as one of the most active metals for ammonia decomposition. However, it is economically infeasible to apply it on a large-scale owing to its high cost and scarcity. To address this issue, researchers have explored HEAs composed of abundant elements on Earth to overcome this limitation. For instance, a low-cost Co Mo binary alloy system with mixed Co Mo sites has been found to be a promising catalyst for ammonia decomposition.54 However, the large miscibility gaps in the phase diagram of Co Mo binary alloys pose a great challenge. Wang and co-workers addressed this issue by using entropy-induced stabilization in HEAs. They developed a novel CoMoFeNiCu HEA catalyst using carbon thermal shock technology, which exhibited enhanced efficiency for ammonia decomposition. The regulation of the Co Mo ratio in HEAs transcends the scope of the traditional binary Co Mo alloy phase diagram, breaking the miscibility limit of bimetallic Co Mo alloys (Fig. 3A).52 By controlling the Co Mo ratio, the surface performance of the HEA catalysts can be optimized for different reaction conditions, leading to remarkable stability and catalytic activity for ammonia decomposition (Fig. 3B). In addition, multi-element nanoparticles exhibit excellent structural and thermal stability along with promising performance in catalyzing NH3 decomposition.10 As shown in Fig. 3C, single-phase Ru-4 MEA (RuRhCoNi) and Ru-5 MEA (RuRhCoNiIr) can be prepared by carbon thermal shock technology. When the temperature elevated to 470 °C, a nearly 100% NH3 decomposition efficiency can be achieved using Ru-4 MEA nanoparticles, which is significantly higher than the conversion efficiency of pure Ru and ternary RuCoNi samples. This indicates the importance of composition control and uniform mixing for performance optimization. Despite the same composition, the performance of Ru-5 MEA-NPs is significantly superior than that of the Ru-5 IMP sample, indicating that the improvement in performance mainly originated from the alloy structure rather than simple element mixing. As shown in Fig. 3D, the conversion efficiency of RuRhCoNi MEA is much higher than that of the Ru + RhCoNi sample that was prepared by simple mixing, indicating that the RuRhCoNi MEA sample has a strong synergistic effect to improve the catalytic performance for NH3 decomposition.7,35 Further characterization was carried out on the MEA-NPs after the catalytic reaction. From the HAADF and EDS spectra, it was observed that Ru-4 MEA-NPs still retained good dispersibility and a phase-free alloy structure (Fig. 3E). The catalytic performance of MEA-NPs has been significantly improved, and the performance of MEA nanoparticles for the decomposition of NH3 is superior to that of the existing Ru-based catalysts reported in the literature (Fig. 3F), further highlighting the merits and feasiblity of multi-element alloy structures for the development of next-generation high-performance catalysts.
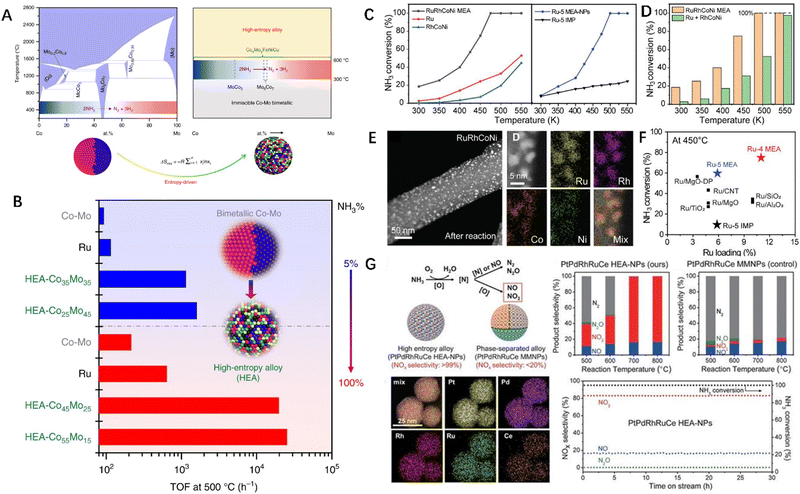 |
| Fig. 3 (A) Schematic showing HEA breaking the mixing limitation of traditional binary alloys. (B) Alteration of the HEA-CoxMoy catalyst composition to optimize the surface performance of NH3 decomposition at different concentrations. (C)–(F) Catalytic performance of Ru-4 and Ru-5 MEA-NPs for ammonia decomposition. (G) Catalytic performance of HEA-NPs for ammonia oxidation. Reproduced with permission from the Nature Publishing Group and Elsevier.52 Reproduced with permission from the American Association for the Advancement of Science.10 Reproduced with permission from the American Association for the Advancement of Science.12 | |
So far, PtPdRh-based multi-metal catalysts have been widely used in industry for ammonia oxidation, despite their extremely high cost. However, to promote the yield of NOx, a reaction temperature of 800 °C is required, which leads to the instability of the catalyst at high temperatures. Additionally, the immiscibility of the PtPdRh polymetallic system brings difficulties to the synthesis of its single-phase solid solution. Hu et al. alloyed eight different elements into single-phase solid solution nanoparticles through loading the metal salt mixture on the carbon carrier by thermal shock. They synthesized a wide range of multi-component nanoparticles with a certain chemical composition, size, and phase by controlling the carbon thermal excitation parameters such as temperature, substrate, duration of shock, and heating/cooling rate. To demonstrate the practicality of HEAs, researchers synthesized pentagonal HEA PtPdRhRuCe nanoparticles for ammonia oxidation catalysts, introducing Ru and Ce to improve overall catalytic activity and reduce the Pt content. The selectivity of industrial ammonia oxidation to produce nitric acid is crucial, and it aims to obtain NO and NO2 for nitric acid production. As shown in Fig. 3G, the sample is a high-entropy alloy with the same elemental composition as the control sample but no alloy structure is present. It was found that at a reaction temperature of 700 °C, the selectivity of the target component can reach over 99%, and the conversion rate can almost reach 100%. Compared to non-high-entropy alloys, the selectivity is only about 20%. Furthermore, HEA PtPdRhRuCe nanoparticles exhibit good stability and will not experience performance degradation even after continuous operation at 700 °C for 30 hours.12
3.2 Electrocatalysis
3.2.1 Hydrogen evolution reaction.
The hydrogen evolution reaction (HER) is a half-reaction of electrochemical water splitting, involving a multi-step reaction on a catalyst surface. Pt and Pd-based catalysts are known for their excellent catalytic activity in this reaction, achieving high hydrogen yield with minimal overpotential in acidic solutions, and they have been regarded as the most active HER catalysts.55 However, the expensiveness of precious metals greatly impedes their large-scale applications. Transition metals and their alloys, which possess partially filled d-orbitals suitable for electron transfer, are expected to exhibit high catalytic activity for hydrogen evolution. Preparing HEA catalysts containing these active metals can not only reduce costs but also maintain catalytic activity. However, the synthesis of uniformly dispersed nanoparticles on particle carriers such as carbon materials, γ-Al2O3 and zeolite is of great importance for practical applications, but further exploration is largely lacking. With the use of an improved fast moving bed pyrolysis method, up to ten elemental components of HEA (MnCoNiCuRhPdSnInPtAu) nanoparticles on narrow particle carriers can be synthesized.45 This strategy ensures that the mixed metal precursors can be rapidly and simultaneously pyrolyzed at high temperatures to produce small-sized nuclei. This method is significantly superior to the traditional fixed-bed pyrolysis method, which is more prone to the formation of phase-separated heterostructures. The representative five-element HEA FeCoPdIrPt nanoparticles show high stability for the HER with high mass activity, which is 26 times higher than that of commercial platinum carbon at 100 mV superpotential (Fig. 4D). In another example, Kitagawa et al. first synthesized a HEA IrPdPtRhRu catalyst composed of five platinum group metals using a one-pot polyol method. The experimental findings revealed that HEA IrPdPtRhRu nanoparticles exhibited higher HER activity in acidic and alkaline solutions compared to single-metal catalysts or even commercial Pt catalysts. In addition, Xu and co-workers also developed a series of HEA FeCoNiWMo catalysts, which cost only 0.04% of the commercial Pt/C electrodes. By varying the Co/Mo element ratio in the HEA FeCoNiWMo nanoparticles, a linear relationship between the content of ordered HEA nanoparticles and their hydrogen evolution activity was observed.56–58
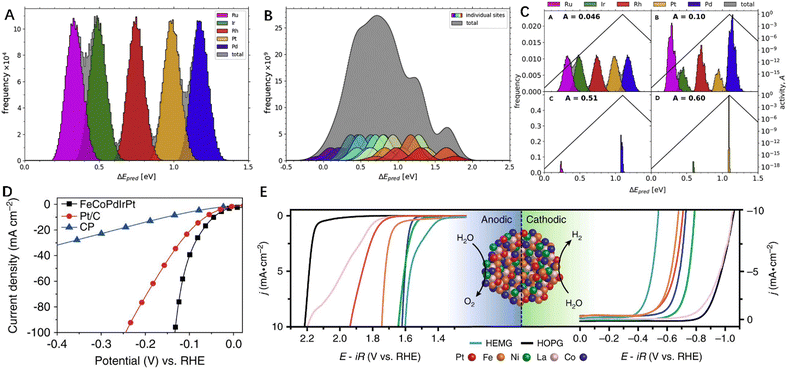 |
| Fig. 4 (A) and (B) The calculated adsorption energies of *OH and *O obtained from DFT, where each color represents a different binding site. (C) Activity of the recombinant HEA-IrPdPtRhRu composition. (D) HER activity of FeCoPdPtIr@GO synthesized by FMBP loaded on carbon paper (CP), Pt/C and pure carbon. (E) Cathode and anode polarization curves of the CoFeLaNiPt HEMG catalyst and its components. (A) and (B) are reproduced with permission from Elsevier.63 Reproduced with permission from the Nature Publishing Group (https://creativecommons.org/licenses/by/4.0/).45 Reproduced with permission from the Nature Publishing Group (http://creativecommons.org/licenses/by/4.0/).64 | |
3.2.2 Oxygen evolution reaction.
Other than the HER, the oxygen evolution reaction (OER) is another half-reaction of electrochemical water splitting. The OER involves complex four-electron/four-proton transfer steps and the formation of oxygen–oxygen bonds. Transition metal oxides or hydroxides, such as IrO2 and RuO2, are common OER catalysts. In order to reduce the amount of precious metals and the cost of catalysts, researchers have explored HEAs as catalysts for the OER. It is worth noting that the active sites of HEA catalysts used for the OER are the surface layers of the reconstructed oxides or hydroxides, rather than the original metal phase.59,60 The high catalytic activity of nanoporous HEAs is not only due to their porous structure facilitating gas transport on the surface but also due to the adsorption and stabilization of a large number of active intermediates by the oxide/hydroxide formed on their surface during the OER process. Researchers have reported the in situ synthesis of ultra-small HEA FeCoNiIrRu nanoparticles in electrospun carbon nanofibers (CNFs), and the in situ characterization results indicate that the formation process is thermodynamically induced phase evolution. This work also highlights that OER performance can be optimized by altering the metal composition and calcination temperature. In situ Raman spectroscopy disclosed the formation of hydroxyl (OH) and superoxide (OO) intermediates on the surface of HEA FeCoNiIrRu nanoparticles. Theoretical calculations show that the redistribution of electron density in HEA FeCoNiIrRu nanoparticles transfers from low electronegativity elements (Fe, Co, Ni) to high electronegativity elements (Ir, Ru), which enhances the OER activity at the Ir site and promotes the conversion of *OOH and the generation of O2.61 In addition, FeCoNiCuMn HEA electrocatalysts can serve as dual-functional electrocatalysts for the HER and the OER. DFT calculations suggest that the Cu site acts as the electron enrichment active site for the HER and the OER, which effectively reduces the adsorption energy of reactants, intermediates, and products, leading to enhanced electrocatalytic activity of HEAs.62
3.2.3 Oxygen reduction reaction.
The oxygen reduction reaction (ORR) also involves four-proton/electron transfers. Four-electron ORR is an important cathode reaction for the development of high-performance fuel cells, and it involves multiple intermediates (OOH*, OH*, and O*). Due to the imperfect ratio between OOH* and OH*, catalysts positioned at the top of the ORR volcano plot also exhibit an overpotential of 0.3–0.4 V, such as the widely studied Pt-based catalytic system. Therefore, the four-electron ORR requires improved electrocatalysts to increase the reaction rate.55 Rossmeisl used density functional theory to calculate the OH* and O* adsorption energies on the random subset of binding sites on the five-element HEA IrPdPtRhRu surface, and established an adsorption energy volcano plot. The adsorption energy can be adjusted and optimized by alloying (Fig. 4A–C).63 By optimizing the composition of HEAs, Ir10.2Pd32.0Pt9.30Rh19.6Ru28.9 was obtained. HEAS Ir10.2Pd32.0Pt9.30Rh19.6Ru28.9 possesses an active site with adsorption energy close to the peak value of the surface volcano curve, and its overvoltage is even lower than that of Pt/C. Therefore, alloying is an effective strategy to improve the ORR catalytic performance of Pt-based catalysts. Although the aforementioned HEA catalysts exhibited improved ORR catalytic activity, the cost of the catalysts is still high. Hence, it is of paramount importance to reduce the cost of ORR catalysts, especially considering their integration with practical devices such as fuel cells and metal–air batteries. Researchers have repeatedly attempted to prepare nano-porous HEAs with low platinum content.78,79 Dick and co-workers prepared pentagonal CoFeLaNiPt high-entropy metal glasses (HEMGs) with low crystallinity using the nanodroplet-mediated electrodeposition method. The HEMGs serve as multifunctional electrocatalysts which exhibit excellent activity and stability for both the HER and the OER (Fig. 4E).64 In addition, a convex cubic HEA Pt34Fe5Ni20Cu31Mo9Ru catalyst with abundant (310) crystal planes and a certain crystal size can be fabricated through a one-pot method. The performance tests show that the HEA Pt34Fe5Ni20Cu31Mo9Ru catalyst exhibits excellent electrocatalytic activity for the ORR, HER, and OER.65
4. High-entropy oxides for catalysis
In 2015, Maria et al. reported on single-phase multi-component oxides, where the composition and structure of the high-entropy rock salt oxides can be greatly affected by the synthesis temperature (Fig. 5A). High-entropy oxides (HEOs) consist of independent anionic and cationic sublattices, which increase structural diversity. They can adopt various structures, including high-entropy spinel-structured oxides, perovskite-structured oxides, fluorite-structured oxides, and rock salt-structured oxides. The catalytic applications of HEOs can be categorized into two types: HEOs as direct catalysts and HEOs as carriers. HEOs are particularly adept at anchoring transition metal and noble metal active species compared to other HECs.
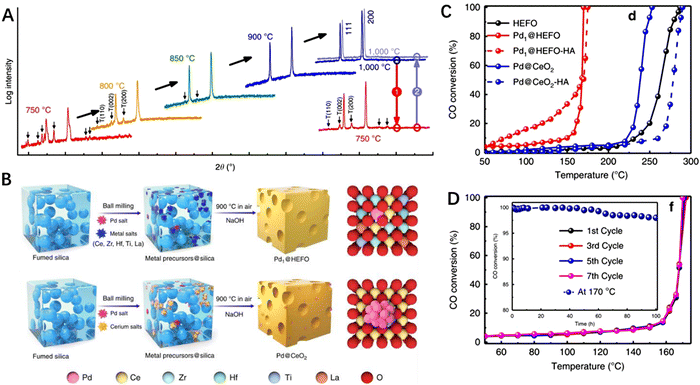 |
| Fig. 5 (A) XRD patterns of an equimolar mixture of MgO, NiO, ZnO, CuO, and CoO. (B) Schematic diagram illustrating the synthesis of Pd1@HEFO and Pd@CeO2. (C) CO conversion activity over various catalysts at different temperatures. (D) Stability test for CO conversion over Pd1@HEFO. (A) Reproduced with permission from the Nature Publishing Group.75 (B) to (D) Reproduced with permission from the Nature Publishing Group.74 | |
4.1 HEOs as catalysts
HEOs with rock salt and fluorite structures have been studied for thermal catalytic reactions. For example, classic rock salt HEOs, Mg0.2Co0.2Ni0.2Cu0.2Zn0.2O, applied in CO oxidation demonstrate increased Cu+ sites that can activate CO. The collisions between O2 and adsorbed CO molecules on Cu+ sites lead to CO oxidation.66 Fracchia et al. investigated the role of other elements in HEOs in CO oxidation and found that Cu species are active. Furthermore, an increase in configurational entropy can stabilize the reversibility of oxidation and reduction reactions over Cu species, which benefits the catalytic cycle of CO oxidation and showcases the intriguing advantage of HEOs in CO oxidation.67 To address the limitations of the small specific surface area and limited pores in traditional HEOs, Zhang et al. developed rock salt HEOs (CuNiFeCoMg) Ox–Al2O3 with abundant pores. With an increase in the SO2 concentration, the activity of the monometallic oxide CuO–Al2O3 significantly decreases. On the other hand, no noticeable deterioration in the performance of HEO–Al2O3 was observed, indicating that the rock salt structure of HEOs exhibits strong sulfur resistance in catalytic CO conversion.68 Notably, Cu-based HEOs exhibit strong resistance to SO2 toxicity. Additionally, MoNiCuZnCoOx HEOs with a rock salt structure exhibit high activity in the oxidative desulfurization of fuels and excellent stability even at high temperatures.69
Perovskite oxides are important catalysts for the OER. A novel lanthanum-based high-entropy perovskite oxide, La(CrMnFeCoNi)O3, synthesized using a simple coprecipitation method, outperforms single perovskite oxides in terms of performance. The optimized high-entropy perovskite oxide exhibits lower overvoltage and good electrochemical stability at a current density of 10 mA cm−2. High-entropy perovskite fluoride also exhibits stronger OER activity compared to single-component perovskites. To further enhance the electrocatalytic performance of oxide perovskites, perovskite oxide fluoride solid solutions have been reported, where all elements are uniformly dispersed to form a single-phase solid solution structure.70–72 Another study focuses on the development of high-entropy perovskite oxide La(Co0.2Mn0.2Fe0.2Ni0.2Cu0.2)O3 with a hollow spherical multilayer structure and oxygen rich vacancies.73 The experimental findings demonstrate that the material exhibits high electrochemical activity and good cycling stability. In a separate study, stable multi-component spinel oxides were successfully synthesized to activate porous spinel NiFe2O4 nanowires. The resulting spinel NiFeXO4(X = Fe, Ni, Al, Mo, Co, Cr) is superior to most spinel phase OER electrocatalysts and is comparable to state-of-the-art NiFe hydroxides. It maintains good stability during electrolysis for over 115 hours. Theoretical calculations indicate that NiFeXO4 possesses high configurational entropy, and their synergistic effect benefits the adsorption of H2O molecules, reducing the adsorption energy barrier of OOH* intermediates. The improved intrinsic activity and high nanopore structure contribute to excellent apparent catalytic performance.74 In addition, another study successfully prepared high-entropy spinel (Co, Cu, Fe, Mn, Ni)3O4 nanoparticles at low temperatures and anchored them on carbon nanotubes as efficient alkaline OER electrocatalysts.37
4.2 HEOs as supports
Presently, there are still significant challenges in preparing stable single atom catalysts on traditional carriers, especially under high temperature conditions. A novel entropy-driven strategy has been reported, combining mechanical grinding and 900 °C calcination to stabilize palladium (Pd) single atoms using high-entropy fluorite oxide (CeZrHfTiLa)Ox (HEFO) as the carrier (Fig. 5B); compared with the traditional carrier stabilized catalysts such as Pd@CeO2, Pd1@HEFO improves the reducibility of lattice oxygen, and stable Pd–O–M species exist, showing both high low temperature CO oxidation activity and excellent stability (Fig. 5C and D). This work demonstrates the superiority of high-entropy materials in the preparation of single atom catalysts.75 Adjusting the composition of HEOs to a certain extent can improve their ability to catalyze CO oxidation. For example, Chen et al. reported on the incorporation of Pt atoms into the NiMgCuZnCoOx rock salt structure, and the resulting PtNiMgCuZnCoOx demonstrated high stability in which no performance deterioration was observed after continuous operation at 135 °C for 40 hours.76 Following that, Dai et al. synthesized HEO supported noble metal catalysts under mild operating conditions with 5% loading of Ru and Pt into NiMgCuZnCoOx. Both catalysts exhibited high activity, selectivity, and temperature stability for selective reduction of CO2 into CO. Furthermore, Hu et al. achieved breakthroughs in the synthesis of multi-element oxide nanoparticles and developed a general and reliable strategy for controllable synthesis of multi-element oxide nanoparticles. Using the carbon thermal shock method, they systematically studied the effects of temperature, oxidation, and entropy on the synthesis of multi-element oxide nanoparticles with adjustable composition, size, and structure, providing guidance for the rational design and controllable synthesis of multi-element oxide nanoparticles. Compared to PdOx and five-element catalysts, the ten-element (ZrCe)0.6(MgLaYHfZrCrMn)0.3Pd0.1O2−x catalyst exhibited high performance and excellent thermal stability during methane combustion for 100 hours.31,77 To explore the interaction between precious metals and HEOs, Au was introduced into HEOs at 900 °C to prepare Au-based HEOs. At 700 °C, Au precipitated on the surface of the oxide, demonstrating self-regeneration and paving the way for the stable deposition of precious metal catalysts for high-temperature reactions.78,79
5. High-entropy sulfides for catalysis
5.1 Electrocatalysis
High-entropy sulfides (HESs), as a novel entropy-stable system, have also been designed and applied in catalytic reactions. Compared with the oxygen atom in HEOs, the P orbital of the sulfur atom in HESs has greater orbital overlap and energy matching with the d orbital of the metal, which introduces additional space for adjusting the electronic structures. Researchers synthesized HES loaded carbon fibers (CoZnCdCuMnS@CF) using the low-temperature cation exchange method. The catalyst exhibits good catalytic activity and excellent thermal stability for complete water hydrolysis in alkaline media. When it was applied as both the anode and cathode, it demonstrated a current density of 10 mA cm−2 at 1.63 V and no performance deterioration was observed after continuous operation for 73 hours.80 In addition, Hu et al. overcame the immiscibility of multiple elements by ultrafast pulse thermal decomposition and single-phase solid solution HES (CrMnFeCoNi)Sx nanoparticles with cubic structure were prepared. The five-component nanoparticles outperformed single- and multi-component (usually less than 5 types) sulfide-based nanoparticles in terms of OER activity, owing to the optimal adsorption of reaction intermediates according to the Sabatier principle (Fig. 6A). This was supported by the theoretical calculations and X-ray photoelectron spectroscopy (XPS) analysis, in which the strong synergistic effect between the metal atoms in the solid solution (CrMnFeCoNi)Sx nanoparticles led to enhanced OER activity and good electrochemical stability.40 In an another study, a series of novel FeNiCoCrXS2 (where X = Mn, Cu, Zn, or Al) HESs were synthesised using a two-step solvothermal method. It was found that FeNiCoCrMnS2 containing sulfate exhibited excellent OER activity, surpassing its corresponding monobasic, binary, ternary, and quaternary metals. Even at high current density, the electrocatalyst can maintain excellent stability and long-term durability after multiple cycles. The self-reconstruction of sulfides during the OER process was studied for the first time using various in situ and non in situ analysis methods, and the resulting metal (oxygen) hydroxides are considered the real active centers for the OER.81
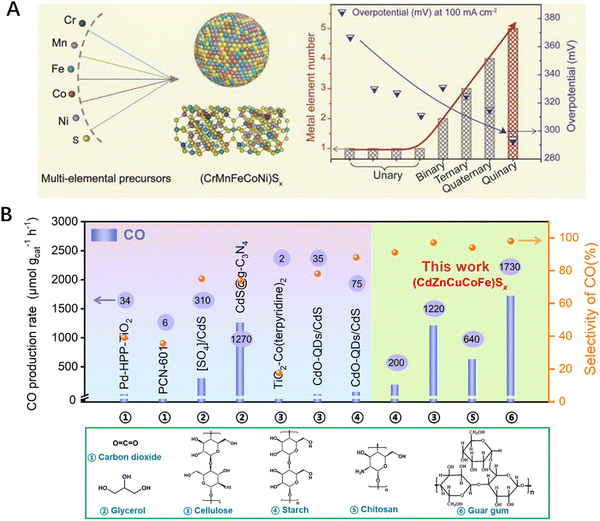 |
| Fig. 6 (A) Schematic diagram of the (CrMnFeCoNi)Sx structure and its overpotential in comparison to single, binary, ternary, and quaternary polymetallic sulphides. (B) The yield and selectivity of biomass glycan conversion into CO catalyzed by different catalysts. Reproduced with permission from John Wiley and Sons.40 Reproduced with permission from the Royal Society of Chemistry.15 | |
5.2 Photocatalysis
In recent years, the conversion of biomass into renewable fuels and value-added chemicals has received widespread attention. Photocatalysis is a promising biomass conversion technology, and there are increasing numbers of catalysts developed to meet this photocatalytic biomass upcycling. Initially, CdS-based photocatalysts were considered as the most promising photocatalysts due to their high catalytic efficiency and opportune bandgap (2.45 eV). Unfortunately, CdS-based catalysts have intrinsic drawbacks such as rapid recombination of photogenerated charge carriers along with their susceptibility to photocorrosion, which greatly limit their applications. At present, the construction of binary or ternary alloys is an effective anti-light corrosion strategy to improve the chemical stability of CdS. To date, there have been very limited studies on the use of sulfides with five or more equimolar ratio metal cations, namely high-entropy sulfides (HESs) for photocatalytic applications. This was first realized by Ye et al., in which HES (CdZnCuCoFe)Sx was synthesized using a one-step solvothermal method. Due to its unique ‘cocktail effect’, the elemental composition and surface geometry of (CdZnCuCoFe)Sx can optimize the active adsorption sites to maximize substrate activation and achieve the optimal catalytic activity. Under mild reaction conditions of ambient temperature and atmospheric pressure, efficient and selective photocatalytic conversion of biomass glycans was observed over the novel high-entropy sulfide (CdZnCuCoFe)Sx with the help of peroxide oxidants (Fig. 6B). Remarkably, a remarkable selectivity of up to 99.1% for CO was achieved, along with the generation of organic acids (formic acid and acetic acid) as by-products in the solution. This work provides a new route for selective conversion of biomass into CO, which not only helps to solve the imminent energy shortage issue but also serves as a reference for subsequent works on biomass conversion. Notably, the reaction can proceed under mild conditions – room temperature and atmospheric pressure, where the inexhaustible solar energy serves as the only energy input source.15
6. Other high-entropy catalysts
High entropy fluorides, nitrides, carbides, and phosphides have also been reported for a myriad of catalytic reactions.18,20,82,83 In an extreme non-equilibrium thermodynamic process, the sub-10 nm high-entropy (MoWVNbTa) C with unique microstructure is expected to replace the noble metal catalyst for hydrogen evolution. In addition, researchers have successfully prepared high-entropy metal nitrides using the mechanochemical-assisted soft urea strategy, with Cr, Nb, Mo, V, and Zr metal components highly dispersed in the cubic crystal structure of metal nitrides (Fig. 7A). Currently, the low conductivity of ABF3-type perovskite fluoride limits its application in the OER. To this, Dai and co-workers have realized the simple synthesis of high-entropy perovskite fluoride at low temperature through the combination of the hydrothermal method and the mechanochemical method. The obtained high-entropy perovskite fluoride possesses highly dispersed active sites, low charge transfer resistance, and high mass transfer rate, demonstrating excellent OER performance (Fig. 7B and C). Another study focused on the pyrolysis of low eutectic solvents to prepare high-entropy metal phosphides, which was simple, controllable, and the emission of phosphine gas was prevented. Electrochemical performance tests have shown that the HER and OER activities of high-entropy metal phosphides are better than those of single-metal phosphides, and they also exhibit excellent performance in fully hydrolyzing water.
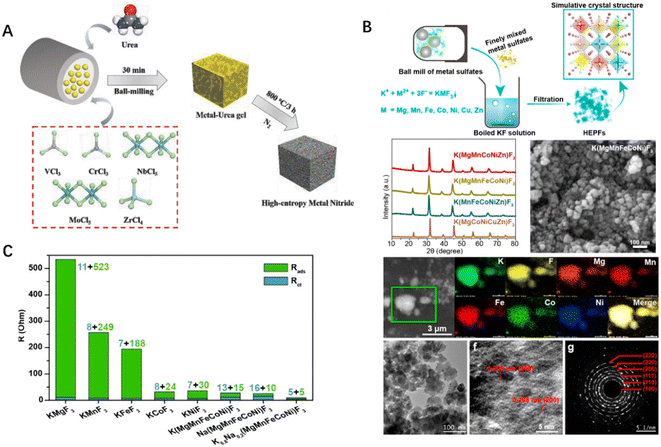 |
| Fig. 7 (A) Schematic diagram to illustrate the preparation of high-entropy nitride by incorporating soft urea and mechanochemistry. (B) Synthesis and characterization of high-entropy perovskite fluorides. (C) Comparison of electrochemical resistance between single component perovskite fluoride and high-entropy perovskite fluoride. Reproduced with permission from John Wiley and Sons82 and reproduced with permission from the American Chemical Society.20 | |
7. Prospects
Although significant progress has been achieved in the research on HECs, further efforts from various aspects are necessary to advance their development. The immiscibility issue arising from the complexity and compositional differences in the elements in HECs necessitates the reliance on non-equilibrium methods, such as temperature and pressure based methods, to achieve uniform mixing and nano-size structures. The ability to synthesize HECs at low temperatures remains limited, as only elements with similar properties can form a single-phase solid solution structure. In addition, there is a need to further explore the techniques for controlling the size and morphology of HECs. Shape-controlled synthesis of HEC catalysts, especially those with high-index crystal planes, presents significant challenges, which hinder the further enhancement of their catalytic performance. In terms of catalytic applications, most of the reported HECs are employed in thermal catalysis and electrocatalysis, while the prospects of HECs in photocatalysis require further exploration. However, there is still a need to solve the problem of designing HECs accurately to suit specific catalytic reactions. Additionally, the relationship between various active sites in HECs and reaction intermediates remains unclear, making it challenging to identify the catalytically active sites accurately and understand the reaction mechanisms. Accurate localization of the targeted metal atoms in HECs is the key to fully understand the structure–activity–selectivity relationship of HECs. For monometallic and bimetallic catalytic systems, the identification of metal active centers may be relatively easy, but with the increasing number of component elements, it will become much more complicated for the accurate understanding of the whole catalytic reaction system mechanism. The selection of a suitable complexing agent to mask a target metal atom is usually considered, but there are many interferents in the actual complex catalytic reaction system. For example, the addition of a complexing agent will bring unknown effects to the catalytic reaction of HECs and the complexing agent will usually complex several metal atoms at the same time, which will lead to inaccurate results, and so on. For catalytic reactions, there is a trend to use calculation methods, such as density functional theory calculations and surface science methods that can help to gain a deeper understanding of the reaction mechanism. For HEC catalytic materials, there is a great variety of surface atom arrangements and combinations, and the computational selection of theoretically corrected models may yield twice the results with half the effort.55 There has been a lot of relevant literature on HECs, but there are no detailed guidelines, e.g., identification of reactive sites, grasp of fundamentals, etc.84–86 Although research on traditional catalysts may provide some answers, the development of high-throughput methods and data mining holds great potential for advancing the research on HECs.
Conflicts of interest
There are no conflicts to declare.
Acknowledgements
This work is supported by the National Natural Science Foundation of China (no. 51872147 and 22136003), the Hubei Provincial Natural Science Foundation of China (no. 2022CFA065), and the 111 Project (D20015). X. Y. K. acknowledges that this research is supported by the Singapore National Academy of Science (SNAS) and the National Research Foundation (NRF) Singapore under SNAS ASEAN Postdoctoral Fellowship (NRF-MP-2020-0001).
References
- C. M. Rost,
et al., Entropy-stabilized oxides, Nat. Commun., 2015, 6, 8485 CrossRef CAS PubMed.
- J. W. Yeh,
et al., Nanostructured high-entropy alloys with multiple principal elements: novel alloy design concepts and outcomes, Adv. Eng. Mater., 2004, 6, 299–303 CrossRef CAS.
- Y. Ma,
et al., High-entropy energy materials: challenges and new opportunities, Energy Environ. Sci., 2021, 14, 2883–2905 RSC.
- C. Oses, C. Toher and S. Curtarolo, High-entropy ceramics, Nat. Rev. Mater., 2020, 5, 295–309 CrossRef CAS.
- Y. Wang, J. Mi and Z.-S. Wu, Recent status and challenging perspective of high-entropy oxides for chemical catalysis, Chem Catal., 2022, 2, 1624–1656 CrossRef CAS.
- Y. Sun and S. Dai, High-entropy materials for catalysis: A new frontier, Sci. Adv., 2021, 7, eabg1600 CrossRef CAS.
- Y. Xin,
et al., High-entropy alloys as a platform for catalysis: progress, challenges, and opportunities, ACS Catal., 2020, 10, 11280–11306 CrossRef CAS.
- D. Wu,
et al., Noble-metal high-entropy-alloy nanoparticles: atomic-level insight into the electronic structure, J. Am. Chem. Soc., 2022, 144, 3365–3369 CrossRef CAS.
- Y.-J. Deng, V. Tripkovic, J. Rossmeisl and M. Arenz, Oxygen reduction reaction on Pt overlayers deposited onto a gold film: ligand, strain, and ensemble effect, ACS Catal., 2016, 6, 671–676 CrossRef CAS.
- Y. Yao,
et al., Computationally aided, entropy-driven synthesis of highly efficient and durable multi-elemental alloy catalysts, Sci. Adv., 2020, 6, eaaz0510 CrossRef CAS PubMed.
- P.-C. Chen,
et al., Polyelemental nanoparticle libraries, Science, 2016, 352, 1565–1569 CrossRef CAS PubMed.
- Y. Yao,
et al., Carbothermal shock synthesis of high-entropy-alloy nanoparticles, Science, 2018, 359, 1489–1494 CrossRef CAS PubMed.
- A. Sarkar,
et al., High-entropy oxides for reversible energy storage, Nat. Commun., 2018, 9, 3400 CrossRef PubMed.
- C. Zhao, F. Ding, Y. Lu, L. Chen and Y. S. Hu, High-entropy layered oxide cathodes for sodium-ion batteries, Angew. Chem., Int. Ed., 2020, 59, 264–269 CrossRef CAS.
- Y. Xu,
et al., Peroxides-Mediated Selective Conversion of Biomass Polysaccharides over High-entropy Sulfides via Solar Energy Catalysis, Energy Environ. Sci., 2023, 16, 1531–1539 RSC.
- C. R. McCormick and R. E. Schaak, Simultaneous multication exchange pathway to high-entropy metal sulfide nanoparticles, J. Am. Chem. Soc., 2021, 143, 1017–1023 CrossRef CAS.
- Z. Deng,
et al., Semiconducting high-entropy chalcogenide alloys with ambi-ionic entropy stabilization and ambipolar doping, Chem. Mater., 2020, 32, 6070–6077 CrossRef CAS.
- S. Niu,
et al., Electrical Discharge Induced Bulk-to-Nanoparticle Transformation: Nano High-Entropy Carbide as Catalysts for Hydrogen Evolution Reaction, Adv. Funct. Mater., 2022, 32, 2203787 CrossRef CAS.
- J. Gild,
et al., High-entropy metal diborides: a new class of high-entropy materials and a new type of ultrahigh temperature ceramics, Sci. Rep., 2016, 6, 1–10 CrossRef PubMed.
- T. Wang, H. Chen, Z. Yang, J. Liang and S. Dai, High-entropy perovskite fluorides: A new platform for oxygen evolution catalysis, J. Am. Chem. Soc., 2020, 142, 4550–4554 CrossRef CAS PubMed.
- D. Wu,
et al., Platinum-group-metal high-entropy-alloy nanoparticles, J. Am. Chem. Soc., 2020, 142, 13833–13838 CrossRef CAS PubMed.
- Z. Jin,
et al., Nanoporous Al-Ni-Co-Ir-Mo high-entropy alloy for record-high water splitting activity in acidic environments, Small, 2019, 15, 1904180 CrossRef CAS PubMed.
- T. Löffler,
et al., Toward a paradigm shift in electrocatalysis using complex solid solution nanoparticles, ACS Energy Lett., 2019, 4, 1206–1214 CrossRef.
- T. Löffler,
et al., Discovery of a multinary noble metal–free oxygen reduction catalyst, Adv. Energy Mater., 2018, 8, 1802269 CrossRef.
- C. Zhan,
et al., Medium/High-Entropy Amalgamated Core/Shell Nanoplate Achieves Efficient Formic Acid Catalysis for Direct Formic Acid Fuel Cell, Angew. Chem., Int. Ed., 2023, 62, e202213783 CrossRef CAS.
- J. Liu,
et al., Polyoxometalate Cluster-Incorporated High-entropy Oxide Sub-1 nm Nanowires, J. Am. Chem. Soc., 2022, 144, 23191–23197 CrossRef CAS PubMed.
- Y. Sun,
et al., A general approach to high-entropy metallic nanowire electrocatalysts, Matter, 2023, 6, 193–205 CrossRef CAS.
- S.-k Wu, Y. Pan, N. Wang, T. Lu and W.-j Dai, Azo dye degradation behavior of AlFeMnTiM (M= Cr, Co, Ni) high-entropy alloys, Int. J. Miner., Metall. Mater., 2019, 26, 124–132 CrossRef CAS.
- Z. Lv,
et al., Development of a novel high-entropy alloy with eminent efficiency of degrading azo dye solutions, Sci. Rep., 2016, 6, 34213 CrossRef CAS PubMed.
- J. Hao,
et al., Unraveling the electronegativity-dominated intermediate adsorption on high-entropy alloy electrocatalysts, Nat. Commun., 2022, 13, 2662 CrossRef CAS.
- T. Li,
et al., Denary oxide nanoparticles as highly stable catalysts for methane combustion, Nat. Catal., 2021, 4, 62–70 CrossRef CAS.
- A. Khorshidi, J. Violet, J. Hashemi and A. A. Peterson, How strain can break the scaling relations of catalysis, Nat. Catal., 2018, 1, 263–268 CrossRef.
- K.-Y. Tsai, M.-H. Tsai and J.-W. Yeh, Sluggish diffusion in co–cr–fe–mn–ni high-entropy alloys, Acta Mater., 2013, 61, 4887–4897 CrossRef CAS.
- H. Luo,
et al., A strong and ductile medium-entropy alloy resists hydrogen embrittlement and corrosion, Nat. Commun., 2020, 11, 3081 CrossRef CAS.
- H. Chen,
et al., Construction of a nanoporous highly crystalline hexagonal boron nitride from an amorphous precursor for catalytic dehydrogenation, Angew. Chem., 2019, 131, 10736–10740 CrossRef.
- L. Huang,
et al., Catalyst design by scanning probe block copolymer lithography, Proc. Natl. Acad. Sci. U. S. A., 2018, 115, 3764–3769 CrossRef CAS.
- D. Wang,
et al., Low-temperature synthesis of small-sized high-entropy oxides for water oxidation, J. Mater. Chem. A, 2019, 7, 24211–24216 RSC.
- Y. Jien-Wei, Recent progress in high-entropy alloys, Ann. Chim. Sci. Mater., 2006, 31, 633–648 CrossRef.
- W.-T. Koo, J. E. Millstone, P. S. Weiss and I.-D. Kim, The design and science of polyelemental nanoparticles, ACS Nano, 2020, 14, 6407–6413 CrossRef CAS.
- M. Cui,
et al., High-entropy metal sulfide nanoparticles promise high-performance oxygen evolution reaction, Adv. Energy Mater., 2021, 11, 2002887 CrossRef CAS.
- H. Wu,
et al., Rapid Joule-Heating Synthesis for Manufacturing High-Entropy Oxides as Efficient Electrocatalysts, Nano Lett., 2022, 22, 6492–6500 CrossRef CAS PubMed.
- B. Wang,
et al., General synthesis of high-entropy alloy and ceramic nanoparticles in nanoseconds, Nat. Syn., 2022, 1, 138–146 CrossRef.
- S. L. James,
et al., Mechanochemistry: opportunities for new and cleaner synthesis, Chem. Soc. Rev., 2012, 41, 413–447 RSC.
- N. L. Broge, M. Bondesgaard, F. Søndergaard-Pedersen, M. Roelsgaard and B. B. Iversen, Autocatalytic Formation of High-Entropy Alloy Nanoparticles, Angew. Chem., 2020, 132, 22104–22108 CrossRef.
- S. Gao,
et al., Synthesis of high-entropy alloy nanoparticles on supports by the fast moving bed pyrolysis, Nat. Commun., 2020, 11, 2016 CrossRef CAS.
- L. De Trizio and L. Manna, Forging colloidal nanostructures via cation exchange reactions, Chem. Rev., 2016, 116, 10852–10887 CrossRef CAS.
- M. Liberato, Forging Colloidal Nanostructures via Cation Exchange Reactions, Chem. Rev., 2016, 116, 10852–10887 CrossRef.
- H. Li,
et al., Sequential cation exchange in nanocrystals: preservation of crystal phase and formation of metastable phases, Nano Lett., 2011, 11, 4964–4970 CrossRef CAS PubMed.
- H. Li,
et al., Synthesis of uniform disk-shaped copper telluride nanocrystals and cation exchange to cadmium telluride quantum disks with stable red emission, J. Am. Chem. Soc., 2013, 135, 12270–12278 CrossRef CAS PubMed.
- L. De Trizio,
et al., Cu3-x P nanocrystals as a material platform for near-infrared plasmonics and cation exchange reactions, Chem. Mater., 2015, 27, 1120–1128 CrossRef CAS.
- W. Van der Stam,
et al., Highly emissive divalent-ion-doped colloidal CsPb1–x M x Br3 perovskite nanocrystals through cation exchange, J. Am. Chem. Soc., 2017, 139, 4087–4097 CrossRef CAS PubMed.
- P. Xie,
et al., Highly efficient decomposition of ammonia using high-entropy alloy catalysts, Nat. Commun., 2019, 10, 4011 CrossRef PubMed.
- Y. Yao,
et al., High-throughput, combinatorial synthesis of multimetallic nanoclusters, Proc. Natl. Acad. Sci., 2020, 117, 6316–6322 CrossRef CAS PubMed.
- C. J. Jacobsen,
et al., Catalyst design by interpolation in the periodic table: bimetallic ammonia synthesis catalysts, J. Am. Chem. Soc., 2001, 123, 8404–8405 CrossRef CAS.
- Z. W. Seh,
et al., Combining theory and experiment in electrocatalysis: Insights into materials design, Science, 2017, 355, eaad4998 CrossRef.
- D. Wu,
et al., On the electronic structure and hydrogen evolution reaction activity of platinum group metal-based high-entropy-alloy nanoparticles, Chem. Sci., 2020, 11, 12731–12736 RSC.
- G. Zhang,
et al., High-entropy alloy as a highly active and stable electrocatalyst for hydrogen evolution reaction, Electrochim. Acta, 2018, 279, 19–23 CrossRef CAS.
- Y. He,
et al., Self-Circulating Adsorption–Desorption Structure of Non-Noble High-Entropy Alloy Electrocatalyst Facilitates Efficient Water Splitting, ACS Sustainable Chem. Eng., 2023, 11, 5055–5064 CrossRef CAS.
- S. Jin, Are Metal Chalcogenides, Nitrides, and Phosphides Oxygen Evolution Catalysts or Bifunctional Catalysts?, ACS Energy Lett., 2017, 2, 1937–1938 CrossRef CAS.
- B. Song,
et al., In situ oxidation studies of high-entropy alloy nanoparticles, ACS Nano, 2020, 14, 15131–15143 CrossRef CAS PubMed.
- H. Zhu,
et al., High-entropy alloy stabilized active Ir for highly efficient acidic oxygen evolution, Chem. Eng. J., 2022, 431, 133251 CrossRef CAS.
- H. Zhu,
et al., High-entropy atomic environment converts inactive to active site for electrocatalysis, Energy Environ. Sci., 2023, 16, 619–628 RSC.
- T. A. Batchelor,
et al., High-entropy alloys as a discovery platform for electrocatalysis, Joule, 2019, 3, 834–845 CrossRef CAS.
- M. W. Glasscott,
et al., Electrosynthesis of high-entropy metallic glass nanoparticles for designer, multi-functional electrocatalysis, Nat. Commun., 2019, 10, 2650 CrossRef PubMed.
- Z. Chen, J. Wen, C. Wang and X. Kang, Convex Cube-Shaped Pt34Fe5Ni20Cu31Mo9Ru High-entropy Alloy Catalysts toward High-Performance Multifunctional Electrocatalysis, Small, 2022, 18, 2204255 CrossRef CAS PubMed.
- F. Tavani,
et al., Structural and mechanistic insights into low-temperature CO oxidation over a prototypical high-entropy oxide by Cu L-edge operando soft X-ray absorption spectroscopy, Phys. Chem. Chem. Phys., 2021, 23, 26575–26584 RSC.
- M. Fracchia,
et al., Stabilization by configurational entropy of the Cu (II) active site during CO oxidation on Mg0.2Co0.2Ni0.2Cu0.2Zn0.2O, J. Phys. Chem. Lett., 2020, 11, 3589–3593 CrossRef CAS PubMed.
- Z. Zhang,
et al., Mechanochemical nonhydrolytic sol–gel-strategy for the production of mesoporous multimetallic oxides, Chem. Mater., 2019, 31, 5529–5536 CrossRef CAS.
- C. Deng,
et al., High-entropy oxide stabilized molybdenum oxide via high temperature for deep oxidative desulfurization, Appl. Materi. Today, 2020, 20, 100680 CrossRef.
- T. X. Nguyen, Y. C. Liao, C. C. Lin, Y. H. Su and J. M. Ting, Advanced high-entropy perovskite oxide electrocatalyst for oxygen evolution reaction, Adv. Funct. Mater., 2021, 31, 2101632 CrossRef CAS.
- L. Tang,
et al., Binary-dopant promoted lattice oxygen participation in OER on cobaltate electrocatalyst, Chem. Eng. J., 2021, 417, 129324 CrossRef CAS.
- T. Wang,
et al., Perovskite oxide–halide solid solutions: A platform for electrocatalysts, Angew. Chem., 2021, 133, 10041–10046 CrossRef.
- Z. Meng,
et al., A general strategy for preparing hollow spherical multilayer structures of Oxygen-Rich vacancy transition metal Oxides, especially high-entropy perovskite oxides, Chem. Eng. J., 2023, 457, 141242 CrossRef CAS.
- Z. Jin,
et al., Rugged high-entropy alloy nanowires with in situ formed surface spinel oxide as highly stable electrocatalyst in Zn–air batteries, ACS Mater. Lett., 2020, 2, 1698–1706 CrossRef CAS.
- H. Xu,
et al., Entropy-stabilized single-atom Pd catalysts via high-entropy fluorite oxide supports, Nat. Commun., 2020, 11, 3908 CrossRef CAS PubMed.
- H. Chen,
et al., Entropy-stabilized metal oxide solid solutions as CO oxidation catalysts with high-temperature stability, J. Mater. Chem. A, 2018, 6, 11129–11133 RSC.
- H. Chen,
et al., Mechanochemical synthesis of high-entropy oxide materials under ambient conditions: Dispersion of catalysts via entropy maximization, ACS Mater. Lett., 2019, 1, 83–88 CrossRef CAS.
- S. Mitchell, R. Qin, N. Zheng and J. Pérez-Ramírez, Nanoscale engineering of catalytic materials for sustainable technologies, Nat. Nanotechnol., 2021, 16, 129–139 CrossRef CAS.
- H. Chen,
et al., Self-regenerative noble metal catalysts supported on high-entropy oxides, Chem. Commun., 2020, 56, 15056–15059 RSC.
- Y. Lei,
et al., Carbon-supported high-entropy Co-Zn-Cd-Cu-Mn sulfide nanoarrays promise high-performance overall water splitting, Nano Res., 2022, 15, 6054–6061 CrossRef CAS.
- T. X. Nguyen, Y. H. Su, C. C. Lin and J. M. Ting, Self-reconstruction of sulfate-containing high-entropy sulfide for exceptionally high-performance oxygen evolution reaction electrocatalyst, Adv. Funct. Mater., 2021, 31, 2106229 CrossRef CAS.
- T. Jin,
et al., Mechanochemical-assisted synthesis of high-entropy metal nitride via a soft urea strategy, Adv. Mater., 2018, 30, 1707512 CrossRef.
- X. Zhao, Z. Xue, W. Chen, Y. Wang and T. Mu, Eutectic synthesis of high-entropy metal phosphides for electrocatalytic water splitting, ChemSusChem, 2020, 13, 2038–2042 CrossRef CAS PubMed.
- M. K. Plenge, J. K. Pedersen, V. A. Mints, M. Arenz and J. Rossmeisl, Following Paths of Maximum Catalytic Activity in the Composition Space of High-Entropy Alloys, Adv. Energy Mater., 2023, 13, 2202962 CrossRef CAS.
- C.-L. Huang,
et al., Atomic scale synergistic interactions lead to breakthrough catalysts for electrocatalytic water splitting, Appl. Catal., B, 2023, 320, 122016 CrossRef CAS.
-
Y. Zhang, High-Entropy Materials-A Brief Introduction, Springer, 2019 Search PubMed.
|
This journal is © the Partner Organisations 2024 |