DOI:
10.1039/D3MA00817G
(Review Article)
Mater. Adv., 2024,
5, 986-1016
Bacteriophages as nanocarriers for targeted drug delivery and enhanced therapeutic effects
Received
6th October 2023
, Accepted 23rd December 2023
First published on 1st January 2024
Abstract
The effective delivery of therapeutic agents is as important as the active therapeutic agent because it may make or mar the outcome. Traditional/conventional drug delivery systems (DDSs) face many limitations, including poor bioavailability, poor specificity and targeting, inconsistent drug adsorption, short half-life, rapid drug clearance, instability, varying and suboptimal drug effects, uncomfortable administration, limited drug loading into delivery vehicles, and poor treatment adherence and compliance, opening the way for innovations, including optimising the use of nanocarriers. Nanocarriers encapsulate and deliver drug agents using nanosized vehicles to enhance drug effectiveness, bioavailability as well as specific, targeted, and controlled drug release. Examples of such nanocarriers include micelles, microemulsions, nanoemulsions, lipid nanoparticles, liposomes, niosomes, dendrimers, and exosomes, which are formulated using different vehicles. Virus-like nanoparticles are emerging, with most involving bacteriophages—the environmentally ubiquitous, abundant, and diverse group of nanosized structurally simple viral particles with the intrinsic and inherent ability to invade bacteria. Studies involving different bacteriophages, their nano drug formulation, and their application against some diseases exist; however, no current review aggregates the advances made so far, which could be attributed to the recency of the research areas. Such a review is vital because it highlights and precipitates milestones and can provide necessary basis for further advancements. Thus, this study aims to evaluate the advancing potential use of bacteriophages as nanocarriers for targeted drug delivery and their potential for enhanced therapeutic effects.
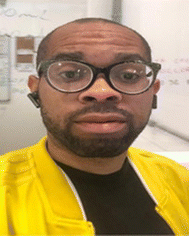
Stephen C. Emencheta
| Stephen Emencheta is a researcher and academic staff member at the University of Nigeria, Nsukka, with a pharmaceutical microbiology and biotechnology background, with research interests encompassing various aspects of bacterial resistance, bacteriophage/phage therapy, bioactive natural products, and bioinformatics, focused on addressing critical issues surrounding the fight against antimicrobial resistance, especially using bacteriophages. He is a recipient of the Nigerian TETFund AST&D scholarship for his PhD benchwork stay at the Viruses of Bacteria Laboratory (VBLab), University of Sorocaba, Brazil. |
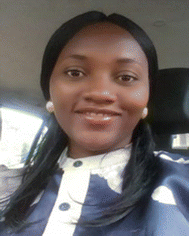
Adaeze L. Onugwu
| Adaeze Onugwu is a lecturer at the Department of Pharmaceutics, University of Nigeria, Nsukka, and a Drug Delivery and Nanomedicines Research Laboratory member. She is a Commonwealth scholar and was a visiting researcher at the University of Reading, United Kingdom. Adaeze completed her PhD in 2023. She has experience in developing lipid and polymer-based nanocarriers for drug delivery. Her research interests include novel drug delivery systems, ocular drug delivery, vaccine delivery, cancer therapy, and phytochemical/herbal medicine. |
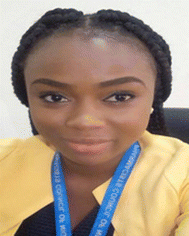
Chisom F. Kalu
| Chisom Flora Kalu is a licensed pharmacist. She has a Bachelor of Pharmacy and Master of Pharmacy degrees, with her latest degree being focused on Pharmaceutical Microbiology and Biotechnology, and she has over five years of experience in community and hospital practice. Beyond her passion for rendering pharmaceutical care, she has a penchant for research, with a research interest in nano-scale drug delivery. She has collaborated with other researchers, publishing research outputs bordering on, but not limited to, her area of interest. |
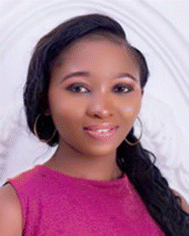
Patience N. Ezinkwo
| Ezinkwo Patience is a Medix Frontier Research team researcher at the College of Medicine, University of Nigeria, Enugu Campus. She obtained her bachelor's degree in Biochemistry/Microbiology from the University of Nigeria, Nsukka, in 2014. She is a 600L student in the Medicine and Surgery Department of the same university. |
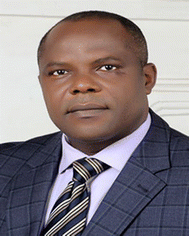
Anthony A. Attama
| Anthony A. Attama is a Professor of Pharmaceutics at the University of Nigeria, Nsukka, where he is the Director of the Institute for Drug-Herbal Medicine-Excipient Research and Development and the Founder and Research Lead Drug Delivery and Nanomedicines Research Laboratory. He was an Alexander von Humboldt postdoctoral researcher at Technische Universitat Braunschweig, Germany, and a Visiting Professor at the Hebrew University of Jerusalem, Israel. He has extensively researched nano drug delivery systems for oral, parenteral, dermal/transdermal, and ocular applications for treating infectious and non-infectious diseases. He is a fellow of the Nigerian Academy of Science. |
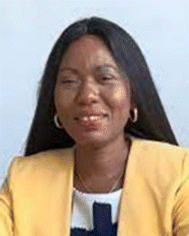
Ebele B. Onuigbo
| Prof. Ebele Onuigbo is a lecturer in the Department of Pharmaceutical Microbiology and Biotechnology, University of Nigeria, Nsukka. She has carried out extensive research on drug delivery, especially vaccine delivery. She has also made contributions to the field of bacteriophages. She was a visiting researcher at the University of Padova, Italy, and the University of Nottingham, United Kingdom. She won the best Merck Foundation female African Researcher award in 2021. |
1. Introduction
Therapeutic agents for medical interventions are delivered to the body to achieve desired pharmacological effects using different systems that enhance therapeutic output and are referred to as drug delivery systems (DDSs).1 They are primarily aimed at improving therapeutic efficacy by providing the optimal or sustained release of bioactive agents; reducing administration frequency; ensuring continuous drug supply at therapeutic levels; achieving the targeted/specific delivery of drugs to the site of action; enhancing drug localisation; reducing off-target effects, minimising side effects; enhancing safety via the minimisation of systemic exposure; protecting drugs against enzymatic degradation, pH and other environmental conditions; improving drug stability and bioavailability; and increasing patient and treatment compliance and adherence.2–6
Traditional/conventional DDSs include the most common and convenient ones: oral DDSs, involving the use of capsules, tablets, suspensions, and syrups adsorbed via the gastrointestinal tract (GIT) into the bloodstream;7 parenteral DDSs, involving the intramuscular, intravenous, or subcutaneous liquid drug passage, bypassing the GIT and rapidly reaching the bloodstream for fast pharmacological activities;8 transdermal/topical DDSs, involving the direct topical application of therapeutic agents, including creams, lotions, ointments, gels, and patches on the skin barrier to reach the bloodstream;9 pulmonary DDSs, involving the localised and rapid administration of therapeutic inhalable particles, such as powders, aerosols, and nebulisers into the lungs;10 nasal DDSs, involving the administration of nasal drops and sprays through the nasal cavity into the bloodstream;11 and recta and vaginal DDSs, involving the respective localised administration of drugs via the rectal and vaginal routes.12 Although these DDSs have been studied and applied extensively, they face many challenges bordering on their efficacies, safety profiles, and patient compliance. Some of these exhibit poor bioavailability owing to factors such as low solubility, liver-related first-pass metabolism, poor permeability, and poor specificity and targeting, leading to increased potential side effects and toxicities, inconsistent drug adsorption owing to varying systemic conditions, including food interactions, pH and intestinal motilities, short half-life leading to frequent drug administration, impacting patient drug regime compliance, rapid drug clearance as seen with hepatic or renal pathways, physical and chemical instabilities while responding to environmental conditions, varying drug effects owing to variations in genetics, metabolism, and physiological states, suboptimal drug effects due to non-uniform drug distribution, uncomfortable administration routes as seen with parenteral DDSs, which also increase the chances of infections, limited drug loading into delivery vehicles, and as already mentioned, poor treatment adherence and compliance.13–17 These challenges leave room for interventions and innovations, including using nano drug carriers for more efficient drug delivery and enhanced therapeutic outcomes.
Nanocarriers are nanosized (size < 500 nm) colloidal systems whose emergence has revolutionised drug delivery technologies and encapsulate and deliver drug agents using nanosized vehicles to enhance drug effectiveness, bioavailability, and specific, targeted, and controlled drug release.18 Micelles, micro- and nanoemulsions, lipid nanoparticles, liposomes, niosomes, dendrimers, and exosomes are the most common nanocarriers and have been developed and delivered using diverse, relevant materials and particles, including natural dwelling life forms.18 One of the emerging advances in nanocarrier technologies is virus-like nanoparticles, including bacteriophages.19
The environmentally ubiquitous, abundant, and diverse group of nanosized structurally simple viral particles with the intrinsic and inherent ability to invade bacteria, making them serve as biological hosts for their propagation through various mechanisms, including the manipulation of the host bacterial replication, the use of the same, and the release of specific associated proteins, such as endolysins and holins, are known as bacteriophages and are abridgedly termed phages.20–22 Phages have distinguishing and associated characteristics, including their small size and ability to invade and propagate with only one or limited but usually related species of bacterial hosts.21,23 This property is one of the essential features of categorising bacteriophages into different host range profiles and has been exploited for research and medicinal purposes.24,25 Bacteriophages, with polyvalent ligands, display scaffolds,26 and have found many applications, including their use in the therapeutic management of disease in different delivery systems and routes of administration, environmental monitoring of pathogenic bacteria for specific purposes, and their use as the basis for the delivery of bioactive agents for specific therapeutic benefits.24,27,28 Alongside the efforts to utilise phages as active therapeutic agents and alternatives to antibiotics in managing diseases, there are also advancing studies in their use as nanocarriers of therapeutic agents in different applications owing to their already-mentioned desirable properties. Different studies involving different bacteriophages, their nano drug formulation, and their application against some diseases exist; however, limited current reviews are aggregating the advances and could be attributed to the recency of the research areas. Such a review is vital because it highlights and precipitates the milestones and can provide the necessary basis for further advancement of the research area, including comparing research methodologies and findings. Therefore, the study aims to evaluate the potential use of bacteriophages as nanocarriers for targeted drug delivery and the potential for enhanced therapeutic effects. Consequently, we described the nature and characteristics of bacteriophages, the basic concepts of nanocarriers in drug delivery, the application of bacteriophages as nanocarriers for drug delivery, possible enhancement effects of bacteriophage-mediated drug delivery, specific application of bacteriophage-mediated drug delivery, and finally the challenges and future directives.
2. Drug delivery systems and nanocarriers
This section examines conventional drug delivery systems, their drawbacks, and novel drug delivery approaches. We also try to describe different nanocarriers and their benefits and limitations as drug delivery systems.
2.1 Overview of drug delivery systems
Drug delivery systems refer to approaches, technologies, and formulations for transporting bioactive compounds in the body safely and efficiently.18 Conventional drug delivery systems include solutions, mixtures, suspensions, tablets, capsules, powders, suppositories, paste, ointment, and aerosol. They face the problems of the uncontrolled and wide distribution of drugs in the body, leading to a high frequency of administration and systemic side effects.29 Consequently, conventional drug delivery systems are associated with low efficacy, increasing toxicity, poor patient compliance, and failure in therapeutic outcomes. Over the past few decades, researchers have developed different delivery systems with the potential for controlled and sustained drug release and the ability to target cargo to specific tissues or cells. A novel delivery system can significantly improve drug pharmacokinetics, efficacy, and safety. An old drug can be reformulated and given a new life via a novel delivery system.
Novel drug delivery systems include nanoparticles, niosomes, nanoemulsions, dendrimers, liposomes, exosomes, and micelles. Researchers have employed several strategies to improve the controlled release and site-specificity of delivery systems. These methods include stimuli-responsive and ligand-modified target drug delivery strategies. In stimuli-responsive drug delivery systems, the release of the cargo is triggered by external or internal stimuli, including pH, temperature, enzymes, and a light magnetic field. Using enzymes, Radhakrishnan et al.30 facilitated the delivery of an anticancer agent into cancer cells by the dual trypsin or hyaluronidase degradation of nanocapsules bearing anticancer agents. Ligands, such as folic acid, sugars, hyaluronic acid, and aptamers, interact with receptors and biomarkers found in target tissues; hence, they can be applied in targeted drug delivery systems.31 Red blood cell membrane-coated nanocrystals were modified with tumour-targeting peptide c(RGDyK) by Chai et al.32 and resultantly presented a superior tumour accumulation and therapeutic efficacy in subcutaneous tumour and orthotropic glioma-induced mice. These strategies ensure that the drugs are released only at the target sites, thereby reducing off-target side effects and increasing drug accumulation at the target site.
2.2 Nanocarriers in drug delivery
Nanomedicine is a new but rapidly growing field in science in which nanosized materials are employed in diagnosing and treating diseases. Nanotechnology-based drug delivery systems (nanocarriers) are colloidal drug carriers with sizes typically less than 500 nm.33 They have been applied in the controlled and targeted delivery of drugs, phytoconstituents, chemotherapeutic agents, genes, and imaging agents to specific body parts. Nanocarriers, such as nanoemulsion, liposomes, and micelles, have been used to treat various diseases, including infections and malignancies, while some are still being investigated in preclinical and clinical studies.4,31,34 Nanocarriers have been reported to offer many advantages over traditional delivery systems. Their unique nanoscale size range, morphology, and biological properties help to improve the solubility, absorption, in vivo stability, site-specific delivery, release, toxicity, and cellular uptake of the drug.35,36 Their compositions, shapes, sizes, and surfaces can be easily modified to achieve the desired therapeutic effects.37 Surface modification by coating with polymeric materials or attachment of ligands or functional groups enhances their pharmacokinetic and biodistribution profiles. Hence, PEGlation, coating, and surface functionalisation are common with nanocarriers.
The description of common nanocarriers, their advantages, and their limitations as drug delivery systems are presented in Table 1. Some common nanocarriers employed in drug delivery are briefly explained below.
Table 1 Novel drug delivery systems with their advantages and limitations
Drug delivery system |
Description |
Advantages |
Limitations |
Nanomicelles |
Self-assembled system of amphiphilic surfactants or block polymeric materials |
Improved drug solubility |
Toxicity |
Increased drug stability |
Poor drug loading |
Suitable for drug targeting |
Lack of scalability. |
Microemulsions |
Colloidal dispersion of oil and water stabilised by surfactant |
Improved solubility |
Toxicity due to the presence of surfactant |
Nanoemulsion |
Ease of formulation |
Low stability |
|
Ability to load both hydrophilic and hydrophobic drugs |
|
Inexpensiveness |
|
Long shelf life |
Lipid nanoparticles |
A colloidal system with a lipid core and a surfactant shell |
Solubilise poorly water-soluble drugs |
Challenging to achieve uniform particle size distribution |
Use of safe and biocompatible lipids |
Expulsion of the drug upon storage |
Provide controlled drug release |
Poor drug loading capacity |
Provide targeted delivery |
Cytotoxic effects associated with the components |
Large scalability potential |
Liposomes |
Vesicular drug delivery systems with an aqueous core and one or more phospholipid bilayers |
Capacity to load both hydrophilic and lipophilic drugs |
Low stability |
Biocompatible and low-toxicity |
Low payload |
High membrane permeability |
Lack of appropriate sterilisation method |
Leaching of encapsulated drug |
Expensive production cost |
Niosomes |
A vesicular system with amphiphilic non-ionic surfactant bilayer |
Less toxic |
Low drug loading |
Biodegradable |
Drug expulsion |
Biocompatible |
Instability and aggregation of vesicles |
High production costs |
Dendrimers |
Star-shaped hyperbranched structure with numerous surface function groups |
Small size |
Low aqueous solubility |
Improved drug solubility |
Nonspecific toxicity |
Capacity to deliver both hydrophilic and hydrophobic drugs |
Low scalability potential |
High loading capacity |
Protection of medicine from premature degradation |
High functionalisation capability |
High drug targeting potential |
Exosome |
Extravascular vesicle |
Biodegradability |
Instability |
Inherent bioactivity |
Low yield |
High tolerability |
Low scalability potential |
High drug loading |
Lack of quality control standards |
High membrane permeability |
High tissue targeting and cellar uptake |
Virus-like nanoparticles |
Self-assembled nanosized viral capsid-based systems with uniform geometry |
Biocompatibility |
Low yield |
High surface functionalisation capability |
High production cost |
Targeted delivery of their cargo |
High encapsulation efficiency |
2.2.1 Micelles.
Micelles are drug delivery systems formed spontaneously in a polymeric or surfactant solution when the concentration of the polymer/surfactant is above the critical micellar concentration.38 They can be grouped into normal, reversed, or polymer micelles (Fig. 1). Normal micelles have a hydrophilic moiety as the core and serve as carriers for hydrophobic drugs. The reversed micelles consist of clusters of hydrophobic moieties as the core and are utilised to deliver hydrophilic medicines.39 Normal and reversed micelles are formed from surfactants, and polymer micelles are formulated using amphiphilic di- or tri-block polymers. Micelles increase the solubility and stability of drugs and sustain their release in vivo.40,41 Some challenges in using micelles as nanocarriers are toxicity, poor drug loading, and lack of scalability.
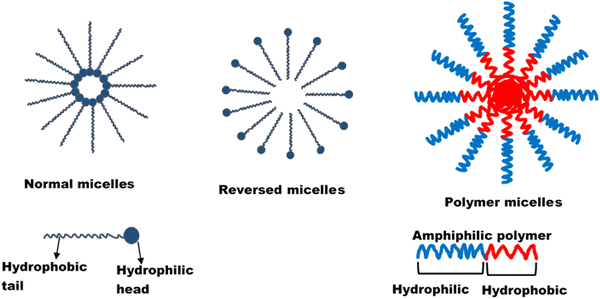 |
| Fig. 1 Structure of micelle. Reprinted from Onugwu et al., 2023 with permission from Elsevier.34 | |
2.2.2 Microemulsions and nanoemulsions.
Microemulsions and nanoemulsions are colloidal dispersions of oil-in-water or water-in-oil. They are stabilised by a surfactant and a cosurfactant.42 Microemulsion and nanoemulsion have similar components but differ in stability. Although the former is thermodynamically stable, the latter is not thermodynamically stable and exhibits only kinetic stability. The increasing interest in these systems is due to their easy formulation process, ability to be loaded with hydrophilic and hydrophobic drugs, and inexpensive and long shelf life.43 However, their instability and toxicity due to high surfactant concentrations hinder drug delivery use.44
2.2.3 Nanoparticles.
Nanoparticles comprise mainly lipid and polymer nanoparticles. However, inorganic nanoparticles, such as gold, silver, mesoporous silica, and iron oxide nanoparticles, are also employed as drug delivery systems. Lipid nanoparticles are colloidal drug delivery systems with a lipid core surrounded by a surfactant shell. Solid lipid nanoparticle is a first-generation lipid nanoparticle similar to nanoemulsion, but the liquid oil used in nanoemulsion is replaced with solid lipid to improve the instability issue of nanoemulsion. Owing to their beneficial properties, SLNs are superior to nanoemulsions, liposomes, and polymeric nanoparticles.45 The lipid core is suitable for solubilising poorly water-soluble drugs in aqueous dispersion. The use of generally regarded as safe and biocompatible lipids in the formulation of SLN improves the safety profile of the system. The solid-state of the lipid core at body temperature increases the stability of this system. They can be easily modified to control drug release and targeting. The drawbacks of using this system are low drug loading, difficulty in obtaining uniform particle dispersion, toxicity, and drug expulsion during storage.46 Recent advancements in this system, such as nanostructured lipid carriers, lipid–drug conjugates, and hybrid lipid polymer nanoparticles, are strategies to overcome the limitations of solid lipid nanoparticles.
Nanostructured lipid carriers (NLCs) are an effective alternative to solid lipid nanoparticles. They comprise a lipid core comprising both liquid and solid lipids (Fig. 2). The presence of both lipids leads to a less perfect crystalline structure with more defects/spaces for drug loading.47 Polymeric nanoparticles are nanosized colloidal systems of polymeric materials with drugs entrapped in the core (nanocapsule) or the polymer matrix (nanosphere). Biocompatible and biodegradable natural or synthetic polymers are employed to formulate polymer nanoparticles. Polymeric nanoparticles have higher storage stability, higher circulation half-life in the biological system, and a better-controlled release profile.48 The lipid-polymer hybrid nanoparticle is an emerging type of lipid nanoparticle, a blend of polymer and lipid nanoparticles. The polymeric core is surrounded by a phospholipid layer coated with a lipid-PEG shell49 (Fig. 2). A system's mixture of lipid and polymer results in higher mechanical strength, biocompatibility, high payload for both hydrophilic and lipophilic drugs and controlled drug release.50
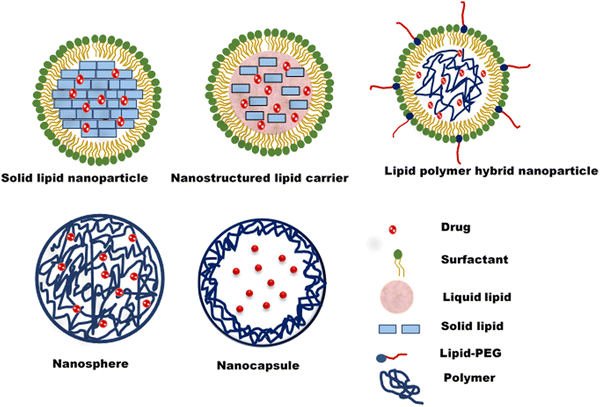 |
| Fig. 2 Different types of lipid nanoparticles. | |
2.2.4 Liposomes.
Liposomes are lipid vesicular drug delivery systems with an aqueous core and one or more phospholipid bilayers. Based on the number and size of the bilayer, liposomes can be classified into small or large unilamellar or multilamellar liposomes.51 Liposomal drug delivery systems show biocompatibility and biodegradability properties. They are biocompatible owing to their structural similarity with cell membranes. Both hydrophilic and hydrophobic compounds can be encapsulated in this carrier. Positive charge liposomes interact with the cell membrane through electrostatic interaction. Their short shelf life, low drug payload, drug leakage during storage, and sterilisation issues limit their use in drug delivery.52
2.2.5 Niosomes.
Niosomes are another vesicular delivery system and are structurally similar to liposomes. However, their bilayer is composed of amphiphilic non-ionic surfactants.53 They are biocompatible, non-immunogenic, and biodegradable and exhibit low toxicity due to non-ionic surfactants. They show more chemical stability and longer shelf life than liposomes.54 Some limitations of niosomes are low drug loading, drug expulsion, instability, and high production cost.44
2.2.6 Dendrimers.
Dendrimers consist of a polymeric star-shaped structure of a core, hyperbranched, and numerous surface function groups.55 They exhibit unique architectural designs with various shapes, sizes, branchings, and surface functional groups. Therapeutic agents can be entrapped in the dendrimer core or conjugated on the surface.56 Different generations of dendrimers (G1, G2, G3, G4, and G5) represent different levels of branches added to the initiator core during the formulation process. Their advantages as drug delivery systems include small size, functionalisation capability, drug targeting, and ease of preparation.57
2.2.7 Exosomes.
Exosomes are bio-inspired vesicular nanocarriers derived from the fusion of multivesicular bodies with the plasma membrane and secreted upon stimulation.58 They are extracellular vesicles with a hydrophilic core surrounded by a phospholipid bilayer. The host automatically generates exosomes without eliciting immunological reactions. They are superior delivery systems compared to liposomes and other nanocarriers. They offer the advantages of biodegradability, inherent bioactivity, high tolerability, drug loading, membrane permeability, tissue targeting, and cellar uptake.59 They have been investigated for the targeted delivery of small molecules, proteins, and genetic materials. Their major setbacks are instability, low yield, scalability potential, and a lack of quality control standards.60
2.2.8 Virus-like nanoparticle.
Viruses are utilised as carriers in drug delivery. Virus-like nanoparticles are self-assembled nanosized viral capsid-based drug delivery systems with uniform and well-defined morphology. They are made from viral shells without genetic materials. Hence, they are non-infectious. They deliver drugs, genes, vaccines, and imaging agents.61 Plant, animal, and bacterial viruses (bacteriophages) are employed in this system. Some favourable features of virus-like nanoparticles as nanocarriers are uniform geometry, biocompatibility, and high surface functionalisation capability.59 The surface of the particles can be easily modified for the targeted delivery of their cargo. They exhibit high encapsulation efficiency of the loaded cargo.62
Nanocarriers have shown great potential for revolutionising drug and gene delivery. Studies have reported promising results involving different modifications/advancements in nanotechnology-based delivery approaches. However, relatively few nanocarriers have been translated into clinical use because of some hindrances militating against their large-scale manufacture and use. Hence, more studies are needed to introduce more nanocarriers into the market.
3. Bacteriophages
Bacteriophage-inspired nanocarriers have been investigated for the targeted delivery of genes and other therapeutic agents. In this section, we consider the structure and classification of bacteriophages as well as their surface engineering as nanocarriers.
3.1 Structure and classification
Bacteriophages, coined by D'Hérelle from “bacteria” and Greek words “
” (phagein), which collectively means “bacteria eater”63 and are referred to as phages, are viruses that infect and replicate within bacteria. They are the most abundant biological entities on earth and are essential components of various microbiomes, playing a crucial role in regulating bacterial populations in various ecosystems. Structurally, bacteriophages are diverse; however, most of them share some common characteristics, including possessing a polyhedral head (consisting of approximately 2000 capsomeres) enclosing the genetic material, a short collar, and a helical tail. They are categorised into various families, including the syringe-behaving contractile tail possessing Myoviridae, long noncontractile tail possessing Siphoviridae, and short noncontractile tail posing Podoviridae; these three families belong to the Caudovirales class,64Tectiviridae with a protein-rich internal membrane and linear dsDNA genome enclosed within a flexible spike associated icosahedral protein capsid,65Corticoviridae with approximately 10 kbp circular DNA and inner membrane within the icosahedral capsid and associated with limited Pseudoalteromonas species,66,67Lipothrixviridae that are filamentous and enveloped, with a single molecule of linear dsDNA within its helical nucleoprotein core and associated with extreme thermophiles,68Plasmaviridae that are enveloped pleomorphic virions, with approximately 12 kbp circular, supercoiled dsDNA and associated with the Acholeplasma species,69Rudiviridae that are stiff-rod-shaped and unenveloped and possess dsDNA with size in the range of 32.3–35.8 kbp,70Fuselloviridae with short tail fibres, heterogenous size but lemon shaped containing circular, supercoiled polyamines and virus-coded basic protein associated dsDNA, with size in the range of 14.8–17.8 kbp,71Inoviridae with flexible filaments and positive sense circular ssDNA within helical array of many copies of a major capsid protein,72Microviridae associated with enterobacteria are nonenveloped, with ssDNA and T = 1 icosaheral symmetry,73Leviviridae that are spherical with T = 3 icosahedral symmetry and containing a molecule of positive sense ssRNA,74 and Cystoviridae that are enveloped with segmented dsRNA and interior protein capsid polymerase complex associated with transcription, replication, and genome packaging,75 according to morphology and type of nucleic acid,76 as presented in Fig. 3. According to their replication cycle, phages are also classified as either lytic, lysogenic, or temperate.77 Following phage adsorption, penetration, and infection of the host bacteria, the lytic phages, the focus of therapeutic phages, utilise the replication mechanism of the bacteria to propagate its progenies, leading to the death of the bacteria; the lysogenic phages integrate their genome into the host chromosome, with only subtle genetic and physiological effects on the host; the temperate phages, however, can switch between the two life cycles.77
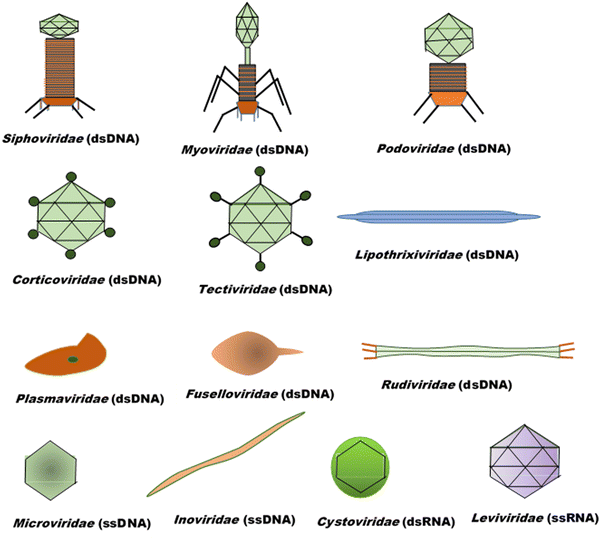 |
| Fig. 3 Basic classes of bacteriophages. | |
3.2 Bacteriophages as nanocarriers for drug delivery
Using self-assembling and easily modifiable bacteriophage capsids as nanocarriers presents potential benefits.78,79 As they can specifically recognise and bind to limited bacterial receptors when engineered to bear the drug payload, including genes, enzymes, nanoparticles, or other therapeutic payloads, phages can potentially deliver these therapeutic agents to bacterial pathogens or disease sites with high precision, thus improving drug specificity and targeting.78 The capsids, usually with large loading capacities, can protect and increase the bioavailability and stability of therapeutic payloads against immune clearance and degradation.80,81 They can contribute to therapeutic actions by the initial lyses of pathogens; subsequently, the direct intracellular delivery of loads, minimising off-target effects and immune responses.82,83 Because they are chiefly biocompatible with human and bacterial cells, they can significantly reduce the chances of toxicity and adverse effects.84–86 Ineffective antibiotics owing to the development of bacterial resistance can be reprioritised by the initial lysis and compromise of the bacterial pathogen, especially when the bacterial resistance mechanism is inherent in the cell wall.87,88 Personalisation and combination therapy can also be enhanced with more efficient therapeutic output.89 Using bacteriophages as nanocarriers in drug delivery often involves surface engineering and the loading of therapeutic agents onto bacteriophages. Loading generally consists of the engineering of phage genetic materials and inserting payloads, surface conjugations, drug capsid encapsulation, electroporation of phage capsids enabling insertion or loading of therapeutic drugs, adsorption via electrostatic or hydrophobic interactions, chemical synthesis during phage assembly, or heat- or pH-induced loading.
3.2.1 Surface engineering of bacteriophages.
Surface engineering is vital to utilising bacteriophages as nanocarriers and involves the manipulation of the outer surface for desired outputs using different mechanisms.90 Peptide display utilises phage capsids to display therapeutic peptides that recognise and bind to specific bacteria surface cell receptors, often involving site-specific mutagenesis N- or C-termini peptide extensions and exterior exposed CP loop insertions.90,91 Antibody display, similar to peptide display, can present antibodies against the cell antigens of specific-infected tissues and cancer cells.28 The chemical conjugation of phages and molecules (e.g., lipids and polymers) facilitates the attachment of drug molecules and improves phage stability, biodistribution, and pharmacokinetics.92 Polymer (e.g., polyethylene glycol) coating of phages reduces immune system recognition and uptake and improves bloodstream circulation.93 In phage capsid manipulations and encapsulation, phages are used as cargo loading for the specific delivery of drug agents within the capsid.94,95 Attaching multi-targeting ligands/moieties onto phage surfaces enables binding to different receptors.96,97 Image tagging of drug payload-carrying bacteriophages enables real-time distribution tracking and targeting efficacy in the body and in disease diagnosis.28 Additionally, phage genome modification involving advanced technologies employs manipulating the genetic material of bacteriophages to enhance the lytic profile.98 Studies on the surface engineering of bacteriophages for drug delivery are described below and summarised in Table 2.
Table 2 Surface engineering of bacteriophages
Type |
Bacteriophage/VLP |
Agent |
Target disease |
Method |
Result |
Ref. |
Peptide display |
Leviviridae PP7 |
C-terminal extensions, dimer loop insertions |
— |
Wild-type PP7 was reconstructed using extensions and insertions by respective C-terminal extensions via PCR flanking with a 3′-sequence base of the primer attached to C′-terminal sequence and 5′-sequence region coding for the extensions and dimer loops insertions (also using similar PCR procedure), after which the construct was expressed and characterised. |
A more tolerable and robust system, displaying a linear and extended looped peptide (exogenous loops between the simultaneous presentation of 2 peptides and capsid monomers) and open room for achieving desirable homogeneous polyvalent particles. |
91
|
Bacteriophage Qβ |
Sialic acid |
Influenza virus infections |
The precise position of sialic acid moieties (ligand) utilising the phage (bacteriophage Qβ) capsid scaffold strategy to achieve the structurally defined positioning of sialic acid matching the binding site of trimeric hemagglutinin of the virus. Following the identification of a capsid residue spaced 5–6 nm on the particle surface, close to the between the sialic acid binding site of hemagglutinin, the expression in the presence of L-homopropargylglycine introduced propargyl group using azide-alkyne cycloaddition and different linkers in anchoring the sialic acid ligands. |
The in vitro result showed enhanced binding affinity of the Qβ capsid to the virus and potent inhibition of the virus, including outperforming oseltamivir (an antiviral agent). The ex vivo and in vivo results significantly reduced viral titre and body weight loss, respectively. |
99
|
Bacteriophage Qβ VLP |
Fluorescent proteins |
— |
The modular system, including specifically engineered RNA–protein interactions and involving chemical derivatisation by acylation and azide-alkyne cycloaddition, encapsulated many copies of fluorescent proteins on Qβ VLP. |
Generated high-affinity carbohydrate-based ligand of the CD22 receptor and particles with similar photochemical and structural properties to monomeric analogue with more stability to thermal and proteolytic degradation. Confocal laser microscopy and flow cytometry were used to detect specific cell labelling by the particles. |
100
|
Bacteriophage P22 VLP |
CD40 L |
Cancer and other immunologically based diseases. |
Multivalent modulation display of CD40 L on the exterior of bacteriophage P22 VLP. |
Indication of higher potency in immunomodulation, following the significant decrease in the half maximal effective concentration (EC50) compared to the control CD40 |
101
|
RNA bacteriophage MS2 |
V3 and ECL2 loop peptides of HIV gp120 and CCR5 coreceptor |
HIV vaccine development |
Engineering and display of V3 and ECL2 loop peptides of HIV gp120 and CCR5 coreceptor, respectively, on RNA bacteriophage MS2. |
Through interrupted protein folding, it was effectively suppressed by the genetic fusion of two identical polypeptides of the VLP coat protein into a single-chain dimer, which supported the display of diverse immunogenic peptides. |
102
|
Antibody display |
Bacteriophage T4 |
Type 3 secretion system low-calcium response V antigen (plague), protective antigen and mutated capsular antigen F1 (anthrax) |
Anthrax and plague |
Engineering of the bacteriophage T4 120- by 86 nm capsids through the multivalent presentation of the Y. pestis 56 kDa-sized type 3 secretion system low-calcium response V antigen and anthrax 83 kDa-sized protective antigen and mutated capsular antigen F1 fused to the 9 kDa-sized Soc protein. |
Potentiation of the in vivo clearance of implicated bacteria through the enhanced elicitation of broad T-helper 1 and 2 immune responses. The resulting vaccine was also reported to be highly stable, requiring no extra adjuvant. |
104
|
Anthrax toxin antigens, lethal factors and their domains, and protective antigens |
Anthrax |
Multiple anthrax antigens, including up to 1662 anthrax toxin antigens, lethal factor and their domains, and protective antigens, were displayed on N and C termini of the small outer capsid (Soc) protein and the Hoc protein of bacteriophage T4 capsid. |
Presented an effective vaccine model against anthrax |
105
|
Anthrax toxin, lethal and oedema factors, functional domains and protective antigens |
Anthrax |
Bacteriophage T4 capsid engineering, multiple display and expression of anthrax toxin, lethal and oedema factors, functional domains and protective antigens on all the binding sites (155) of the N-terminal hexahistidine-tagged Hoc. |
Elicitation of potent antigens-specific antibodies against anthrax |
103
|
Nef, P24-gag, and modified gp41 C peptide trimer |
HIV vaccine development |
Multiple displays of human immunodeficiency virus (HIV) antigens, including Nef, P24-gag, and modified gp41 C peptide trimer on bacteriophage T4 capsid Hoc sites |
The study finds potential applications in HIV vaccine development. The in vivo evaluation of the engineered capsid bearing the HIV antigens demonstrated elicitation of strong p24-specific and Th1 and Th2 cellular antibodies and responses in mice, even without an adjuvant. |
106
|
Phages and molecules' chemical conjugation |
Tobacco mosaic virus |
Amines and oxime formation sequence/diazonium coupling |
— |
Respective capsid interior and exterior attachment of amines to the glutamic side chain via carbodiimide coupling and oxime formation sequence/diazonium coupling, installing many thousand copies of material on the tobacco mosaic virus, demonstrating the associated orthogonalities. |
Presented functional application of VLP nanoscale materials. |
107
|
Bacteriophage M13 |
Chlorin e6 (Ce6) |
SKOV3 and COV362 ovarian cancer cell lines |
Conjugated the bacteriophage M13 capsid with chlorin e6 (Ce6) assayed killing effectiveness against SKOV3 and COV362 ovarian cancer cell lines |
Generated reactive oxygen species (ROS) via the type 1 mechanism, increased uptake of the resulting M13-Ce6 within the mitochondria, targeting the epidermal growth factor receptor (EGFR), and inducing autophagy against the cancer cell lines. |
108
|
Polymer coating |
Bacteriophage M13 |
Poly(caprolactone-b-2-vinyl pyridine) and folate |
Cancer |
Poly(caprolactone-b-2-vinyl pyridine) and folate (conjugated to phage surface N-terminal and lysine residue) to form a nanocore shell that encapsulated doxorubicin (an antitumor agent) |
Increased release and cellular uptake of the doxorubicin, cytotoxicity via receptor-mediated endocytosis, and higher selective uptake in tumour cells. |
26
|
CN8 bacteriophages |
Polyvinyl polymers with ether, alcohol, and pyrrolidone functional groups |
Goss's witt |
Stabilised CN8 bacteriophages specific against Clavibacter michiganensis subsp. nebraskensis associated with Goss's witt, leading to yield loss in maise seeds using polyvinyl polymers with ether, alcohol, and pyrrolidone functional groups. |
Increased stability of the CN8 with the polyvinyl alcohol providing the highest stability, allowing long-term storage (four (4)–seven (7) months) under different temperature conditions combined with whey protein isolate and with no negative impact on the seed germination, significantly reduced bacterial loads in the contaminated seedlings. |
121
|
Phages of methicillin-resistant S. aureus (MRSA) |
Linezolid, hydroxypropyl methylcellulose |
Methicilin-resistant S. aureus infection |
Employed phages of methicillin-resistant S. aureus (MRSA) implicated in orthopaedic device-related infections, linezolid, and polymer (hydroxypropyl methylcellulose) in the coating of wires implanted into the intramedullary canal of the femur of experimental animals in a simulation of surgical implantations, after which methicilin resistant S. aureus ATCC 433000 was inoculated within the implantation area. |
Phage-linezolid-biopolymer coating maximally reduced bacterial adherence and joint inflammation, prevented the appearance of resistant mutants, and increased motor function of the limb and resumption of locomotion. |
109
|
T7 phage |
Cysteine constrained heptapeptide |
Cystic fibrosis |
Following the screening of peptide–presenting phage and high throughput sequencing techniques, identified T7 phage-displayed cysteine-constrained heptapeptide serving as phage coating. |
Enhanced drug penetration through the mucus, uptake into the epithelial cells of the lungs, distribution uniformity, and retention in the lung airways in treating cystic. |
110
|
Bacteriophage MS2 |
PEG chain surface coating, fluorescein |
— |
Dual modification using orthogonal coupling, including the site-specific PEG chain surface coating and interior surface conjugation with several copies of fluorescein (fluorescent dye) on bacteriophage MS2 capsid was reported by Kovacs et al.111 |
Achieved the intact assemblage of the capsid following the extensive modifications and 90% reduction/blockage of the interaction of the phage capsid surface and polyclonal antibodies. Additionally, the polymer-attached biotin group retained the streptavidin binding ability even though it was placed close to the PEG layer. |
111
|
Phage capsid manipulations and encapsulation |
Bacteriophage T3 |
Gelstar, bleomycin |
Cancer cells |
Loading of Gelstar and bleomycin incubated with the phage capsid at the appropriate temperature (45 °C). The confirmation of loading was determined using the band fluorescence produced by the compounds in dry native gel electrophoresis. |
Loaded DNA emitting greenish colouration as expected. The engineering could adequately protect the loaded compounds through the blood/plasma and present the potential for the specific monitoring and delivery of anticancer drugs (often susceptible to blood metabolic interruption) to tumour cells. |
112
|
Phage T4 |
Ethidium, bleomycin |
Cancer cells |
Loading of ethidium and bleomycin |
Presented the gating and protective potential of phage T4, which at 4 °C for a month and 37 and 42 °C for 6 days, maintained the integrity of the loaded compounds within the loaded phage capsids. The engineering could adequately protect the loaded compounds through the blood/plasma and present the potential for the specific monitoring and delivery of anticancer drugs (often susceptible to blood metabolic interruption) to tumour cells. |
113
|
PRD1 |
Chlorpromazine |
Antipsychotic conditions |
The mode of binding and organisation of chlorpromazine complexed with PRD1 |
The PRD1 membrane enabled the ejection of the phage DNA. The injection of the drug was achieved, showing the accumulation of the drug at specific locations in the PRD1 capsid while maintaining the stability of the virions. The accumulation was attributed to the formation of micelles, polar N,N-dimethyl propylamine moiety associated with the negatively charged residues at the capsid protein P3 jellyrolls, and the formation of turreted morphology, leading to the packed and specific hexagonal lattice formation. Additionally, the drug formed aromatic rings between the guanidium group of arginine and the indole ring of the tryptophan side chain. |
79
|
Attachment of multi-targeting ligands/moieties |
Filamentous phage |
Phage proteins (pVIII), paclitaxel, 1,2-distearoyl-sn-glycero-3-phosphoethanolamine-N-[methoxy(polyethyleneglycol)-2000 |
Breast cancer |
MCF-7 cancer cell-specific filamentous phage proteins, pVIII, were used in the preparation of phage-paclitaxel micelles after phage self-assembling with the drug and 1,2-distearoyl-sn-glycero-3-phosphoethanolamine-N-[methoxy(polyethyleneglycol)-2000 conjugates. |
The directed targeting of breast cancer cells showed in vitro and in vivo a dramatic tumour reduction and necrosis and apoptosis, respectively. The therapy was also considered safe following the absence of hepatoxicity or pathological tissue changes. |
114
|
Phage clone library |
121 phage-displayed neuroblastoma-binding peptides |
Neuroblastoma |
Using molecular targeting and a phage clone library, identified a total of 121 phage-displayed neuroblastoma (childhood tumour)-binding peptides. |
26, 15, 57 and 23 of the peptides specifically targeting the primary tumour, metastatic mass, and specific microenvironments. These were also demonstrated and validated ex vivo and in vivo following the coupling of some of the peptides with doxorubicin-loaded liposomes with a corresponding consequential significant reduction and enhancement of tumour volume and survival rates. |
115
|
Image tagging |
Bacteriophage M13 |
Folic acids, fluorescent molecules |
Human cancer cell |
Using bacteriophage M13 capsid surface and through conjugation with folic acids targeting cancer cells and small fluorescent molecules, they modified three (3) reactive groups, including the amino group of lysine, carboxylic acids groups of aspartate, and phenol group of tyrosine residues. |
A highly fluorescent bacteriophage M13 possesses significant binding affinity to human cancer cells, enabling targeting and probing. |
116
|
Filamentous phage |
Ketones, alkoxyamine groups (fluorophores) |
Breast cancer |
The N-terminal amines of the proteins were converted into ketones, which served as the chemo-specific handles for attaching alkoxyamine groups (fluorophores) via oxime formation and many 2 kDa poly(ethylene glycol) molecules. |
There is no effect on the resulting single-chain antibody fragment (displayed in the phage coat proteins) binding to the human epidermal growth factor receptor 2 and epidermal growth factor receptor. The phage modification potentially enabled breast cancer diagnostic applications. |
117
|
Bacteriophage M13 |
Isothiocyanate of fluorescein |
Multiple myeloma |
Most suitable M13-pVIII phage display clones were explicitly labelled with isothiocyanate of fluorescein. |
Provided fluorescent signals that separately identified multiple myeloma from individuals related to different CDs and presented a sensitive and rapid detection of the disease-associated immunophenotype subtypes and the disease status characterisation |
118
|
Genome modification |
Phage T4 |
Cytosine, glucosyl-hydro methylcytosine |
— |
Blocking the attack of CRISPR-Cas9 nuclease complexes, an adaptive immunity effector in bacteria, Bryson et al. edited and covalently replaced cytosine with glucosyl-hydro methylcytosine in Phage T4. |
Reported less susceptibility of modified Phage T4 (replacement of cytosine with glucosyl-hydro methylcytosine) to Cas9 nuclease attack compared to the unmodified phage T4 genome |
119 and 120
|
1. Peptide display.
Zhao et al.91 genetically modified Leviviridae PP7 capsid protein to display functional polypeptides, including one1 kDa-sized cell-penetrating peptide, fourteen14 kDa-sized Fc-binding Z-domain, and dimeric construct. Wild-type PP7 was reconstructed using extensions and insertions by respective C′-terminal extensions via polymerase chain reaction (PCR) by flanking with a 3′-sequence base of the primer attached to C′-terminal sequence and 5′-sequence region coding for the extensions and dimer loop insertions (also using similar PCR procedure), after which the construction was expressed and characterised. The result presented a more tolerable and robust system, displaying a linear and extended looped peptide (exogenous loops between the simultaneous presentation of 2 peptides and capsid monomers) and an open room for achieving desirable homogeneous polyvalent particles.
In targeting influenza A virus replication by competitive blocking of the virus and cell carbohydrate interaction, Gallego et al.99 reported the precise position of sialic acid moieties (ligand) utilising the phage (Bacteriophage Qβ) capsid scaffold strategy to achieve the structurally defined positioning of sialic acid matching the binding site of trimeric hemagglutinin of the virus. Following the identification of a capsid residue spaced 5–6 nm on the particle surface, close to the sialic acid binding site of hemagglutinin, the expression in the presence of L-homopropargylglycine introduced a propargyl group using azide-alkyne cycloaddition and different linkers to anchor the sialic acid ligands. The in vitro result showed enhanced binding affinity of the Qβ capsid to the virus and potent inhibition of the virus, including outperforming oseltamivir (an antiviral agent). The ex vivo and in vivo results also significantly reduced viral titre and body weight loss, respectively.
Additionally, Rhee et al.,100 using a modular system, including specifically engineered RNA–protein interactions and involving chemical derivatisation by acylation and azide-alkyne cycloaddition, encapsulated many copies of fluorescent proteins on Qβ VLP, generating high-affinity carbohydrate-based ligands of the CD22 receptor and particles with similar photochemical and structural properties to monomeric analogue with more stability to thermal and proteolytic degradation.
Similarly, Goodall et al.101 utilised multivalent modulation to display the CD40 ligand (CD40 L), an immuno-stimulating ligand of the tumour necrosis factor superfamily with a trimeric quaternary structure on the exterior of bacteriophage P22 VLP, which results in the indication of higher potency in immunomodulation, following the significant decrease in the half maximal effective concentration (EC50) compared to the control CD40 and finding application in the management of cancer and other immunologically based diseases. Confocal laser microscopy and flow cytometry were used to detect the specific cell labelling by the particles. Specific sequences were displayed on RNA bacteriophage MS2 (a VLP) by Peabody et al.,102 with potential for application in HIV vaccine development. The results showed improved potent immunogenicity of the VLP, following the engineering and display of V3 and ECL2 loop peptides of HIV gp120 and CCR5 coreceptor, respectively. Though interrupted by protein folding, it was effectively suppressed by the genetic fusion of two identical polypeptides of the VLP coat protein into a single-chain dimer, which supported the display of diverse immunogenic peptides.
2. Antibody display.
In antigen-antibody display, bacteriophage T4 has been extensively employed, most involving the two2 highly antigenic outer capsid (Hoc) and small outer capsid (Soc) proteins that bind to localised capsid sites.103
In the advancement of vaccine research against the highly pathogenic Bacillus anthracis and Yersinia pestis, respectively, implicated in severe anthrax and plague, Tao et al.104 reported the potentiation of the in vivo clearance of implicated bacteria through the enhanced elicitation of the broad T-helper 1 and 2 immune responses, following the engineering of the bacteriophage T4 120- by 86 nm capsids, through the multivalent presentation of the Y. pestis 56 kDa-sized type 3 secretion system low-calcium response V antigen and anthrax 83 kDa-sized protective antigen and mutated capsular antigen F1 fused to the 9 kDa-sized Soc protein. The resulting vaccine was also reported to be highly stable, requiring no extra adjuvant. Similarly, Li et al.105 successfully presented multiple anthrax antigens, including up to 1662 anthrax toxin antigens, lethal factor and their domains, and protective antigens were displayed on N and C termini of the small outer capsid (Soc) protein, and the Hoc protein of bacteriophage T4 capsid and also presented an effective vaccine model against anthrax.
In addition, Shivachandra et al.103 showed using mice the elicitation of strong antigen-specific antibodies against anthrax following bacteriophage T4 capsid engineering, multiple display and expression of anthrax toxin, lethal and oedema factors, functional domains, and protective antigens on all the binding sites155 of the N-terminal hexahistidine-tagged Hoc. Sataliyawala et al.106 also utilised the Hoc-capsid interaction in the multiple displays of human immunodeficiency virus (HIV) antigens, including Nef, P24-gag, and modified gp41 C peptide trimer on bacteriophage T4 capsid Hoc sites, sufficiently saturated. The study finds potential application in HIV vaccine development, with the in vivo evaluation of the engineered capsid bearing the HIV antigens using mice showing, in the absence of adjuvant, the elicitation of strong p24-specific and Th1 and Th2 cellular antibodies and responses, respectively.
3. Chemical conjugation of phages and molecules.
Schlick et al.107 reported the respective capsid interior and exterior attachments of amines to the glutamic side chain via carbodiimide coupling and oxime formation sequence/diazonium coupling, installing many thousand copies of material on the tobacco mosaic virus, which demonstrates the associated orthogonalities and presents a functional application of VLP as nanoscale materials. The SKOV3 and COV362 ovarian cancer cell lines were photodynamically targeted using bacteriophage M13 by Bortot et al.108 They conjugated the phage capsid with chlorin e6 (Ce6), which on irradiation synergistically generated reactive oxygen species (ROS) via the type 1 mechanism, increased uptake of the resulting M13-Ce6 within the mitochondria, targeted the epidermal growth factor receptor (EGFR), and induced autophagy in cancer cell lines.
4. Polymer coating.
Suthiwangcharoen et al.26 used cancer cells assembled with bacteriophage M13, poly(caprolactone-b-2-vinylpyridine) and folate (conjugated to the phage surface N-terminal and lysine residues) to form a nanocore shell that encapsulated doxorubicin (an antitumor agent), which on characterisation showed increased release and cellular uptake of doxorubicin, cytotoxicity via receptor-mediated endocytosis, and higher selective uptake in tumour cells. Kimmelshue et al.93 used polymers to stabilise CN8 bacteriophages specific against Clavibacter michiganensis subsp. nebraskensis associated with Goss's witt, yielding loss in maise seeds. The polymers were polyvinyl polymers with ether, alcohol, and pyrrolidone functional groups. The study showed increased stability of CN8, with the polyvinyl alcohol providing the highest stability, which allows for long-term storage (four (4)–seven (7) months) under different temperature conditions combined with whey protein isolate, and without a negative impact on seed germination, significantly reducing bacterial loads in the contaminated seedlings.
In an animal model, Kaur et al.109 employed phages of methicillin-resistant Staphylococcus aureus (MRSA) implicated in orthopaedic device-related infections, linezolid, and polymer (hydroxypropyl methylcellulose) in the coating of wires, which were implanted into the intramedullary canal of the femur of experimental animals in a simulation of surgical implantations, after which methicilin-resistant S. aureus ATCC 433000 was inoculated within the implantation area. Compared to the control, the phage-linezolid-biopolymer coating maximally reduced bacterial adherence and joint inflammation, prevented the appearance of resistant mutants, and increased the motor function of the limb and resumption of locomotion.
To enhance drug penetration through the mucus, uptake into the epithelial cells of the lungs, distribution uniformity, and retention in the lung airways in treating cystic fibrosis, Leal et al.,110 following the screening of peptide – presenting phages and high-throughput sequencing techniques, identified T7 phage-displayed cysteine-constrained heptapeptide serving as phage coating, which ex vivo among the already stated and achieved objectives increased mucus penetration up to a 600 fold. Dual modification using orthogonal coupling, including the site-specific PEG chain surface coating and interior surface conjugation with several copies of fluorescein (fluorescent dye) on bacteriophage MS2 capsid was reported by Kovacs et al.111 and achieved the intact assemblage of the capsid, following the extensive modifications and 90% reduction/blockage of the interaction of the phage capsid surface and polyclonal antibodies. Additionally, the polymer-attached biotin group retained its streptavidin binding ability, even though it was placed close to the PEG layer.
5. Phage capsid manipulation.
Murine blood persistent bacteriophage T3 capsid, with gating capacity, was utilised by Serwer et al.112 in the loading of fluorescent compounds gelstar (a dye) and bleomycin (an anticancer agent). The compounds were incubated with the phage capsid at the appropriate temperature (45 °C). The loading confirmation was determined using the band fluorescence produced by the compounds in dry native gel electrophoresis, with the loaded DNA emitting greenish colouration as expected. Moreover, using similar procedures, Serwer and Wright113 achieved the loading of ethidium and bleomycin (also a fluorescent compound), presented the gating and protective potential of phage T4 at 4 °C for a month and 37 and 42 °C for 6 days and maintained the integrity of the loaded compounds within the loaded phage capsids. These studies showed that engineering could adequately protect the loaded compounds through the blood/plasma and present the potential for the specific monitoring and delivery of anticancer drugs (often susceptible to blood metabolic interruption) to tumour cells.
Additionally, the mode of binding and organisation of chlorpromazine, an antipsychotic amphipathic medication, complexed with PRD1 A (an icosahedral membrane-containing and double-stranded DNA phage) capsid protein P3 (43 kDa), in trimeric units, was reported by Duyvesteyn et al.79 The results showed that although the PRD1 membrane enabled the ejection of phage DNA without biochemical or genetic modifications, the injection of the drug was achieved following specific mixing procedures with PRDI, with subsequent cryo-electron microscopy, crystallography, and molecular and biochemical dynamic techniques showing the accumulation of the drug at particular locations in the PRD1 capsid while maintaining the stability of the virions. The accumulation was attributed to the formation of micelles, polar N,N-dimethyl propylamine moieties associated with the negatively charged residues at the capsid protein P3 jellyrolls, and the formation of turreted morphology led to the packed and specific hexagonal lattice formation. Furthermore, the drug formed aromatic rings between the guanidium group of arginine and the indole ring of the tryptophan side chain.
6. Attachment of multi-targeting ligands/moieties.
Multi-targeting phage protein micelles with specific interactions with tumour and vasculature surrounding cells ligated with paclitaxel, utilised in treating metastatic breast cancer, were reported by Petrenko and Torchilin.114 To achieve this, MCF-7 cancer cell-specific filamentous phage proteins, pVIII, were used in the preparation of phage-paclitaxel micelles after phage self-assembling with the drug and 1,2-distearoyl-sn-glycero-3-phosphoethanolamine-N-[methoxy(polyethyleneglycol)-2000 conjugates. The directed targeting of breast cancer cells showed a dramatic tumour reduction and necrosis and apoptosis in vitro and in vivo. The therapy was also considered safe following the absence of hepatoxicity or pathological tissue changes. In addition, Loi et al.,115 using molecular targeting and a phage clone library, identified 121 phage-displayed neuroblastoma (childhood tumour)-binding peptides with 26, 15, 57 and 23 of the peptides specifically targeting the primary tumour, metastatic mass, and specific microenvironments. These were also demonstrated and validated ex vivo and in vivo following the coupling of some of the peptides with doxorubicin-loaded liposomes with a corresponding consequential significant reduction and enhancement of tumour volume and survival rates, respectively. These results open room for rapid diagnosis and targeted treatment of these diseases.
7. Image tagging.
Li et al.116 showed the potential application of phage display through chemical modification in cancer cell imaging and probing. Using bacteriophage M13 capsid surface and through conjugation with folic acids targeting cancer cells and small fluorescent molecules, they modified three reactive groups, including the amino group of lysine, carboxylic acid groups of aspartate, and phenol group of tyrosine residues, producing a highly fluorescent bacteriophage M13 that possessed significant binding affinity to human cancer cells, which enables targeting and probing. Carrico et al.117 utilised multicolour fluorescence to allow for the characterisation of breast cancer cells by modifying a filamentous phage with approximately 4200 coat proteins. The N-terminal amines of the proteins were converted into ketones, which served as the chemo-specific handles for attaching alkoxyamine groups (fluorophores) via oxime formation and many 2 kDa poly(ethylene glycol) molecules, and this did not affect the resulting single-chain antibody fragment (displayed in the phage coat proteins) binding to the human epidermal growth factor receptor 2 and epidermal growth factor receptor. These receptors are prevalently associated with breast cancer, and phage modification potentially enabled breast cancer diagnostic applications.
Similarly, De Plano et al.118 utilised a phage probe for different clusters of differentiation as a diagnostic marker for multiple myeloma. The CDs enable the selection of aberrant and normal myeloma plasma cells associated with multiple myeloma.118 To achieve discrimination, the most suitable M13-pVIII phage display clones were explicitly labelled with isothiocyanate of fluorescein, in which ex vivo provided fluorescent signals that separately identified multiple myeloma from individuals related to different CDs. The study presented a sensitive and rapid detection of the disease-associated immunophenotype subtypes and the disease status characterisation.
8. Genome modification.
Attempting to block the attack of CRISPR-Cas9 nuclease complexes, an adaptive immunity effector in bacteria, Bryson et al.119 edited and covalently replaced cytosine with glucosyl-hydro methylcytosine in Phage T4, with the results showing the promise of averting bacterial resistance to phages with a resultant intermediate resistance of the Phage T4 mutant against the unmodified cytosine bearing Phage T4 that was sensitive. Similarly, Tao et al.120 reported less susceptibility of modified Phage T4 (replacement of cytosine with glucosyl-hydro methylcytosine) to Cas9 nuclease attack compared to the unmodified Phage T4 genome.
Generally, compared to other nanocarriers, bacteriophage applications as nanocarriers in drug development present desirable advantages over alternative nanocarriers. Specifically, their biological origin makes them biocompatible and stable, thus minimising the risk of immunogenic reactions, as seen in other nanocarriers, such as polymeric nanoparticles, micelles, and niosomes, which are also rapidly released.122 They can be engineered to target specific bacteria or cells, thus allowing for precision in drug delivery. They have ease of modification, enabling the incorporation of payloads. They also have a high cargo packaging capacity, allowing the carriage of substantial therapeutic agents, a limitation of most polymeric micelles and liposomes with limited cargo loading capacities.123 They are also less toxic than microemulsions, nanoemulsions, dendrimers, metal and metal oxide nanocarriers, organic, inorganic, hybrid nanocarriers, and carbon-based nanotubes.
3.2.2 Enhanced therapeutic effects of bacteriophage-mediated drug delivery.
Bacteriophage-mediated drug delivery systems offer many benefits, including increased drug loading, stability, site-specific targeting, reduced toxicity, synergistic effects with antibodies and improved therapeutic efficacy. These benefits are discussed in this subsubsection.
1. Increased drug loading, stability and efficacy.
Despite advancements in modern-day medicine, the issues of controlled and site-specific drug delivery, solubility, and increased bioavailability have remained largely unresolved. Drug hydrophobicity has been associated with decreased efficacy, as only a fraction of the active ingredients is delivered to the site of action.85 The design of highly targetable and specific carrier systems, such as nanoparticles, nanospheres, liposomes, foams, carbon nanotubes, dendrimers, hydrogels, and other biomaterials, has considerably impacted drug delivery. Ideal carrier systems are expected to have specific physicochemical properties. Also considered are the effects on cellular uptake, intracellular distribution, accumulation, retention, and excretion of these vehicles.124 While synthetic and semi-organic carriers such as nanoparticles, quantum dots, dendrimers, vesicles, and liposomes all possess these qualities, natural carrier systems such as viruses and virus-like particles, specifically bacteriophages, have been shown to present more advantages in terms of biocompatibility, improved pharmacokinetics, low toxicity and immunogenicity.62,125 Considering the natural capacity of viruses to invade and infect host cells, advancements in nanotechnology have revolutionised viruses as the safe and efficient delivery of therapeutic and genomic materials.85
Bacteriophages have an excellent capacity for packaging therapeutic payloads, including genetic materials, and they are relatively categorised as safe in humans. These advantages make phages great candidates for nanocarriers.21 It is believed that bacteriophages can control the many adverse effects of conventional therapeutic agents owing to their inherent biocompatible nature, which would, in turn, enhance treatment efficacy. Combining phages and therapeutic compounds could generate hybrid vehicles that serve as highly potent drug delivery systems.21 Phages are viable biomaterials capable of being manipulated into hybrid bacteriophage-based nanocarriers in combination with various therapeutic moieties. The conjugation of inorganic materials onto the surface of phages displaying ligands introduces novel properties to phage carriers.
Additionally, phage conjugation with therapeutic substances, such as drugs, metabolites, and growth factors, enables the delivery of higher concentrations of therapeutic payloads without any unwanted effects on healthy surrounding tissues and cells. Phage-based nanocarriers could increase the solubility of poorly water-soluble drugs usually through materials on the phage coat that can serve as solubilising and sequestering agents. These delivery systems can equally present improved stability under certain pH and temperature conditions.126
In a study conducted by Yacoby et al.,92 a highly toxic antibacterial agent, chloramphenicol, was attached, as a prodrug, to p8 coat protein molecules on the surface of the m13 phage subtype A12C. Upon release of active chloramphenicol against S. aureus, partial growth inhibition was observed, which was interpreted as a possible limitation in drug loading primarily owing to the hydrophobic nature of chloramphenicol. This challenge was improved in subsequent studies in which a conjugation method involving aminoglycosides as branched, solubility-enhancing linkers was adopted, resulting in a drastic increase in the drug-loading capacity of the phages. It was noted that over 40
000 chloramphenicol molecules/phages were loaded without disrupting the phage composition. However, about 10
000 molecules/phages were sufficient to produce the desired result, ultimately leading to an improved phage-antibiotic formulation. The results from this study demonstrated an improvement factor of approximately 20
000 compared to the free drug. Another study showed rapid uptake and enhanced antibiotic efficacy of phage-antibiotics upon conjugation of azithromycin to bacteriophage Qβ both in vitro and in mouse lungs. The results also indicate the possibility of adopting this formulation to achieve more efficient antibiotic treatment. This strategy could also be explored for pulmonary drug delivery.127 The enhanced therapeutic effect of phage-mediated drug delivery due to its ability to take up more than one therapeutic material was demonstrated by Stephanopoulos et al.128 by dual modification of the MS2 coat protein, generating a target against Jurkat leukemic T-cell therapeutic nanocarrier. A cell-specific DNA sequence was attached to the phage envelope, and the porphyrins were bound to the phage interior. Upon delivery of the cargo to cells of interest, there was illumination of porphyrins, which resulted in the release of a significant amount of reactive oxygen radicals. This reaction was achieved via a photodynamic effect. The generation of oxygen radicals led to the destruction of the target cells within 20 minutes. The integration of nanotechnology with phage techniques has led to the development of novel strategies to improve therapeutic activities and reduce the toxic effects of chemotherapeutic agents.129 A study assessing the anti-tumour activity of MCF-7-specific phage fusion protein-modified liposomal doxorubicin (Doxil) resulted in more rapid and effective antitumor activities than the non-targeted formulations.130 There was a higher accumulation of phage-Doxil in the tumour cells. Very mild side effects were noted in vivo, and hepatotoxicity tests were negative in the phage-doxil-treated mice. Gan et al.131 conducted a study to stop neovascularisation caused by vascular endothelial growth factor (VEGF) and its receptor VEGFR2. By conjugating VEGFR2 to T4 phages, T4-VEGFR2 was obtained. The VEGFR2-phage was then cultured with endothelial cells. There was a significant impairment of neovascularisation due to the inhibition of VEGFR2 phosphorylation and downstream signalling. It was also observed that upon administration of T4-VEGFR2 phages through the intravenous route to experimental animals with lung and colon cancer, there was notable inhibition of tumour growth and vascularisation.
2. Specific targeting and site-specific delivery.
Specific barriers affect the permeability of therapeutics. For example, the difficulty in shuttling drugs across the blood-brain barrier (BBB) can impair drug delivery to the central nervous system (CNS). Viruses can intrinsically recognise and attach to specific cell receptors and deliver genes or drugs (Fig. 4). This unique characteristic makes them ideal candidates for targeted delivery systems.132 The display of tissue-targeting peptides on the phage coat was shown to deliver therapeutic compounds into cardiac myoblasts. Zahid et al.133 modified M13 surface proteins with an APWHLSSQYSRT peptide sequence, enabling the affinity of the phages to cardiac myoblasts. The capsid of M13 has also been modified for breast tissue-specific delivery of therapeutics by adding targeting peptides, such as VOWMEPAYQRFLGGG. This phage capsid-peptide combination was able to recognise breast cancer tissues and neuroblastoma cells.134 More interestingly, filamentous phages can also penetrate the blood-brain barrier, and this property could make them suitable for use in the treatment of Alzheimer's disease.135 Rakover et al.136 discovered that the fibrous nature of filamentous phages enables their permeation into the central nervous system via nasal administration. They conjugated myelin oligodendrocyte glycoprotein immunodominant epitope (MOG 36-44) on a filamentous phage as an immune-recognising epitope for the treatment of multiple sclerosis. This setup was proposed to function through the inactivation of demyelinating antibodies in the central nervous system. There was significant termination of autoantibodies against MOG and demyelination, which led to reduced inflammation in the CNS and periphery as well as enhancement of overall clinical outcomes in treated mice. The above studies show that there is potential for phages to be loaded with therapeutic agents that can be delivered to target sites, which are challenging to target.
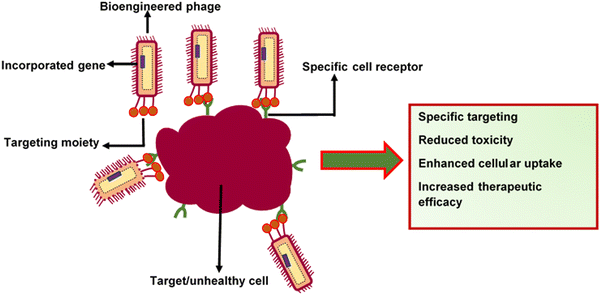 |
| Fig. 4 Specific cell targeting using bioengineered bacteriophages. | |
To date, the liver has remained one of the most challenging sites for drug delivery. The option of injecting therapeutic agents into liver tissues has been explored. However, the detection of these agents by Kupffer cells stimulates undesirable immune responses.137 It has been observed that by labelling the tail fibre protein (P17) of the T7 phage with the asialoglycoprotein receptor (ASGPr), drugs could be successfully delivered into hepatocytes.138 Wong et al.138 adopted this principle by attaching a targeting ligand composed of 33 amino acids to P17 to transfer siRNA, liposomes, and DNA polyplexes into hepatocytes. Ashley et al.95 established phages as highly reliable cargoes for delivering diagnostic agents, nanoparticles, and siRNA molecules. In their work, MS2 virus-like particles (VLPs) modified with a peptide (SP94) that binds to hepatocellular carcinoma (HCC) exhibited a 104-fold higher avidity for HCC than for hepatocytes, endothelial cells, monocytes, or lymphocytes. This modification also enabled the delivery of higher concentrations of therapeutic agents to the cytosol of HCC cells.139 Vaks and Benhar140 presented a method involving both the genetic and chemical modifications of filamentous phages to achieve targeted delivery. Through genetic manipulation, phages can display host-specificity-conferring ligands, and by chemical conjugation, cytotoxic drugs can be loaded into the phages. Selective lysis of cancer cells was achieved upon detection of target cells and delivery of therapeutic substances. Scibilia et al.141 reported a different kind of phage-based formulation. Here, a hybrid-nanostructured carrier consisting of an M13 P9b phage clone co-formulated with silver nanoparticles (AgNPs) was developed and used in a therapy targeted against Pseudomonas aeruginosa.
3. Synergistic effects of antibiotics.
Phages have long been used as nanocarriers for the delivery of antibiotics. The significant antibacterial improvement obtained with chloramphenicol, as reported by Yacoby et al.,92 is because of the increased drug payload from 3000 to 10
000 molecules/phage. Additionally, the synergistic effect was attributed to the improved solubility and possible bioavailability of the entire phage-based antibiotic platform. It was noted that neomycin, used as the aminoglycoside linker, has antibiotic properties. However, the chemical linkage to the phage capsid was caused by a non-labile bond. Therefore, neomycin was not released and did not contribute to the obtained antibacterial effect.
4. Reduced side effects and toxicity.
In the study by Vaks and Benhar,140 an antibody was displayed on the pIII minor coating protein of the f1 phage, with chloramphenicol also chemically conjugated to the phage. This conjugation to a filamentous phage resulted in controlled toxicity and side effects of chloramphenicol at a therapeutic dose. Du et al.142 employed a 12-mer commercial M13-displayed library for the in vivo biopanning of xenograft models of BEL-7402 hepatocarcinoma (HCC). Phages were administered intravenously, after which specific tumour-enriched phage clones displaying AGKGTPSLETTP peptides were selected from tumour masses in mice. The therapeutic agent doxorubicin (Dox) was conjugated to the A54 peptide, enabling a strong antitumor effect in HCC tumour-bearing mice. No side effects were observed from this formulation compared to treatment with the drug in its unconjugated state.
4. Applications of bacteriophage-mediated drug delivery systems
As nanocarriers, bacteriophage-inspired drug delivery systems have been applied in cancer therapy, gene therapy, treatment of bacterial infections, vaccination and detection of serological biomarkers, as illustrated in Fig. 5. These applications are described below.
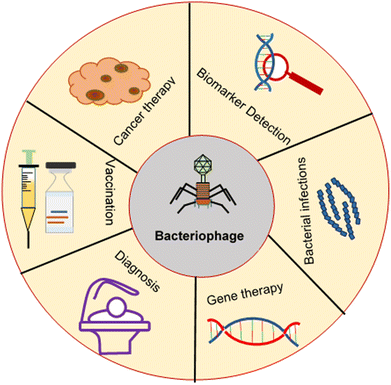 |
| Fig. 5 Applications of bacteriophages. | |
4.1 Cancer therapy
Chemotherapeutic agents delivered using conventional drug delivery systems pose several challenges, such as low drug concentration at the target site, high toxicity resulting from a wide distribution in the body, and the induction of drug resistance. To overcome this problem, the use of bacteriophages as bio-inspired nanocarriers has attracted attention in recent years. Bacteriophages offer many benefits over traditional delivery systems in cancer therapy.143 Such benefits include their favourable physicochemical properties of nanosized and polyvalent surface morphology. In addition, phages do not exhibit mammalian cell tropism; therefore, they do not affect normal human cells. This allows engineered phages with peptide displays on their surfaces to be targeted to specific tumour cells. Some bacteriophages can avoid clearance by the reticuloendothelial system, thereby exhibiting a long circulation time in the body and increased bioavailability.144 In addition, bacteriophages are non-toxic, cost-effective, and easy to produce with high scalability potential.145,146 Phage-based nanocarriers have been investigated in cancer therapy via phage display technology, production of DNA vaccines, genes, and targeted drug delivery using MS2, Lambda, T4, M13, T7, and QB bacteriophages.
Bacteriophages can induce immunity and are studied for use as carriers of cancer vaccines. The phages are taken up by antigen-presenting cells, while the peptide (antigen) on its surface is presented via major histocompatibility complexes (MHCs). The antigen presentation on MHC leads to the activation of CD8+ T and CD4+ T cells into cytotoxic T lymphocytes and T helper cells, respectively. The former directly kills infected host cells, while the latter aids in producing specific antibodies by B cells.147 The mechanism of phage-induced immunity is shown in Fig. 6. Iwagam et al. (2017) investigated the antitumor effect of lambda (λ) phage vaccine against aspartate β-hydroxylase (ASPH) expressing murine liver tumours. The mice were vaccinated pre- and post-subcutaneous implantation of a hepatocellular carcinoma (HCC) cell line. A significant delay in the growth and progression of HCC was recorded after the prophylactic and therapeutic vaccination. Antigen (ASPH) specific CD4+ and CD8+ lymphocytes, Th1 and Th2 cytokines, were generated by tumour-bearing mice.148 Similarly, Wang et al. (2022) investigated M13 bacteriophages for both prophylactic and therapeutic vaccination against breast cancer. The phage was designed to display the extracellular and transmembrane domains of human HER2 or Δ16-HER2 (a splice variant of HER2) on their surface.149 These surface peptides aid in the navigation of the bacteriophage and the identification of target molecules. The onset of the mammary tumour was delayed, and the tumour growth rate was reduced in Δ16HER2 transgenic mice. HER2-specific antibody production correlates with the antitumor effect. The suggested mechanisms of this antitumor activity are the impairment of ERK phosphorylation and the reactivation of suppressor retinoblastoma protein function.149 Therefore, phage-based vaccines impair the onset and progression of tumours and can be a platform for cancer prophylaxis and treatment.
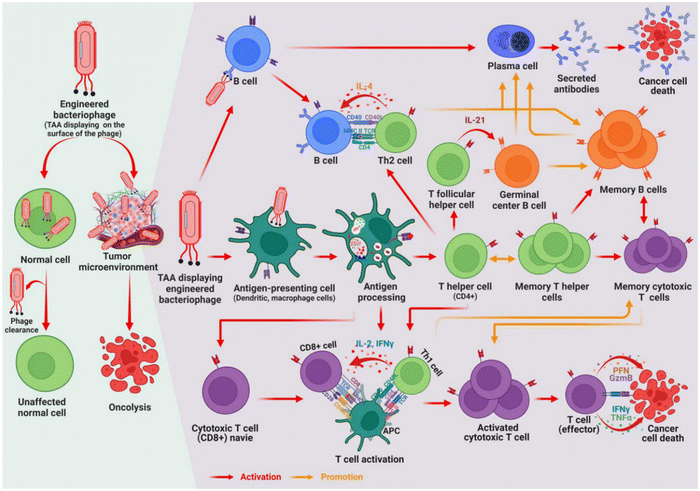 |
| Fig. 6 Mechanism of phage-induced immunity. Reprinted from Ragothaman et al., 2023 with permission from MDPI.150 | |
Bacteriophages are carriers of drugs, genes, and imaging agents. Through phage display technology, bacteriophages can be engineered to display functional peptides on their surfaces. Such proteins act as ligands interacting with receptors and biomarkers in the tumour cell microenvironment. This interaction increases active targeting and enhances chemotherapeutic penetration and retention effects. Thus, phage display technology has been widely investigated in targeted gene delivery systems. Chondrosarcoma exhibits treatment resistance using conventional methods of radiotherapy and chemotherapy.151 Chongchai et al.152 employed bacteriophage display technology to design a phage carrying a human tumour necrosis factor-alpha (TNFα) transgene cassette for targeting chondrosarcoma. The phage containing the incorporated gene was designed to display the endosomal escape peptide and RGD4C ligand to enhance gene delivery and specifically bind to integrin receptors (Fig. 7). The tumour cell overexpresses the integrin receptor and human tumour necrosis factor alpha (TNFα) receptors. Therefore, these receptors are targets in drug delivery to tumour cells. The expression of a high amount of TNFα and other apoptosis-related genes after treating the tumour cells with the phage led to significant cell death. Suppression of tumour growth in mice treated with the targeted bacteriophage was also reported.
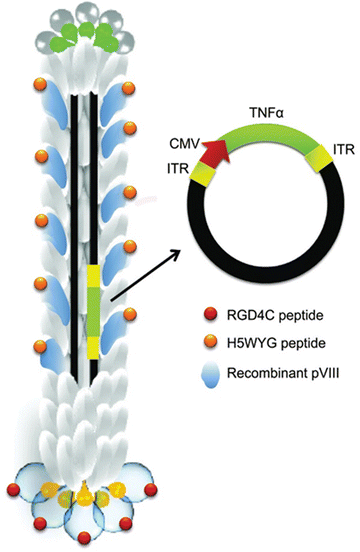 |
| Fig. 7 Schematic representation of the RGD4C-targeted phage encoding TNFα. Modified phage capsid with RGD4C ligand and endosomal escape peptide, H5WYG, displayed on the pIII minor and pVIII major coat proteins. The incorporated transgene expression cassette was composed of tumour necrosis factor alpha (TNFα) transgene, cytomegalovirus (CMV), and inverted terminal repeats (ITRs) cis-elements from adeno-associated virus (AAV2). Adapted from Chongchai et al., 2021 with permission from Wiley.152 | |
Another lethal resistant tumour investigated for treatment using bacteriophage-based suicide gene therapy is glioblastoma multiforme (GBM). Przystal et al.153 developed a multi-component M13 phage system consisting of a recombinant adeno-associated virus genome, a glucose-regulated protein (Grp 78), a chemotherapeutic agent (temozolomide), and a phage display protein (RGD4C). The RGD4C ligand binds to integrin receptors on tumour cells, while temozolomide induces the expression of the Grp78 gene and promotes transgene expression. This combination of gene therapy and chemotherapy has been reported to have a synergistic antitumor effect.
Hwang et al.154 designed a T7 bacteriophage displaying a Pep42 peptide targeting glucose-regulated protein 78 (GRP78) expressed in murine melanoma cells. A mammalian expression cassette of the cytokine granulocyte macrophage-colony stimulating factor (GM-CSF) was incorporated into the phage genomic DNA. There was 100% survival of mice treated with the phage, while the untreated group recorded only 40% survival within the study period. Tumour growth was remarkably inhibited in the treated group. Immunological responses were also observed during treatment. Such responses include increased antitumor immune cells, including IL-1α, TNF-α, and GM-CSF, and the migration of macrophages, dendritic cells (DCs), and CD8+ T cells into tumour cells.
Another strategy for cancer therapy is via the vascular endothelial growth factor (VEGF) signalling pathway. This pathway is essential in the angiogenesis of cancer cells. T4 phages displaying the extracellular domain of VEGF receptor 2 were investigated owing to their anticancer activity.155 The VEGF receptor 2 phages specifically bind to VEGF, causing the inhibition of VEGF-mediated phosphorylation of the VEGF receptor and other signalling pathways, including extracellular signal-regulated kinase (ERK) and p38 mitogen-activated protein kinase (MAPK). Hence, murine Lewis lung and colon carcinoma models hinder cell proliferation and tumour growth. In addition, the survival time was prolonged in the tumour-bearing mice.
Several reports have shown the potential of microRNAs in cancer therapy. However, instability and low cellular delivery efficiency hinder their use. Bacteriophages can be used to deliver microRNAs to cells and protect them from enzymatic degradation by RNase. Sun et al.156 developed PP7 bacteriophage-based virus-like particles to provide microRNAs using an E. coli expression system. They conjugated particles with cell-penetrating particles (transactivated transcription (TAT) peptide) to enhance the in vivo delivery of RNA. The authors reported that the phage virus-like particles modified with a penetrating peptide penetrated the liver cancer cells. The upregulation of RNA and the subsequent reduction of liver-intestine cadherin expression led to the suppression of hepatoma. Previous studies using phage virus-like particles conjugated to cell-penetrating peptides in liver cancer reported similar findings.157,158
A combination of photothermal and chemotherapy was studied using a genetically engineered T7 phage. Oh et al.159 investigated the T7 phage with a prostate cancer cell-binding peptide and a gold-binding peptide for targeted photothermal therapy of prostate cancer. Self-assembling gold nanoparticles and the engineered phage formed phage-gold nanoparticle clusters. Compared to non-targeted gold nanoparticles, the targeted phage-gold nanoparticles destroyed the cancer cells within few minutes when irradiated. The results demonstrated the uptake of phage-gold nanoparticles by human prostate carcinoma cells when treated with the engineered phage.159 This selective accumulation of nanoparticles presents a prostate cancer cell-targeted delivery.
4.2 Therapeutic effects against bacterial infections
Abuse of antibiotics in humans has led to the development and spread of antimicrobial resistance worldwide.160,161 Common multidrug-resistant organisms are ESKAPE pathogens, which include Enterococcus faecium, S. aureus, Klebsiella pneumoniae, Acinetobacter baumannii, P. aeruginosa, and Enterobacter species.162 Efforts are geared toward solving this public health issue. One of the approaches investigated is the use of bacteriophages. Their specificity and role in maintaining healthy microbiota make them an exciting and promising option. Phages can engage with pattern recognition receptors to activate immune signalling pathways and to generate and recruit immune cells to the infection site. Bioengineered phages with surface peptides are also engulfed by APC and presented to MHC to generate cytotoxic T lymphocytes and T helper cells to kill the infected cells directly or to produce antigen-specific antibodies against the causative organisms.
Eradication of ESKAPE pathogens using bacteriophages alone or with antibiotics has been reported in the literature.163,164 Diabetic foot ulcers are deadly diabetic complications characterised by infections with multidrug-resistant bacteria. Ghanaim et al.165 investigated the topical use of a phage cocktail to treat diabetic foot ulcers infected by S. aureus, P. aeruginosa, Klebsiella variicola, Escherichia coli, and Proteus mirabilis, with the therapy showing better wound healing and anti-inflammatory parameters than those treated with ceftriaxone. Hence, phage therapy could be an effective option for treating multidrug polymicrobial infections. MMI-Ps1 is a newly isolated phage with a powerful lytic effect. A single dose of this phage administered immediately or 12 hours post-infection exhibited a protective effect in a mouse model of acute lung infection with P. aeruginosa. The phage therapy also enhanced the complement-mediated lysis and elimination of the resistant Pseudomonas strain.166
Lytic enzymes from bacteriophages (lysins, endolysins, or enzybiotics) are potent antibacterial agents with faster killing rates than standard antibiotics. The benefits of using phage lysins as an antimicrobial agent include high specificity, activity against multidrug-resistant organisms, low toxicity, and low chances of resistance development.167 The antimicrobial activity of three lysins- wild-type Cpl-1, the engineered Cpl-7S, and the chimaera Cpl-711 were evaluated against a pneumococcal colonised nasopharyngeal mouse model.168 Human nasopharyngeal and lung epithelial cells were infected with three different multidrug-resistant clinical isolates of S. pneumonia, and a single dose of complementary lysin was given afterwards. The engineered Cpl-7S had no activity against the test organism, while Cpl-1 and Cpl-711 effectively killed the organism. Treatment with Cpl-711 showed superior antimicrobial activity against the multidrug-resistant organism than Cpl-1. Furthermore, a significant reduction in pneumococcal colonisation was observed in mice treated with Cpl-711, with no detection of bacterial load in 20 to 40% of the mice.168
In a randomised clinical trial, intravesical bacteriophage therapy exhibited efficacy similar to standard antibiotic therapy in treating urinary tract infections in patients undergoing transurethral resection of the prostate with a better safety profile.169 Apart from the efficacy of bacteriophages in treating diseases, their safety is of utmost importance. Fabijan et al.170 assessed the safety of phage therapy in patients with severe S. aureus infection. Patients who were given a phage cocktail of three Myoviridae bacteriophages (AB-SA01) intravenously twice daily for two weeks showed no sign of adverse reactions within a study period of 3 months.
4.3 Gene therapy
Gene therapy requires safe and efficient delivery systems that can target a specific cell or tissue. Viruses are natural carriers of genetic materials and can transfer the same into other biological systems.21 The intrinsic potential for bacteriophages and phage particles to be loaded with genetic materials makes them attractive nano-vehicles for gene delivery. Phages equally display foreign proteins on their surfaces, enabling targeted gene therapy to specific cells.140,171,172 Ghaemi et al.173 conducted several experiments to study lambda-ZAP bacteriophage-mediated gene transfer and expression in vitro. In their study, genes encoding an enhanced green fluorescent protein (EGFP) and E7 of human papillomavirus (HPV) were conjugated to recombinant lambda-phage nanobioparticles to enhance the anti-tumour immune response against HPV. Dual modification of bacteriophages is a promising strategy for improving targeted gene delivery in combating disease conditions. In this method, the phage surface displays specific ligands, while the genes of interest are encapsulated in the phage capsid, where they are protected from enzymatic degradation.174 Thomas et al.175 established that dual modification could elicit higher immune responses. Transacting activation transduction (TAT) peptides, which serve as cell-penetrating ligands, were used in a study conducted by Tian et al.,176 who conjugated this TAT peptide onto the exterior surface of a TMV phage. The TMV–TAT conjugation system successfully delivered green fluorescent protein (GFP) silencing RNA (siRNA) into GFP-expressing hepatocellular carcinoma tumours in vivo.
4.4 Diagnosis, disease detection, and monitoring
Traditional molecular imaging methods using one biomarker have resulted in inaccurate conclusions. Their use is also limited by the high cost of fabrication and the need for sophisticated handling. Therefore, multi-modification of phages could be used in the accurate and precise diagnosis of diseases, which would ultimately enable personalized therapy.177,178 Viruses, such as bacteriophages, can carry a wide range of targeting agents, immune-fluorescent labels and various markers to target particular cells and tissues. Viruses bearing imaging labels have the advantage of short retention times, making them easily removed from the system compared to other non-viral biomaterials. Virus-based nanocarriers have, therefore, been used in imaging processes, such as fluorescence imaging, magnetic resonance imaging (MRI), positron emission tomography (PET), computed tomography (CT), and others.179,180 The use of single-walled carbon nanotubes (SWNTs) in medical diagnosis has been flawed because of their lack of stability in biological environments. To proffer a solution, Yi et al.181 bound SWNTs to the pVIII of M13 phage peptides specific to prostate cancer. The aim was to generate a deep-tissue diagnostic imaging agent. From the M13-SWCNT phage, cell targeting was achieved with highly visible fluorescent images of the tumour, and more biologically stable SWNTs were obtained in the biological environment. It was, therefore, concluded that phage-assembled SWNTs may be used as reliable imaging agents. There have been reports of in vivo optical imaging with fluorescent dyes for the detection of bacterial infections using bacteriophage M13.182 They demonstrated that by conjugating these dyes to bacteriophage M13, F-pili expressing and F-negative strains of E. coli could be identified. The phage coat was equally modified by adding biotin acceptor peptides to enable the detection of other bacterial strains as well. It was reported that the biotinylated M13 phage specifically detected and targeted S. aureus infections. Phages engineered with fluorescent or radiolabelled peptides on their protein coats are usually employed in molecular imaging to distinguish between various cancer or metastatic cells. For example, in these studies, molecular imaging was carried out in vivo using green fluorescent protein (GFP) conjugated to phages.183,184 Using recombinant DNA technology, Choi et al.185 co-engineered a bacteriophage that expressed cyclic RGD (cRGD) peptides on the pVIII major coat protein. They proposed that upon modification of this cRGD peptide, engineered phages with therapeutic or imaging agents could find use in therapy or diagnosis. Bacteriophages can also be used in theranostics when loaded simultaneously with imaging biomarkers and therapeutics, as described by Dymova et al.186 using a tumor-targeting bacteriophage technology, diagnostic imaging and therapeutic agents were delivered to malignant triple negative MDA-MB-231 breast cancer cells. They proposed that modification of phages using modifying agents, such as FAM–NHS dye, enhanced internalization of the phages into cancer cells, specifically MDA-MB-231 cells.
Autoantibodies for disease conditions were obtained from serum. This indicates that serum can be used to screen, diagnose, or monitor diseases.187 In the diagnosis of serological biomarkers, large volumes of serum and serum samples are typically used, which is very costly. The T7 phage display system can detect serological biomarkers more accurately and cheaply. This was demonstrated by Talwar et al.188 in detecting antibodies reactive to Mycobacterium tuberculosis (MTB) antigens in bodily fluids. In this study, sarcoidosis, which has clinical and pathological characteristics similar to MTB, was used. Four T7 phage display cDNA libraries were prepared and screened using sera from healthy controls, sarcoidosis and culture-positive MTB patients. The results showed highly sensitive and specific biomarkers for MTB in the sera of subjects with culture-positive MTB.188 In a similar study by this group, T7 phages were immune screened with sera of smear-positive TB patients. This was done to identify specific diagnostic biomarkers from the sera of TB-positive patients. From the results, highly specific TB clones, which can be used to differentiate TB patients from healthy controls and sarcoidosis patients, were clearly detected.189 The pIII protein of filamentous phage nanocarriers can be used to display a targeting sequence for the bio-detection of specific antigens from sera, while diagnostic peptides can be engineered to the pVIII protein coat.190 Lee et al.190 showed that pVIII modification with plasmon-resonant gold nanoparticles could rapidly detect antigens initially recognized by peptides displayed on the pIII protein.
4.5 Detection of bacteria in food
To avoid developing and disseminating food-borne diseases, a strategy for food safety assessments could be achieved using genetically engineered bacteriophages. Wisuthiphaet et al.191 demonstrated this using phage T7-ALP, which expresses the enzyme alkaline phosphatase. By infecting the phage with E. coli, there was overexpression of alkaline phosphatase. This serves as a signal indicating bacterial presence in the model beverage samples. This process was followed by fluorescence imaging and image analysis for the more sensitive detection of single-cell bacteria. Another advantage of this method is the relatively short completion time of less than 6 h, which makes it ideal for use in major industrial food processing facilities with up to 8 h operational times. Another T7 phage conjugated to iron oxide magnetic beads was developed to rapidly detect and separate E. coli in drinking water.192 The T7 phages lysis E. coli cells and endogenous β-galactosidase were then detected using chlorophenol red-β-D-galactopyranoside, a colourimetric substrate, which changes from yellow to red in the presence of β-galactosidase. This strategy showed high sensitivity and specificity against a background of competing bacteria.
4.6 Phage-based vaccination
The application of phage-based nanocarriers in vaccination has emerged as an intriguing area for researchers. Immunity can be achieved with bacteriophages by direct vaccination with antigen-loaded phage particles or by delivering DNA vaccines expressed in the phage genome. Antigens of interest can be displayed on phages, which trigger an antibody response upon administration, thereby generating immunity.193,194 Deng et al.195 fused an extracellular domain of matrix protein 2 to the N terminus (pVIII) of M13 phages. The recombinant phages were assessed in vivo for immunogenic potentials. A very significant protein 2 immune response was elicited, and the protein-phage was able to confer protection in mice infected by human and avian type A influenza viruses. Ghaemi et al.196 conducted a study on phage vaccination against cervical cancer. The gene coding for human papillomavirus 16 (HPV16) proteins E7 was incorporated into the Lambda ZAP CMV vector to give a ZAP HPV16 E7 bacteriophage complex. The immunogenicity of this complex was tested ex vivo on cells from immunized mice. There was an increased release of granzyme B, which is actively implicated in cytotoxicity and antiviral activities. Phage-based vaccination has also been applied to fungal infections. In Candida albicans, the secretory aspartyl proteinase (SAP) family proteins are known to possess virulence attributes. Filamentous fd phages displaying the epitope (the peptide Val-Lys-Tyr-Thr-Ser) from SAP2 were developed and established to produce a strong immune response in vivo. Mice infected with C. albicans and vaccinated using the fd phage-peptide dramatically increased survival compared to non-vaccinated mice.197 It was concluded that this phage-based vaccine showed promising potential and could be studied further in clinical trials.
Interestingly, it has been noted that phage-based vaccines play roles in disease prevention. Additionally, they have been studied for hormonal control. An example was cited where fertility could be controlled with gonadotropin-releasing hormone-based antigenic vaccines. It was proposed that this vaccine could inactivate endogenous gonadotropin-releasing hormones, thereby regulating their release and, by extension, fertility.198 Bacteriophage-particle vaccines have been developed for viral infections, such as Zika, influenza A, and Norwalk, and bacterial diseases, such as brucellosis, anthrax, and bubonic plague.104 Phage virus-like particles (VLPs) have also presented the human papillomavirus (HPV) L2 epitope, leading to the development of HPV-neutralizing antibodies.199 By conjugating a tandem HPV31/16L2 peptide (amino acid 17–31) onto the surface of bacteriophage MS2 virus-like particles (VLPs), immunized mice models released high antibodies against individual L2 epitopes and were protected from multiple HPV types.199
Some of the studies on the application of bacteriophages are summarized in Table 3.
Table 3 Summary of some studies on the applications of bacteriophages
Disease |
Type of therapy |
Bacteriophage |
Cargo |
Phage displays protein or targeting ligand |
Ref. |
Hepatocellular carcinoma |
Immunotherapy |
Lambda (λ) phage |
— |
Aspartate β-hydroxylase (ASPH) |
148
|
Breast cancer |
Immunotherapy |
M13 bacteriophages |
— |
Extracellular and transmembrane domains of human HER2 or its Δ16HER2 splice variant |
149
|
Chondrosarcoma |
Gene therapy |
M13 phage |
Tumour necrosis factor-alpha (TNFα) transgene cassette |
RGD4C |
152
|
Glioblastoma multiforme |
Gene and drug therapy |
M13 phage |
Adeno-associated virus genome, Grp 78, temozolomide) |
RGD4C |
153
|
Melanoma |
Gene therapy |
T7 bacteriophage |
Granulocyte macrophage-colony stimulating factor (GM-CSF |
Pep42 peptide |
154
|
Lung carcinoma and colon carcinoma |
Targeted therapy |
T4 phages |
— |
Vascular endothelial growth factor (VEGF) |
155
|
Hepatoma |
Gene therapy |
PP7 bacteriophage-based virus-like particles |
MicroRNAs |
Transactivated transcription (TAT) peptide |
156
|
Human papilloma virus (HPV) |
Gene therapy (gene transfer and expression) |
Lambda-ZAP bacteriophage |
Recombinant lambda-phage nanobioparticles |
Enhanced green fluorescent protein and E7 of HPV |
173
|
Hepatocellular carcinoma tumours |
Silencing RNA (siRNA) |
TMV phage |
TMV–TAT conjugation system |
Transacting activation transduction (TAT) |
176
|
Prostate cancer |
Deep-tissue diagnostic imaging |
M13 phages |
M13-SWCNT phage |
Single-walled carbon nanotubes (SWNTs) |
181
|
E. coli and S. aureus bacterial infections |
In vivo optical imaging |
M13 phages coat |
Biotinylated M13 phage |
Fluorescent dyes, biotin acceptor peptides |
182
|
Cancerous and metastatic cells |
Molecular imaging |
Engineered phage protein coat |
Engineered phage protein coat |
Green fluorescent protein (GFP) |
183 and 184
|
— |
Diagnosis |
— |
pVIII major coat protein |
Cyclic RGD (cRGD) |
185
|
Malignant triple negative MDA-MB-231 breast cancer cells |
Therapy and diagnosis |
Tumor-targeting bacteriophage |
— |
FAM–NHS dye |
186
|
Mycobacterium tuberculosis
|
Diagnosis |
T7 phage |
— |
Sarcoidosis antigens |
189
|
E. coli infection |
Detection of E. coli in Food-borne infection |
T7 phage |
Phage T7-ALP |
Alkaline phosphatase |
191
|
E. coli infection |
Detection and separation of E. coli in drinking water |
T7 phage |
T7 phage-conjugated to iron oxide magnetic beads |
— |
192
|
Human and avian type A influenza viruses |
Immunogenicity |
Recombinant M13 phages |
N terminus (pVIII) of M13 phages |
Protein 2 |
195
|
Cervical cancer |
Vaccination |
Lambda ZAP CMV |
ZAP HPV16 E7 bacteriophage complex |
Gene coding for human papillomavirus 16 (HPV16) proteins E7 |
196
|
Candida albicans infection |
Immune response |
Filamentous fd phages |
— |
Val-Lys-Tyr-Thr-Ser epitope from secretory aspartyl proteinase (SAP) |
197
|
Fertility control |
Hormonal control |
— |
— |
— |
198
|
Human papillomaviruses (HPVs) |
Immunization |
MS2 phage-like particles |
Phage virus-like particles (VLPs) |
Human papillomaviruses (HPVs) L2 epitope (HPV31/16L2 peptide) |
199
|
5. Challenges
Though inherently considered safe, there are often issues with safety considerations, depending on the phage type, preparation, and route of administration.200 These include the potential ability to trigger immune responses, neutralization and clearance, and chances of toxicity attributable to the phage component or loaded cargoes (e.g. phage-associated conversion of Tox− Streptococcus pyogenes into Tox+ bacteria in vivo, as described by Broudy et al.,201 especially in temperate phages202), unintended accumulation in non-target tissues, conferment of resistance genes to pathogenic bacterial strains, poor storage and stability profile, and unintended potential consequences of genetic engineering. Thus, safety evaluations are essential to harness their therapeutic effects safely and effectively in targeted drug delivery.
The regulatory challenges of using bacteriophages as nanocarriers in targeted drug delivery and therapeutic applications are complex and multifaceted but are centred on specific considerations, such as classification, economic viability and manufacturing standards, safety assessments, quality control, clinical trials, intellectual property, regulatory guidance, environmental impact, global harmonization, and post-approval monitoring. Long-term monitoring of safety and efficacy post-approval is essential, especially given the evolving nature of bacteriophage interactions and potential unforeseen effects. The regulation of phage therapy is growing, and addressing these regulatory concerns would require close collaboration among researchers, regulatory agencies, and other stakeholders.203
The other apparent challenges include the high selectivity and specificity of bacteriophages to limited species of host bacteria, thus limiting their applicability against a broad range of pathogens and diseases; rapid and over-amplification of therapeutic phages in vivo in the presence of their host bacteria, leading to unintended consequences; the potential development of resistance of target pathogen to bacteriophage formulations and undermining the long-term efficacy of the phage-based treatments; complexities associated with the delivery of phage formulations to target sites within the body owing to certain factors, including the pharmacokinetics and bioavailability; ethical concerns and public acceptance of genetically modified bacteriophages; limited data and clinical trials to validate research claims, effectiveness, long-term effects, and optimal usage; and the inadequate standardization of large scale phage formulation/preparations and production.204–207
6. Future perspectives and research directions
Surprisingly, low phage cytotoxicity studies have been published; thus, there is a need to advance this area.208 As Pirisi209 rightly summed: “What we think we know about phages has to be verified and then deemed reproducible, safe and effective”. Currently, most phage products are administered as suspensions or dressings (for skin infections), which are usually unsuitable for targeting specific target sites, as phages are rapidly cleared around these application areas.208 Pharmaceutical formulations must encapsulate and protect phages from the environment while preserving their biological activity, especially their lytic activity. Moreover, the excipients selected must be biocompatible and can release phages at the desired site of infection.208 The treatment outcomes of self-replicating pharmaceutical agents depend critically on various density-dependent thresholds, often with apparent paradoxical consequences; thus, the ability to predict these thresholds and associated critical time points becomes necessary for the clinical use of phage therapy.210 The exact modalities for using each phage product must be ascertained, including treatment regimens, route of administration, concentration and quantity, and methods.208 Finally, in the interim, the potential of phage therapy may be fulfilled using individual preparations on a named-patient basis, with extensive monitoring and multidisciplinary team input.202
7. Conclusion
The efficient delivery of therapeutic agents to target sites is a crucial problem and area in managing disease conditions. Nanotechnological advances utilising various nano-sized particles present promising platforms for this course. VLPs, specifically bacteriophages, with their desirable properties, including having potential therapeutic effects and serving as alternatives to antibiotics, also present the opportunity for use as effective nanocarriers in the delivery of drugs. Their use in drug delivery has many advantages, including biocompatibility, large loading capabilities, stability, evasion of immune responses, and easy modifiability. The various forms of the use of bacteriophages in this regard include their use in the capsid-associated display of peptides and antibodies, chemical conjugation of molecules, polymer coating of capsids, capsid manipulations and encapsulation, attachment of multi-targeting ligands and moieties, and image tagging. The bacteriophage-mediated drug delivery has many applications, such as in cancer therapies, bacterial infections and gene therapies. Although there are currently certain safety and regulatory challenges, the continuous research effects promise to open more doors increasingly.
Author contributions
S. C. E.: conceptualization, writing – original draft, writing – review & editing; A. L. O.: conceptualization, writing – original draft, writing – review & editing; C. F. K.: writing – original draft; P. N. E.: writing – original draft; O. C. E.: writing – original draft; M. M. D. C. V.: supervision, validation; V. M. B.: supervision, validation; A. A. A.: supervision, validation; E. B. O.: supervision, validation.
Conflicts of interest
There are no conflicts to declare.
References
- T. C. Ezike, U. S. Okpala, U. L. Onoja, C. P. Nwike, E. C. Ezeako and O. J. Okpara,
et al., Advances in drug delivery systems, challenges and future directions, Heliyon, 2023, 9(6), e17488 CrossRef CAS
.
- Z. Li, K. Xu, L. Qin, D. Zhao, N. Yang and D. Wang,
et al., Hollow Nanomaterials in Advanced Drug Delivery Systems: From Single- to Multiple Shells, Adv. Mater., 2023, 35(12), 2203890 CrossRef CAS
.
- S. Ranghar, P. Sirohi, P. Verma and V. Agarwal, Nanoparticle-based drug delivery systems: promising approaches against infections, Braz. Arch. Biol. Technol., 2014, 57, 209–222 CrossRef CAS
.
- J. K. Patra, G. Das, L. F. Fraceto, E. V. R. Campos, M. D. P. Rodriguez-Torres and L. S. Acosta-Torres,
et al., Nano based drug delivery systems: Recent developments and future prospects, J. Nanobiotechnol., 2018, 16(1), 1–33 CrossRef
.
- J. F. Coelho, P. C. Ferreira, P. Alves, R. Cordeiro, A. C. Fonseca and J. R. Góis,
et al., Drug delivery systems: Advanced technologies potentially applicable in personalized treatments, EPMA J., 2010, 1(1), 164–209 CrossRef
.
- A. Mansour, M. Romani, A. B. Acharya, B. Rahman, E. Verron and Z. Badran, Drug Delivery Systems in Regenerative Medicine: An Updated Review, Pharmaceutics, 2023, 15(2), 695 CrossRef CAS PubMed
.
- S. Adepu and S. Ramakrishna, Controlled drug delivery systems: Current status and future directions, Molecules, 2021, 26, 19 CrossRef
.
-
N. K. Shah, E. A. Torrico Guzmán, Z. Wang and S. A. Meenach, Routes of administration for nanocarriers, in Nanoparticles for Biomedical Applications, ed. E. J. Chung, L. Leon and C. Rinaldi, Micro and Nano Technologies, Elsevier, 2020, ch. 6, pp. 67–87, Available from: https://www.sciencedirect.com/science/article/pii/B9780128166628000060 [cited 2023 Aug 18] Search PubMed
.
- M. T. P. Paiva, J. O. F. Kishima, J. B. M. D. Silva, J. Mantovan, F. G. Colodi and S. Mali, Crosslinking Methods in Polysaccharide-Based Hydrogels for Drug Delivery Systems, Biomed. Mater. Devices, 2023, s44174-023-00118-4 Search PubMed
.
- A. K. Thakur, D. K. Chellappan, K. Dua, M. Mehta, S. Satija and I. Singh, Patented therapeutic drug delivery strategies for targeting pulmonary diseases, Expert Opin. Ther. Pat., 2020, 30(5), 375–387 CrossRef CAS
.
- R. Lombardo, T. Musumeci, C. Carbone and R. Pignatello, Nanotechnologies for intranasal drug delivery: an update of literature, Pharm. Dev. Technol., 2021, 26(8), 824–845 CrossRef CAS
.
- S. Mahant, A. K. Sharma, H. Gandhi, R. Wadhwa, K. Dua and D. N. Kapoor, Emerging Trends and Potential Prospects in Vaginal Drug Delivery, Curr. Drug Delivery, 2023, 20(6), 730–751 CrossRef CAS
.
- G. Tiwari, R. Tiwari, B. Sriwastawa, L. Bhati, S. Pandey and P. Pandey,
et al., Drug delivery systems: An updated review, Int. J. Pharm. Investig., 2012, 2(1), 2–11 CrossRef
.
- G. Mehta, A. Y. Hsiao, M. Ingram, G. D. Luker and S. Takayama, Opportunities and challenges for use of tumor spheroids as models to test drug delivery and efficacy, J. Controlled Release, 2012, 164(2), 192–204 CrossRef CAS
.
- D. Chenthamara, S. Subramaniam, S. G. Ramakrishnan, S. Krishnaswamy, M. M. Essa and F. H. Lin,
et al., Therapeutic efficacy of nanoparticles and routes of administration, Biomater. Res., 2019, 23(1), 20 CrossRef CAS PubMed
.
- H. Wen, H. Jung and X. Li, Drug Delivery Approaches in Addressing Clinical Pharmacology-Related Issues: Opportunities and Challenges, AAPS J., 2015, 17(6), 1327–1340 CrossRef CAS
.
- C. S. Jun, L. Z. Qiang, S. Guo, J. G. Ping and L. H. Ling, An update – Prolonging the action of protein and peptide drugs, J. Drug Delivery Sci. Technol., 2021, 61, 102124 CrossRef
.
- F. U. Din, W. Aman, I. Ullah, O. S. Qureshi, O. Mustapha and S. Shafique,
et al., Effective use of nanocarriers as drug delivery systems for the treatment of selected tumors, Int. J. Nanomed., 2017, 12, 7291–7309 CrossRef
.
- B. Yuan, Y. Liu, M. Lv, Y. Sui, S. Hou and T. Yang,
et al., Virus-like particle-based nanocarriers as an emerging platform for drug delivery, J. Drug Target., 2023, 31(5), 433–455 CrossRef CAS
.
- J. J. Barr, A bacteriophages journey through the human body, Immunol. Rev., 2017, 279(1), 106–122 CrossRef CAS
.
- M. Karimi, H. Mirshekari, M. MoosaviBasri, S. Bahrami, M. Moghoofei and M. R. Hamblin, Bacteriophages and Phage-Inspired Nanocarriers for Targeted Delivery of Therapeutic Cargos, Adv. Drug Delivery Rev., 2016, 106(Pt A), 45–62 CrossRef CAS PubMed
.
- S. C. Emencheta, A. A. Attama, E. N. Ezeibe, A. A. Agubata and E. B. Onuigbo, Isolation of Escherichia Coli Phages from Waste Waters, Hosts Viruses, 2022, 9(2), 12–18 Search PubMed
, Available from: https://researcherslinks.com/current-issues/Isolation-Escherichia-Coli-Phages-from-Waste-Waters/6/1/4940/html.
- S. C. Emencheta, C. C. Eze, A. A. Attama, D. E. Agbo and E. B. Onuigbo, Isolation of Pseudomonas aeruginosa Phages from Wastewaters, Hosts Viruses, 2021, 8(6), 1–6 CAS
, Available from: https://researcherslinks.com/current-issues/Isolation-Pseudomonas-aeruginosa-Phages-from-Wastewaters/6/1/4548/html.
- S. Meile, J. Du, M. Dunne, S. Kilcher and M. J. Loessner, Engineering therapeutic phages for enhanced antibacterial efficacy, Curr. Opin. Virol., 2022, 52, 182–191 CrossRef CAS PubMed
.
- S. C. Emencheta, C. V. Olovo, O. C. Eze, C. F. Kalu, D. P. Berebon and E. B. Onuigbo,
et al., The Role of Bacteriophages in the Gut Microbiota: Implications for Human Health, Pharmaceutics, 2023, 15(10), 2416 CrossRef CAS
.
- N. Suthiwangcharoen, T. Li, K. Li, P. Thompson, S. You and Q. Wang, M13 bacteriophage-polymer nanoassemblies as drug delivery vehicles, Nano Res., 2011, 4(5), 483–493 CrossRef CAS
.
- P. Rogovski, R. D. Cadamuro, R. Da Silva, E. B. De Souza, C. Bonatto and A. Viancelli,
et al., Uses of Bacteriophages as Bacterial Control Tools and Environmental Safety Indicators, Front. Microbiol., 2021, 12, 793135 CrossRef PubMed
.
- V. Foglizzo and S. Marchiò, Bacteriophages as Therapeutic and Diagnostic Vehicles in Cancer, Pharmaceuticals, 2021, 14(2), 161 CrossRef CAS PubMed
.
-
S. Bhattacharya, P. Rodriques and B. Prajapati, Introductory Chapter: Advanced Drug Delivery Systems, Advanced Drug Delivery Systems, IntechOpen, 2023 Search PubMed
.
- K. Radhakrishnan, J. Tripathy, D. P. Gnanadhas, D. Chakravortty and A. M. Raichur, Dual enzyme responsive and targeted nanocapsules for intracellular delivery of anticancer agents, RSC Adv., 2014, 4(86), 45961–45968 RSC
.
- D. Liu, F. Yang, F. Xiong and N. Gu, The smart drug delivery system and its clinical potential, Theranostics, 2016, 6(9), 1306–1323 CrossRef CAS PubMed
.
- Z. Chai, D. Ran, L. Lu, C. Zhan, H. Ruan and X. Hu, Ligand-Modified Cell Membrane Enables the Targeted Delivery of Drug Nanocrystals to Glioma, ACS Nano, 2019, 13, 5591–5601 CrossRef CAS PubMed
.
- R. H. H. Neubert, Potentials of new nanocarriers for dermal and transdermal drug delivery, Eur. J. Pharm. Biopharm., 2011, 77(1), 1–2 CrossRef CAS PubMed
.
- A. L. Onugwu, C. S. Nwagwu, O. S. Onugwu, A. C. Echezona, C. P. Agbo and S. A. Ihim,
et al., Nanotechnology based drug delivery systems for the treatment of anterior segment eye diseases, J. Controlled Release, 2023, 354, 465–488 CrossRef CAS
.
- B. Mishra, B. B. Patel and S. Tiwari, Colloidal nanocarriers: a review on formulation technology, types and applications toward targeted drug delivery, Nanomedicine, 2010, 6(1), 9–24 CrossRef CAS
.
- A. L. Onugwu, O. L. Ugorji, C. A. Ufondu, S. A. Ihim, A. C. Echezona and C. S. Nwagwu,
et al., Nanoparticle-based delivery systems as emerging therapy in retinoblastoma: recent advances, challenges and prospects, Nanoscale Adv., 2023, 5(18), 4628–4648 RSC
.
- S. Hossen, M. K. Hossain, M. K. Basher, M. N. H. Mia, M. T. Rahman and M. J. Uddin, Smart nanocarrier-based drug delivery systems for cancer therapy and toxicity studies: A review, J. Adv. Res., 2019, 15, 1–18 CrossRef CAS PubMed
.
- V. Gote, M. Ansong and D. Pal, Prodrugs and nanomicelles to overcome ocular barriers for drug penetration, Expert Opin. Drug Metab. Toxicol., 2020, 16(10), 885–906 CrossRef CAS PubMed
.
- R. Trivedi and U. B. Kompella, Nanomicellar formulations for sustained drug delivery: strategies and underlying principles, Nanomedicine, 2010, 5(3), 485–505 CrossRef CAS
.
- W. Xu, P. Ling and T. Zhang, Polymeric Micelles, a Promising Drug Delivery System to Enhance Bioavailability of Poorly Water-Soluble Drugs, J. Drug Delivery, 2013, 2013, 1–15 CrossRef PubMed
.
- C. O. Rangel-Yagui, A. Pessoa and L. C. Tavares, Micellar solubilization of drugs, J. Pharm. Pharm. Sci., 2005, 8(2), 147–165 CAS
.
- P. Ghosh and R. Murthy, Microemulsions: A Potential Drug Delivery System, Curr. Drug Delivery, 2006, 3(2), 167–180 CrossRef CAS PubMed
.
- N. Üstündağ Okur, E. Ş. Çağlar, M. D. Arpa and H. Y. Karasulu, Preparation and evaluation of novel microemulsion-based hydrogels for dermal delivery of benzocaine, Pharm. Dev. Technol., 2017, 22(4), 500–510 CrossRef
.
- S. Gorantla, V. K. Rapalli, T. Waghule, P. P. Singh, S. K. Dubey and R. N. Saha,
et al., Nanocarriers for ocular drug delivery: current status and translational opportunity, RSC Adv., 2020, 10(46), 27835–27855 RSC
.
- A. L. Onugwu, A. A. Attama, P. O. Nnamani, S. O. Onugwu, E. B. Onuigbo and V. V. Khutoryanskiy, Development and optimization of solid lipid nanoparticles coated with chitosan and poly(2-ethyl-2-oxazoline) for ocular drug delivery of ciprofloxacin, J. Drug Delivery Sci. Technol., 2022, 74, 103527 CrossRef CAS
.
- S. Li, L. Chen and Y. Fu, Nanotechnology-based ocular drug delivery systems: recent advances and future prospects, J. Nanobiotechnol., 2023, 21(1), 232 CrossRef
.
- I. Chauhan, M. Yasir, M. Verma and A. P. Singh, Nanostructured Lipid Carriers: A Groundbreaking Approach for Transdermal Drug Delivery, Adv. Pharm. Bull., 2020, 10(2), 150–165 CrossRef CAS
.
-
M. N. Sardoiwala, B. Kaundal and S. Roy Choudhury, Development of Engineered Nanoparticles Expediting Diagnostic and Therapeutic Applications Across Blood–Brain Barrier, Handbook of Nanomaterials for Industrial Applications, Elsevier, 2018, pp. 696–709 Search PubMed
.
- S. Shah, P. Famta, R. S. Raghuvanshi, S. B. Singh and S. Srivastava, Lipid polymer hybrid nanocarriers: Insights into synthesis aspects, characterization, release mechanisms, surface functionalization and potential implications, Colloid Interface Sci Commun., 2022, 46, 100570 CrossRef CAS
.
- A. L. Onugwu, C. S. Nwagwu, O. S. Onugwu, A. C. Echezona, C. P. Agbo and S. A. Ihim,
et al., Nanotechnology based drug delivery systems for the treatment of anterior segment eye diseases, J. Controlled Release, 2023, 354, 465–488 CrossRef CAS PubMed
.
- A. Akbarzadeh, R. Rezaei-Sadabady, S. Davaran, S. W. Joo, N. Zarghami and Y. Hanifehpour,
et al., Liposome: classification, preparation, and applications, Nanoscale Res. Lett., 2013, 8(1), 102 CrossRef PubMed
.
- V. V. S. N. L. Andra, S. V. N. Pammi, L. V. K. P. Bhatraju and L. K. Ruddaraju, A Comprehensive Review on Novel Liposomal Methodologies, Commercial Formulations, Clinical Trials and Patents, Bionanoscience, 2022, 12(1), 274–291 CrossRef
.
- P. Aparajay and A. Dev, Functionalized niosomes as a smart delivery device in cancer and fungal infection, Eur. J. Pharm. Sci., 2022, 168, 106052 CrossRef CAS
.
- A. Verma, A. Jain, A. Tiwari, S. Saraf, P. K. Panda and S. K. Jain, Promising Antifungal Potential of Engineered Non-ionic Surfactant-Based Vesicles: In Vitro and In Vivo Studies, AAPS PharmSciTech, 2021, 22(1), 19 CrossRef CAS PubMed
.
- A. Chauhan, Dendrimers for Drug Delivery, Molecules, 2018, 23(4), 938 CrossRef PubMed
.
-
V. Patel, C. Rajani, D. Paul, P. Borisa, K. Rajpoot, S. R. Youngren-Ortiz, et al., Dendrimers as novel drug-delivery system and its applications, Drug Delivery Systems, Elsevier, 2020, pp. 333–392 Search PubMed
.
- V. Rastogi, P. Yadav, M. Porwal, S. Sur and A. Verma, Dendrimer as nanocarrier for drug delivery and drug targeting therapeutics: a fundamental to advanced systematic review, Int. J. Polym. Mater. Polym. Biomater., 2022, 1–23 Search PubMed
.
- H. Chen, L. Wang, X. Zeng, H. Schwarz, H. S. Nanda and X. Peng,
et al., Exosomes, a New Star for Targeted Delivery, Front. Cell Dev. Biol., 2021, 9, 751079 CrossRef
.
- G. M. Ngandeu Neubi, Y. Opoku-Damoah, X. Gu, Y. Han, J. Zhou and Y. Ding, Bio-inspired drug delivery systems: an emerging platform for targeted cancer therapy, Biomater. Sci., 2018, 6(5), 958–973 RSC
.
- Y. Zhang, Y. Liu, H. Liu and W. H. Tang, Exosomes: biogenesis, biologic function and clinical potential, Cell Biosci., 2019, 9(1), 19 CrossRef PubMed
.
- S. Nooraei, H. Bahrulolum, Z. S. Hoseini, C. Katalani, A. Hajizade and A. J. Easton,
et al., Virus-like particles: preparation, immunogenicity and their roles as nanovaccines and drug nanocarriers, J. Nanobiotechnol., 2021, 19(1), 59 CrossRef CAS
.
- Y. H. Chung, H. Cai and N. F. Steinmetz, Viral nanoparticles for drug delivery, imaging, immunotherapy, and theranostic applications, Adv. Drug Delivery Rev., 2020, 156, 214–235 CrossRef CAS
.
- N. M. Mansour, Bacteriophages are natural gift, could we pay further attention!, J. Food Microbiol., 2018, 2(1), 1–3 Search PubMed
, Available from: https://www.alliedacademies.org/abstract/bacteriophages-are-natural-gift-could-we-pay-further-attention-9734.html.
-
K. D. Weynberg, Chapter One - Viruses in Marine Ecosystems: From Open Waters to Coral Reefs, in Advances in Virus Research, ed. C. M. Malmstrom, Academic Press, 2018, Environmental Virology and Virus Ecology, vol. 101, pp. 1–38, Available from: https://www.sciencedirect.com/science/article/pii/S0065352718300046, [cited 2023 Nov 18] Search PubMed
.
-
Family – Tectiviridae, in Virus Taxonomy, ed. A. M. Q. King, M. J. Adams, E. B. Carstens and E. J. Lefkowitz, Elsevier, San Diego, 2012, pp. 317–321. Available from: https://www.sciencedirect.com/science/article/pii/B9780123846846000306, [cited 2023 Jun 12] Search PubMed
.
-
Family – Corticoviridae, in Virus Taxonomy, ed. A. M. Q. King, M. J. Adams, E. B. Carstens and E. J. Lefkowitz, Elsevier, San Diego, 2012, pp. 179–182. Available from: https://www.sciencedirect.com/science/article/pii/B978012384684600015X, [cited 2023 Nov 18] Search PubMed
.
- H. M. Oksanen, ICTV Report Consortium. ICTV Virus Taxonomy Profile: Corticoviridae, J. Gen. Virol., 2017, 98(5), 888–889 CrossRef CAS PubMed
.
-
Family – Lipothrixviridae, in Virus Taxonomy, ed. A. M. Q. King, M. J. Adams, E. B. Carstens and E. J. Lefkowitz, Elsevier, San Diego, 2012, pp. 211–221. Available from: https://www.sciencedirect.com/science/article/pii/B9780123846846000203, [cited 2023 Nov 18] Search PubMed
.
- M. Krupovic, Ictv Report Consortium null. ICTV Virus Taxonomy Profile: Plasmaviridae, J. Gen. Virol., 2018, 99(5), 617–618 CrossRef CAS PubMed
.
- D. Prangishvili, H. P. Arnold, D. Götz, U. Ziese, I. Holz and J. K. Kristjansson,
et al., A novel virus family, the Rudiviridae: Structure, virus-host interactions and genome variability of the sulfolobus viruses SIRV1 and SIRV2, Genetics, 1999, 152(4), 1387–1396 CrossRef CAS
.
-
Family – Fuselloviridae, in Virus Taxonomy, ed. A. M. Q. King, M. J. Adams, E. B. Carstens and E. J. Lefkowitz, Elsevier, San Diego, 2012, pp. 183–186. Available from: https://www.sciencedirect.com/science/article/pii/B9780123846846000161, [cited 2023 Nov 18] Search PubMed
.
-
Family – Inoviridae, in Virus Taxonomy, ed. A. M. Q. King, M. J. Adams, E. B. Carstens and E. J. Lefkowitz, Elsevier, San Diego, 2012, pp. 375–383. Available from: https://www.sciencedirect.com/science/article/pii/B9780123846846000367, [cited 2023 Nov 18] Search PubMed
.
-
Family – Microviridae, in Virus Taxonomy, ed. A. M. Q. King, M. J. Adams, E. B. Carstens and E. J. Lefkowitz, Elsevier, San Diego, 2012, pp. 385–393. Available from: https://www.sciencedirect.com/science/article/pii/B9780123846846000379, [cited 2023 Jun 12] Search PubMed
.
-
Family – Leviviridae, in Virus Taxonomy, ed. A. M. Q. King, M. J. Adams, E. B. Carstens and E. J. Lefkowitz, Elsevier, San Diego, 2012, pp. 1035–1043. Available from: https://www.sciencedirect.com/science/article/pii/B9780123846846000896, [cited 2023 Jun 12] Search PubMed
.
-
Family – Cystoviridae, in Virus Taxonomy, ed. A. M. Q. King, M. J. Adams, E. B. Carstens and E. J. Lefkowitz, Elsevier, San Diego, 2012, pp. 515–518. Available from: https://www.sciencedirect.com/science/article/pii/B9780123846846000471, [cited 2023 Nov 18] Search PubMed
.
- S. Matsuzaki, M. Rashel, J. Uchiyama, S. Sakurai, T. Ujihara and M. Kuroda,
et al., Bacteriophage therapy: a revitalized therapy against bacterial infectious diseases, J. Infect. Chemother., 2005, 11(5), 211–219 CrossRef
.
-
M. T. Madigan, K. S. Bender, D. H. Buckley, W. M. Sattley and D. A. Stahl, Brock biology of microorganisms, Pearson, NY, NY, 15th edn, 2018, p. 1058 Search PubMed
.
- V. B. Rao and J. Zhu, Bacteriophage T4 as a nanovehicle for delivery of genes and therapeutics into human cells, Curr. Opin. Virol., 2022, 55, 101255 CrossRef CAS
.
- H. M. E. Duyvesteyn, I. Santos-Pérez, F. Peccati, A. Martinez-Castillo, T. S. Walter and D. Reguera,
et al., Bacteriophage PRD1 as a nanoscaffold for drug loading, Nanoscale, 2021, 13(47), 19875–19883 RSC
.
- G. Chauhan, M. J. Madou, S. Kalra, V. Chopra, D. Ghosh and S. O. Martinez-Chapa, Nanotechnology for COVID-19: Therapeutics and Vaccine Research, ACS Nano, 2020, 14(7), 7760–7782 CrossRef CAS PubMed
.
- S. Shukla, H. Hu, H. Cai, S. K. Chan, C. E. Boone and V. Beiss,
et al., Plant Viruses and Bacteriophage-Based Reagents for Diagnosis and Therapy, Annu. Rev. Virol., 2020, 7(1), 559–587 CrossRef CAS PubMed
.
- K. Khambhati, G. Bhattacharjee, N. Gohil, G. K. Dhanoa, A. P. Sagona and I. Mani,
et al., Phage engineering and phage-assisted CRISPR-Cas delivery to combat multidrug-resistant pathogens, Bioeng. Transl. Med., 2023, 8(2), e10381 CrossRef CAS
.
- J. Zhou, N. Krishnan, Y. Jiang, R. H. Fang and L. Zhang, Nanotechnology for virus treatment, Nano Today, 2021, 36, 101031 CrossRef CAS
.
- J. Hopf, M. Waters, V. Kalwajtys, K. E. Carothers, R. K. Roeder and J. D. Shrout,
et al., Phage-mimicking antibacterial core–shell nanoparticles, Nanoscale Adv., 2019, 1(12), 4812–4826 RSC
.
- H. E. van Kan-Davelaar, J. C. M. van Hest, J. J. L. M. Cornelissen and M. S. T. Koay, Using viruses as nanomedicines, Br. J. Pharmacol., 2014, 171(17), 4001–4009 CrossRef CAS PubMed
.
- N. F. Steinmetz, Viral nanoparticles as platforms for next-generation therapeutics and imaging devices, Nanomedicine, 2010, 6(5), 634–641 CrossRef CAS PubMed
.
- S. Raza, K. Matuła, S. Karoń and J. Paczesny, Resistance and Adaptation of Bacteria to Non-Antibiotic Antibacterial Agents: Physical Stressors, Nanoparticles, and Bacteriophages, Antibiotics, 2021, 10(4), 435 CrossRef CAS
.
- F. Gomes and M. Henriques, Control of Bovine Mastitis: Old and Recent Therapeutic Approaches, Curr. Microbiol., 2016, 72(4), 377–382 CrossRef CAS
.
- A. T. Yayehrad, E. A. Siraj, G. B. Wondie, A. A. Alemie, M. T. Derseh and A. S. Ambaye, Could Nanotechnology Help to End the Fight Against COVID-19? Review of Current Findings, Challenges and Future Perspectives, Int. J. Nanomed., 2021, 16, 5713–5743 CrossRef PubMed
.
- J. Lu, J. Wang and D. Ling, Surface Engineering of Nanoparticles for Targeted Delivery to Hepatocellular Carcinoma, Small, 2018, 14(5), 1702037 CrossRef
.
- L. Zhao, M. Kopylov, C. S. Potter, B. Carragher and M. G. Finn, Engineering the PP7 Virus Capsid as a Peptide Display Platform, ACS Nano, 2019, 13(4), 4443–4454 CrossRef CAS
.
- I. Yacoby, H. Bar and I. Benhar, Targeted drug-carrying bacteriophages as antibacterial nanomedicines, Antimicrob. Agents Chemother., 2007, 51(6), 2156–2163 CrossRef CAS
.
- K. P. Kim, J. D. Cha, E. H. Jang, J. Klumpp, S. Hagens and W. D. Hardt,
et al., PEGylation of bacteriophages increases blood circulation time and reduces T-helper type 1 immune response, Microb. Biotechnol., 2008, 1(3), 247–257 CrossRef CAS
.
- A. E. Czapar and N. F. Steinmetz, Plant viruses and bacteriophages for drug delivery in medicine and biotechnology, Curr. Opin. Chem. Biol., 2017, 38, 108–116 CrossRef CAS
.
- C. E. Ashley, E. C. Carnes, G. K. Phillips, P. N. Durfee, M. D. Buley and C. A. Lino,
et al., Cell-Specific Delivery of Diverse Cargos by Bacteriophage MS2 Virus-like Particles, ACS Nano, 2011, 5(7), 5729–5745 CrossRef CAS
.
- X. Wang, S. Li, Y. Shi, X. Chuan, J. Li and T. Zhong,
et al., The development of site-specific drug delivery nanocarriers based on receptor mediation, J. Controlled Release, 2014, 193, 139–153 CrossRef CAS PubMed
.
- V. A. Petrenko and J. W. Gillespie, Paradigm shift in bacteriophage-mediated delivery of anticancer drugs: from targeted ‘magic bullets’ to self-navigated ‘magic missiles’, Expert Opin. Drug Delivery, 2017, 14(3), 373–384 CrossRef CAS PubMed
.
- M. Mahler, A. R. Costa, S. P. B. Beljouw, P. C. van, Fineran and S. J. J. Brouns, Approaches for bacteriophage genome engineering, Trends Biotechnol., 2023, 41(5), 669–685 CrossRef CAS PubMed
.
- I. Gallego, I. Lostalé-Seijo and J. Montenegro, Stronger Together: Multivalent Phage Capsids Inhibit Virus Entry, ChemBioChem, 2021, 22(3), 478–480 CrossRef CAS PubMed
.
-
J. K. Rhee, M. Hovlid, J. D. Fiedler, S. D. Brown, F. Manzenrieder, H. Kitagishi, et al., Colorful Virus-like Particles: Fluorescent Protein Packaging by the Qβ Capsid, ACS Publications, American Chemical Society, 2011 DOI:10.1021/bm200983k, [cited 2023 Aug 10]
.
- C. P. Goodall, B. Schwarz, E. Selivanovitch, J. Avera, J. Wang, H. Miettinen and T. Douglas, Controlled Modular Multivalent Presentation of the CD40 Ligand on P22 Virus-like Particles Leads to Tunable Amplification of CD40 Signaling, ACS Appl. Bio Mater., 2021, 4, 8205–8214 CrossRef CAS
.
- D. S. Peabody, B. Manifold-Wheeler, A. Medford, S. K. Jordan, J. do Carmo Caldeira and B. Chackerian, Immunogenic Display of Diverse Peptides on Virus-like Particles of RNA Phage MS2, J. Mol. Biol., 2008, 380(1), 252–263 CrossRef CAS PubMed
.
- S. B. Shivachandra, Q. Li, K. K. Peachman, G. R. Matyas, S. H. Leppla and C. R. Alving,
et al., Multicomponent anthrax toxin display and delivery using bacteriophage T4, Vaccine, 2007, 25(7), 1225–1235 CrossRef CAS
.
- P. Tao, M. Mahalingam, J. Zhu, M. Moayeri, J. Sha, W. S. Lawrence, S. H. Leppla, A. K. Chopra and V. B. Rao, A Bacteriophage T4 Nanoparticle-Based Dual Vaccine against Anthrax and Plague, mBio, 2018, 9(5), e01926-18 CrossRef
.
- Q. Li, S. B. Shivachandra, Z. Zhang and V. B. Rao, Assembly of the Small Outer Capsid Protein, Soc, on Bacteriophage T4: A Novel System for High Density Display of Multiple Large Anthrax Toxins and Foreign Proteins on Phage Capsid, J. Mol. Biol., 2007, 370(5), 1006–1019 CrossRef CAS
.
- T. Sathaliyawala, M. Rao, D. M. Maclean, D. L. Birx, C. R. Alving and V. B. Rao, Assembly of Human Immunodeficiency Virus (HIV) Antigens on Bacteriophage T4: a Novel In Vitro Approach To Construct Multicomponent HIV Vaccines, J. Virol., 2006, 80(15), 7688–7698 CrossRef CAS PubMed
.
-
T. L. Schlick, Z. Ding, E. W. Kovacs and M. B. Francis, Dual-Surface Modification of the Tobacco Mosaic Virus, ACS Publications. American Chemical Society, 2005 DOI:10.1021/ja046239n, [cited 2023 Aug 15]
.
- B. Bortot, M. Apollonio, G. Baj, L. Andolfi, L. Zupin and S. Crovella,
et al., Advanced photodynamic therapy with an engineered M13 phage targeting EGFR: Mitochondrial localization and autophagy induction in ovarian cancer cell lines, Free Radic. Biol. Med., 2022, 179, 242–251 CrossRef CAS PubMed
.
- S. Kaur, K. Harjai and S. Chhibber,
In Vivo Assessment of Phage and Linezolid Based Implant Coatings for Treatment of Methicillin Resistant S. aureus (MRSA) Mediated Orthopaedic Device Related Infections, PLoS One, 2016, 11(6), e0157626 CrossRef
.
- J. Leal, X. Peng, X. Liu, D. Arasappan, D. C. Wylie and S. H. Schwartz,
et al., Peptides as surface coatings of nanoparticles that penetrate human cystic fibrosis sputum and uniformly distribute in vivo following pulmonary delivery, J. Controlled Release, 2020, 322, 457–469 CrossRef CAS PubMed
.
- E. W. Kovacs, J. M. Hooker, D. W. Romanini, P. G. Holder, K. E. Berry and M. B. Francis, Dual-Surface-Modified Bacteriophage MS2 as an Ideal Scaffold for a Viral Capsid-Based Drug Delivery System, Bioconjug. Chem., 2007, 18(4), 1140–1147 CrossRef CAS
.
-
P. Serwer, E. T. Wright, C. B. Gonzales, P. Serwer, E. T. Wright and C. B. Gonzales, Phage Capsids as Gated, Long-Persistence, Uniform Drug Delivery Vehicles, Current and Future Aspects of Nanomedicine, IntechOpen, 2020, Available from: https://www.intechopen.com/chapters/70945, [cited 2023 Aug 18] Search PubMed
.
- P. Serwer and E. T. Wright, Gated Ethidium- and Bleomycin-Loading in Phage T4 That Is Subsequently Purified Leak-Free, Biophysica, 2022, 2(4), 366–380 CrossRef
.
-
V. Petrenko and V. Torchilin, Precise Phage-navigating Breast Cancer-targeted Paclitaxel-Micell Nanomedicines, 2018 Search PubMed
.
- M. Loi, D. Di Paolo, M. Soster, C. Brignole, A. Bartolini and L. Emionite,
et al., Novel phage display-derived neuroblastoma-targeting peptides potentiate the effect of drug nanocarriers in preclinical settings, J. Controlled Release, 2013, 170(2), 233–241 CrossRef CAS
.
- K. Li, Y. Chen, S. Li, H. G. Nguyen, Z. Niu and S. You,
et al., Chemical Modification of M13 Bacteriophage and Its Application in Cancer Cell Imaging, Bioconjug. Chem., 2010, 21(7), 1369–1377 CrossRef CAS
.
- Z. M. Carrico, M. E. Farkas, Y. Zhou, S. C. Hsiao, J. D. Marks and H. Chokhawala,
et al., N-Terminal Labeling of Filamentous Phage To Create Cancer Marker Imaging Agents, ACS Nano, 2012, 6(8), 6675–6680 CrossRef CAS
.
- L. M. De Plano, D. Franco, M. Bonsignore, E. Fazio, S. Trusso and A. Allegra,
et al., Phage-Phenotype Imaging of Myeloma Plasma Cells by Phage Display, Appl. Sci., 2021, 11(17), 7910 CrossRef CAS
.
- A. L. Bryson, Y. Hwang, S. Sherrill-Mix, G. D. Wu, J. D. Lewis, L. Black, T. A. Clark and F. D. Bushman, Covalent Modification of Bacteriophage T4 DNA Inhibits CRISPR-Cas9, mBio, 2015, 6(3), e00648 CrossRef CAS PubMed
.
- P. Tao, X. Wu, W. C. Tang, J. Zhu and V. Rao, Engineering of Bacteriophage T4 Genome Using CRISPR-Cas9, ACS Synth. Biol., 2017, 6(10), 1952–1961 CrossRef
.
- C. Kimmelshue, A. S. Goggi and R. Cademartiri, The use of biological seed coatings based on bacteriophages and polymers against Clavibacter michiganensis subsp. nebraskensis in maize seeds, Sci. Rep., 2019, 9(1), 17950 CrossRef PubMed
.
- Z. Edis, J. Wang, M. K. Waqas, M. Ijaz and M. Ijaz, Nanocarriers-Mediated Drug Delivery Systems for Anticancer Agents: An Overview and Perspectives, Int. J. Nanomed., 2021, 16, 1313 CrossRef
.
- S. Chowdhury, F. Yusof, W. W. A. W. Salim, N. Sulaiman and M. O. Faruck, An overview of drug delivery vehicles for cancer treatment: Nanocarriers and nanoparticles including photovoltaic nanoparticles, J. Photochem. Photobiol., B, 2016, 164, 151–159 CrossRef CAS
.
- P. Trucillo, Drug Carriers: Classification, Administration, Release Profiles, and Industrial Approach, Processes, 2021, 9(3), 470 CrossRef CAS
.
- A. M. Wen, P. H. Rambhia, R. H. French and N. F. Steinmetz, Design rules for nanomedical engineering: from physical virology to the applications of virus-based materials in medicine, J. Biol. Phys., 2013, 39(2), 301–325 CrossRef CAS PubMed
.
- I. Gierlicka, S. I. S. Rattan and M. Wnuk, Perspectives on using bacteriophages in biogerontology research and interventions, Chem. – Biol. Interact., 2022, 366, 110098 CrossRef CAS PubMed
.
- S. N. Crooke, J. Schimer, I. Raji, B. Wu, A. K. Oyelere and M. G. Finn, Lung Tissue Delivery of Virus-Like Particles Mediated by Macrolide Antibiotics, Mol. Pharmaceutics, 2019, 16(7), 2947–2955 CrossRef CAS
.
- N. Stephanopoulos, G. J. Tong, S. C. Hsiao and M. B. Francis, Dual-surface modified virus capsids for targeted delivery of photodynamic agents to cancer cells, ACS Nano, 2010, 4(10), 6014–6020 CrossRef CAS
.
- S. Kaur, A. Kumari, A. Kumari Negi, V. Galav, S. Thakur and M. Agrawal,
et al., Nanotechnology Based Approaches in Phage Therapy: Overcoming the Pharmacological Barriers, Front. Pharmacol., 2021, 12, 699054 CrossRef CAS
.
- T. Wang, W. C. Hartner, J. W. Gillespie, K. P. Praveen, S. Yang and L. A. Mei,
et al., Enhanced tumor delivery and antitumor activity in vivo of liposomal doxorubicin modified with MCF-7-specific phage fusion protein, Nanomedicine, 2014, 10(2), 421–430 CrossRef CAS PubMed
.
- L. Gan, P. Fagerholm and J. Palmblad, Vascular endothelial growth factor (VEGF) and its receptor VEGFR-2 in the regulation of corneal neovascularization and wound healing, Acta Ophthalmol. Scand., 2004, 82(5), 557–563 CrossRef CAS PubMed
.
- R. Farr, D. S. Choi and S. W. Lee, Phage-based nanomaterials for biomedical applications, Acta Biomater., 2014, 10(4), 1741–1750 CrossRef CAS
.
- M. Zahid, B. E. Phillips, S. M. Albers, N. Giannoukakis, S. C. Watkins and P. D. Robbins, Identification of a Cardiac Specific Protein Transduction Domain by In Vivo Biopanning Using a M13 Phage Peptide
Display Library in Mice, PLoS One, 2010, 5(8), e12252 CrossRef
.
- Y. Ma, R. J. M. Nolte and J. J. L. M. Cornelissen, Virus-based nanocarriers for drug delivery, Adv. Drug Delivery Rev., 2012, 64(9), 811–825 CrossRef CAS PubMed
.
- A. Ksendzovsky, S. Walbridge, R. C. Saunders, A. R. Asthagiri, J. D. Heiss and R. R. Lonser, Convection-enhanced delivery of M13 bacteriophage to the brain, J. Neurosurg., 2012, 117(2), 197–203 CAS
.
- I. S. Rakover, N. Zabavnik, R. Kopel, M. Paz-Rozner and B. Solomon, Antigen-specific therapy of EAE via intranasal delivery of filamentous phage displaying a myelin immunodominant epitope, J. Neuroimmunol., 2010, 225(1–2), 68–76 CrossRef CAS
.
- C. I. Colino, J. M. Lanao and C. Gutierrez-Millan, Targeting of Hepatic Macrophages by Therapeutic Nanoparticles, Front. Immunol., 2020, 11, 218 CrossRef CAS
.
- S. C. Wong, D. Wakefield, J. Klein, S. D. Monahan, D. B. Rozema and D. L. Lewis,
et al., Hepatocyte targeting of nucleic acid complexes and liposomes by a T7 phage p17 peptide, Mol. Pharmaceutics, 2006, 3(4), 386–397 CrossRef CAS
.
- H. Bar, I. Yacoby and I. Benhar, Killing cancer cells by targeted drug-carrying phage nanomedicines, BMC Biotechnol., 2008, 8(1), 37 CrossRef PubMed
.
- L. Vaks and I. Benhar,
In vivo characteristics of targeted drug-carrying filamentous bacteriophage nanomedicines, J. Nanobiotechnol., 2011, 9, 58 CrossRef CAS
.
- S. Scibilia, G. Lentini, E. Fazio, D. Franco, F. Neri and A. M. Mezzasalma,
et al., Self-assembly of silver nanoparticles and bacteriophage, Sens. Bio-Sens. Res., 2016, 7, 146–152 CrossRef
.
- B. Du, H. Han, Z. Wang, L. Kuang, L. Wang and L. Yu,
et al., targeted drug delivery to hepatocarcinoma in vivo by phage-displayed specific binding peptide, Mol. Cancer Res., 2010, 8(2), 135–144 CrossRef CAS PubMed
.
- V. Foglizzo and S. Marchiò, Bacteriophages as Therapeutic and Diagnostic Vehicles in Cancer, Pharmaceuticals, 2021, 14(2), 161 CrossRef CAS
.
- C. R. Merril, B. Biswas, R. Carlton, N. C. Jensen, G. J. Creed and S. Zullo,
et al., Long-circulating bacteriophage as antibacterial agents, Proc. Natl. Acad. Sci. U. S. A., 1996, 93(8), 3188–3192 CrossRef CAS
.
- N. Principi, E. Silvestri and S. Esposito, Advantages and Limitations of Bacteriophages for the Treatment of Bacterial Infections, Front. Pharmacol., 2019, 10, 513 CrossRef
.
- H. Ling, X. Lou, Q. Luo, Z. He, M. Sun and J. Sun, Recent advances in bacteriophage-based therapeutics: Insight into the post-antibiotic era, Acta Pharm. Sin. B, 2022, 12(12), 4348–4364 CrossRef CAS PubMed
.
- M. Popescu, J. D. Van Belleghem, A. Khosravi and P. L. Bollyky, Bacteriophages and the Immune System, Annu. Rev. Virol., 2021, 8(1), 415–435 CrossRef CAS PubMed
.
- Y. Iwagami, S. Casulli, K. Nagaoka, M. Kim, R. I. Carlson and K. Ogawa,
et al., Lambda phage-based vaccine induces antitumor immunity in hepatocellular carcinoma, Heliyon, 2017, 3(9), e00407 CrossRef PubMed
.
- J. Wang, A. Lamolinara, L. Conti, M. Giangrossi, L. Cui and M. B. Morelli,
et al., HER2-Displaying M13 Bacteriophages induce Therapeutic Immunity against Breast Cancer, Cancers, 2022, 14(16), 4054 CrossRef CAS
.
- M. Ragothaman and S. Y. Yoo, Engineered Phage-Based Cancer Vaccines: Current Advances and Future Directions, Vaccines, 2023, 11(5), 919 CrossRef CAS
, Available from: https://www.ncbi.nlm.nih.gov/pmc/articles/PMC10222954/,.
- V. Monga, H. Mani, A. Hirbe and M. Milhem, Non-Conventional Treatments for Conventional Chondrosarcoma, Cancers, 2020, 12(7), 1962 CrossRef CAS
.
- A. Chongchai, S. Waramit, K. Suwan, M. Al-Bahrani, S. Udomruk and T. Phitak,
et al., Bacteriophage-mediated therapy of chondrosarcoma by selective delivery of the tumor necrosis factor alpha (TNFα) gene, FASEB J., 2021, 35(5), 1–14 CrossRef
.
- J. M. Przystal, S. Waramit, M. Z. I. Pranjol, W. Yan, G. Chu and A. Chongchai,
et al., Efficacy of systemic temozolomide-activated phage-targeted gene therapy in human glioblastoma, EMBO Mol. Med., 2019, 11(4), 1–21 Search PubMed
.
- Y. J. Hwang and H. Myung, Engineered Bacteriophage T7 as a Potent Anticancer Agent in vivo, Front. Microbiol., 2020, 11, 491001 CrossRef PubMed
.
- S. Zuo, G. Dai, L. Wang, Y. Wen, Z. Huang and W. Yang,
et al., Suppression of angiogenesis and tumor growth by recombinant T4 phages displaying extracellular domain of vascular endothelial growth factor receptor 2, Arch. Virol., 2019, 164(1), 69–82 CrossRef CAS
.
- Y. Sun, Y. Sun and R. Zhao, Establishment of MicroRNA delivery system by PP7 bacteriophage-like particles carrying cell-penetrating peptide, J. Biosci. Bioeng., 2017, 124(2), 242–249 CrossRef CAS PubMed
.
- Y. Pan, Y. Zhang, T. Jia, K. Zhang, J. Li and L. Wang, Development of a microRNA delivery system based on bacteriophage MS2 virus-like particles, FEBS J., 2012, 279(7), 1198–1208 CrossRef CAS PubMed
.
- L. Chang, G. Wang, T. Jia, L. Zhang, Y. Li and Y. Han,
et al., Armored long non-coding RNA MEG3 targeting EGFR based on recombinant MS2 bacteriophage virus-like particles against hepatocellular carcinoma, Oncotarget, 2016, 7(17), 23988–24004 CrossRef
.
- M. H. Oh, J. H. Yu, I. Kim and Y. S. Nam, Genetically Programmed Clusters of Gold Nanoparticles for Cancer Cell-Targeted Photothermal Therapy, ACS Appl. Mater. Interfaces, 2015, 7(40), 22578–22586 CrossRef CAS
.
- C. L. Ventola, The antibiotic resistance crisis: part 1: causes and threats, P & T Peer-Rev. J. Formul. Manag., 2015, 40(4), 277–283 Search PubMed
.
- N. Taneja and M. Sharma, Antimicrobial resistance in the environment: The Indian scenario, Indian J. Med. Res., 2019, 149(2), 119 CrossRef
.
- M. S. Mulani, E. E. Kamble, S. N. Kumkar, M. S. Tawre and K. R. Pardesi, Emerging Strategies to Combat ESKAPE Pathogens in the Era of Antimicrobial Resistance: A Review, Front. Microbiol., 2019, 10, 539 CrossRef PubMed
.
- M. Corbellino, N. Kieffer, M. Kutateladze, N. Balarjishvili, L. Leshkasheli and L. Askilashvili,
et al., Eradication of a Multidrug-Resistant, Carbapenemase-Producing Klebsiella pneumoniae Isolate Following Oral and Intra-rectal Therapy With a Custom Made, Lytic Bacteriophage Preparation, Clin. Infect. Dis., 2020, 70(9), 1998–2001 CrossRef CAS PubMed
.
- R. Nir-Paz, D. Gelman, A. Khouri, B. M. Sisson, J. Fackler and S. Alkalay-Oren,
et al., Successful Treatment of Antibiotic-resistant, Poly-microbial Bone Infection with Bacteriophages and Antibiotics Combination, Clin. Infect. Dis., 2019, 69(11), 2015–2018 CrossRef PubMed
.
- A. M. Ghanaim, M. A. Foaad, E. Z. Gomaa, K. A. E. L. Dougdoug, G. E. Mohamed and A. H. Arisha,
et al., Bacteriophage therapy as an alternative technique for treatment of multidrug-resistant bacteria causing diabetic foot infection, Int. Microbiol., 2022, 26(2), 343–359 CrossRef PubMed
.
- A. M. Abd El-Aziz, A. Elgaml and Y. M. Ali, Bacteriophage Therapy Increases Complement-Mediated Lysis of Bacteria and Enhances Bacterial Clearance after Acute Lung Infection with Multidrug-Resistant Pseudomonas aeruginosa, J. Infect. Dis., 2019, 219(9), 1439–1447 CrossRef CAS
.
- V. A. Fischetti, Bacteriophage lysins as effective antibacterials, Curr. Opin. Microbiol., 2008, 11(5), 393–400 CrossRef CAS PubMed
.
- B. Corsini, R. Díez-Martínez, L. Aguinagalde, F. González-Camacho, E. García-Fernández and P. Letrado,
et al., Chemotherapy with phage lysins reduces pneumococcal colonization of the respiratory tract, Antimicrob. Agents Chemother., 2018, 62(6), e02212-17 CrossRef PubMed
.
- L. Leitner, A. Ujmajuridze, N. Chanishvili, M. Goderdzishvili, I. Chkonia and S. Rigvava,
et al., Intravesical bacteriophages for treating urinary tract infections in patients undergoing transurethral resection of the prostate: a randomised, placebo-controlled, double-blind clinical trial, Lancet Infect. Dis., 2021, 21(3), 427–436 CrossRef CAS
.
- A. Petrovic Fabijan, R. C. Y. Lin, J. Ho, S. Maddocks, N. L. Ben Zakour and J. R. Iredell,
et al., Safety of bacteriophage therapy in severe Staphylococcus aureus infection, Nat. Microbiol., 2020, 5(3), 465–472 CrossRef CAS
.
- J. R. Clark and J. B. March, Bacteriophages and biotechnology: vaccines, gene therapy and antibacterials, Trends Biotechnol., 2006, 24(5), 212–218 CrossRef CAS PubMed
.
- J. R. Clark, Bacteriophage therapy: history and future prospects, Future Virol., 2015, 10(4), 449–461 CrossRef CAS
.
- A. Ghaemi, H. Soleimanjahi, P. Gill, Z. Hassan, S. R. M. Jahromi and F. Roohvand, Recombinant lambda-phage nanobioparticles for tumor therapy in mice models, Genet. Vaccines Ther., 2010, 8, 3 CrossRef
.
- J. J. Min, V. H. Nguyen and S. S. Gambhir, Molecular Imaging of Biological Gene Delivery Vehicles for Targeted Cancer Therapy: Beyond Viral Vectors, Nucl. Med. Mol. Imaging, 2010, 44(1), 15–24 CrossRef CAS
.
- B. S. Thomas, S. Nishikawa, K. Ito, P. Chopra, N. Sharma and D. H. Evans,
et al., Peptide vaccination is superior to genetic vaccination using a recombineered bacteriophage λ subunit vaccine, Vaccine, 2012, 30(6), 998–1008 CrossRef CAS PubMed
.
- Y. Tian, M. Zhou, H. Shi, S. Gao, G. Xie and M. Zhu,
et al., Integration of Cell-Penetrating Peptides with Rod-like Bionanoparticles: Virus-Inspired Gene-Silencing Technology, Nano Lett., 2018, 18(9), 5453–5460 CrossRef CAS PubMed
.
- H. M. Li, K. Guo, Z. Yu, R. Feng and P. Xu, Diagnostic value of protein chips constructed by lung-cancer-associated markers selected by the T7 phage display library, Thorac. Cancer, 2015, 6(4), 469–474 CrossRef CAS
.
- T. U. Berendonk, C. M. Manaia, C. Merlin, D. Fatta-Kassinos, E. Cytryn and F. Walsh,
et al., Tackling antibiotic resistance: the environmental framework, Nat. Rev. Microbiol., 2015, 13(5), 310–317 CrossRef CAS PubMed
.
- M. Wu and J. Shu, Multimodal Molecular Imaging: Current Status and Future Directions, Contrast Media Mol. Imaging, 2018, 2018, 1382183 Search PubMed
.
- K. S. Sunderland, M. Yang and C. Mao, Phage-Enabled Nanomedicine: From Probes to Therapeutics in Precision Medicine, Angew. Chem., Int. Ed., 2017, 56(8), 1964–1992 CrossRef CAS
.
- H. Yi, D. Ghosh, M.-H. Ham, J. Qi, P. W. Barone and M. S. Strano,
et al., M13 phage-functionalized single-walled carbon nanotubes as nanoprobes for second near-infrared window fluorescence imaging of targeted tumors, Nano Lett., 2012, 12(3), 1176–1183 CrossRef CAS
, Available from: https://pubmed.ncbi.nlm.nih.gov/22268625/.
- N. M. Bardhan, D. Ghosh and A. M. Belcher, M13 Virus based detection of Bacterial Infections in Living Hosts, J. Biophotonics, 2014, 7(8), 617–623 CrossRef CAS
.
- F. Abbaszadeh, H. E. Leylabadlo, F. Alinezhad, H. Feizi, A. Mobed and S. Baghbanijavid,
et al., Bacteriophages: cancer diagnosis, treatment, and future prospects, J. Pharm. Investig., 2021, 51(1), 23–34 CrossRef
.
- Z. Kaźmierczak, A. Piotrowicz, B. Owczarek, K. Hodyra, P. Miernikiewicz and D. Lecion,
et al., Molecular imaging of T4 phage in mammalian tissues and cells, Bacteriophage, 2014, 4(1), e28364 CrossRef
.
- D. S. Choi, H. E. Jin, S. Y. Yoo and S. W. Lee, Cyclic RGD Peptide Incorporation on Phage Major Coat Proteins for Improved Internalization by HeLa Cells, Bioconjug. Chem., 2014, 25(2), 216–223 CrossRef CAS PubMed
.
- M. A. Dymova, Y. A. Utkin, M. D. Dmitrieva, E. V. Kuligina and V. A. Richter, Modification of a Tumor-Targeting Bacteriophage for Potential Diagnostic Applications, Molecules, 2021, 26(21), 6564 CrossRef CAS
.
- H. Yue, Y. Li, M. Yang and C. Mao, T7 Phage as an Emerging Nanobiomaterial with Genetically Tunable Target Specificity, Adv. Sci., 2022, 9(4), 2103645 CrossRef CAS PubMed
.
- H. Talwar, R. Rosati, J. Li, D. Kissner, S. Ghosh and F. Fernández-Madrid,
et al., Development of a T7 Phage Display Library to Detect Sarcoidosis and Tuberculosis by a Panel of Novel Antigens, EBioMedicine, 2015, 2(4), 341–350 CrossRef
.
- H. Talwar, J. Talreja and L. Samavati, T7 Phage Display Library a Promising Strategy to Detect Tuberculosis Specific Biomarkers, Mycobact. Dis. Tuberc. Lepr., 2016, 6(2), 214 Search PubMed
, Available from: https://www.ncbi.nlm.nih.gov/pmc/articles/PMC5111870/.
- J. H. Lee, D. W. Domaille and J. N. Cha, Amplified protein detection and identification through DNA-conjugated M13 bacteriophage, ACS Nano, 2012, 6(6), 5621–5626 CrossRef CAS
.
- N. Wisuthiphaet, X. Yang, G. M. Young and N. Nitin, Rapid detection of Escherichia coli in beverages using genetically engineered bacteriophage T7, AMB Express, 2019, 9(1), 55 CrossRef
.
- J. Chen, S. D. Alcaine, Z. Jiang, V. M. Rotello and S. R. Nugen, Detection of Escherichia coli in Drinking Water Using T7 Bacteriophage-Conjugated Magnetic Probe, Anal. Chem., 2015, 87(17), 8977–8984 CrossRef CAS PubMed
.
- S. Adhya, C. R. Merril and B. Biswas, Therapeutic and Prophylactic Applications of Bacteriophage Components in Modern Medicine, Cold Spring Harb. Perspect. Med., 2014, 4(1), a012518 CrossRef PubMed
.
- Z. Golkar, O. Bagasra and D. G. Pace, Bacteriophage therapy: a potential solution for the antibiotic resistance crisis, J. Infect. Dev. Ctries, 2014, 8(2), 129–136 CrossRef PubMed
.
- L. Deng, L. I. Ibañez, V. Van Den Bossche, K. Roose, S. A. Youssef and A. De Bruin,
et al., Protection against Influenza A Virus Challenge with M2e-Displaying Filamentous Escherichia coli Phages, ed. S. M. Kang, PLoS One, 2015, 10(5), e0126650 CrossRef
.
- A. Ghaemi, H. Soleimanjahi, P. Gill, Z. M. Hassan, S. Razeghi and M. Fazeli,
et al., Protection of mice by a λ-based therapeutic vaccine against cancer associated with human papillomavirus type 16, Intervirology, 2011, 54(3), 105–112 CrossRef CAS
.
- Y. Wang, Q. Su, S. Dong, H. Shi, X. Gao and L. Wang, Hybrid phage displaying SLAQVKYTSASSI induces protection against Candida albicans challenge in BALB/c mice, Hum. Vaccines Immunother., 2014, 10(4), 1057–1063 CrossRef
.
- A. Samoylov, A. Cochran, B. Schemera, M. Kutzler, C. Donovan and V. Petrenko,
et al., Humoral immune responses against gonadotropin releasing hormone elicited by immunization with phage-peptide constructs obtained via phage display, J. Biotechnol., 2015, 216, 20–28 CrossRef CAS
.
- L. Zhai, J. Peabody, Y. Y. S. Pang, J. Schiller, B. Chackerian and E. Tumban, A novel candidate HPV vaccine: MS2 phage VLP displaying a tandem HPV L2 peptide offers similar protection in mice to Gardasil-9, Antiviral Res., 2017, 147, 116–123 CrossRef CAS
.
-
Bacteriophages: Biology and Applications, ed. E. Kutter and A. Sulakvelidze, CRC Press, Boca Raton, 2004, p. 528 Search PubMed
.
- T. B. Broudy and V. A. Fischetti,
In Vivo Lysogenic Conversion of Tox− Streptococcus pyogenes to Tox+ with Lysogenic Streptococci or Free Phage, Infect. Immun., 2003, 71(7), 3782–3786 CrossRef CAS PubMed
.
- A. Henein, What are the limitations on the wider therapeutic use of phage?, Bacteriophage, 2013, 3(2), e24872 CrossRef PubMed
.
- A. Fauconnier, Phage Therapy Regulation: From Night to Dawn, Viruses, 2019, 11(4), 352 CrossRef PubMed
.
- R. N. Ng, A. S. Tai, B. J. Chang, S. M. Stick and A. Kicic, Overcoming Challenges to Make Bacteriophage Therapy Standard Clinical Treatment Practice for Cystic Fibrosis, Front. Microbiol., 2021, 11, 593988 CrossRef PubMed
.
- N. Principi, E. Silvestri and S. Esposito, Advantages and Limitations of Bacteriophages for the Treatment of Bacterial Infections, Front. Pharmacol., 2019, 10, 513 CrossRef
.
- K. M. Caflisch, G. A. Suh and R. Patel, Biological challenges of phage therapy and proposed solutions: a literature review, Expert Rev. Anti-Infect. Ther., 2019, 17(12), 1011–1041 CrossRef CAS
.
- J. Anomaly, The Future of Phage: Ethical Challenges of Using Phage Therapy to Treat Bacterial Infections, Public Health Ethics, 2020, 13(1), 82–88 CrossRef
.
- T. Briot, C. Kolenda, T. Ferry, M. Medina, F. Laurent and G. Leboucher,
et al., Paving the way for phage therapy using novel drug delivery approaches, J. Controlled Release, 2022, 347, 414–424 CrossRef CAS
.
- A. Pirisi, Phage therapy – advantages over antibiotics?, Lancet, 2000, 356(9239), 1418 CrossRef CAS PubMed
.
- R. J. Payne, D. Phil and V. A. Jansen, Phage therapy: the peculiar kinetics of self-replicating pharmaceuticals, Clin. Pharmacol. Ther., 2000, 68(3), 225–230 CrossRef CAS
.
|
This journal is © The Royal Society of Chemistry 2024 |
Click here to see how this site uses Cookies. View our privacy policy here.