DOI:
10.1039/D2NA00625A
(Review Article)
Nanoscale Adv., 2023,
5, 46-80
Advanced nanomaterials for modulating Alzheimer's related amyloid aggregation
Received
14th September 2022
, Accepted 15th November 2022
First published on 21st November 2022
Abstract
Alzheimer's disease (AD) is a common neurodegenerative disease that brings about enormous economic pressure to families and society. Inhibiting abnormal aggregation of Aβ and accelerating the dissociation of aggregates is treated as an effective method to prevent and treat AD. Recently, nanomaterials have been applied in AD treatment due to their excellent physicochemical properties and drug activity. As a drug delivery platform or inhibitor, various excellent nanomaterials have exhibited potential in inhibiting Aβ fibrillation, disaggregating, and clearing mature amyloid plaques by enhancing the performance of drugs. This review comprehensively summarizes the advantages and disadvantages of nanomaterials in modulating amyloid aggregation and AD treatment. The design of various functional nanomaterials is discussed, and the strategies for improved properties toward AD treatment are analyzed. Finally, the challenges faced by nanomaterials with different dimensions in AD-related amyloid aggregate modulation are expounded, and the prospects of nanomaterials are proposed.
1 Introduction
Protein misfolding can form abnormal amyloid aggregates, further leading to amyloid extracellular deposition.1 These amyloid deposits are widely believed to be closely related to various neurodegenerative diseases and are even considered to be the culprit.2 Among them, Alzheimer's disease (AD) is the most common form of neurodegenerative disease, and according to the “2021 World Alzheimer's Disease Report”, more than 55 million people worldwide have dementia. This number gets even more staggering as it grows daily and is expected to reach 78 million by 2030.3 Although the pathogenesis of AD has not been clearly confirmed, the amyloid plaque hypothesis has been the most widely accepted until now.4 As the most important component of amyloid aggregates, Aβ is derived from the sequential proteolytic cleavage of β-amyloid precursor protein (APP) by β- and γ-secretase in vivo.5 In addition, Aβ is a hydrophobic peptide with a molecular weight of 4 kDa and consists of 39–42 amino acid residues.5,6 The Aβ monomer undergoes secondary structural transitions and misfolds in physiological environments.7 This misfolding Aβ can rapidly self-assemble with surrounding Aβ and form oligomers through hydrophobic interactions. Meanwhile, the oligomers can then decrease through the conversion of non-fibrillar to fibrillar oligomers, elongating fibrillar oligomers and finally forming mature amyloid fibrils.8 The process of Aβ aggregation can trigger the production of intra- and extra-cellular reactive oxygen species (ROS), which can lead to oxidation and cellular damage.9 In addition, neurotoxicity was induced by Aβ oligomers and fibrils through binding to the plasma membrane, resulting in metabolic dysfunction and neuronal cell death.10 Therefore, the inhibition of Aβ fibrillation, the disintegration of mature Aβ aggregates, and the promotion of the clearance of Aβ to maintain the balance of the metabolism and catabolism of Aβ appear to be quite significant for the prevention and treatment of AD. Recently, numerous efforts have been made to inhibit Aβ aggregation by blocking fibril formation and reducing the number of fibrils to halt the extent of AD pathology.10–12 Among them, nanomaterials have great advantages in influencing amyloid fibril nucleation, disintegrating matured amyloid fibrils, and targeting amyloid plaques via crossing the blood–brain barrier (BBB).10,13–16 At the same time, nanomaterials have an ability to respond to light, sound, heat, electricity, and magnetism because of the physical properties of some nanomaterials, and they have also been gradually developed and applied in the research of neurodegenerative diseases.10,17–21
Nanomaterials can be classified into one-dimensional, two-dimensional, zero-dimensional, and other nanomaterials according to their dimensions.22 One-dimensional nanomaterials exhibit a high degree of anisotropy, possessing excellent properties such as plasmon resonance, optical properties and anti-oxidation.23 Two-dimensional nanomaterials have excellent physical and chemical properties, can bind peptides through non-covalent forces, have good biocompatibility, and have good photothermal conversion and photocatalytic capabilities.24–26 The large specific surface area of zero-dimensional nanomaterials makes them have unique physical and chemical properties.27 Besides, some composite nanomaterials prepared from other nanocarriers, such as metal–organic frameworks, polyoxometalates, and silica, have multiple synergistic effects.28–30 Based on the three-dimensional scale of nanomaterials, this review deeply analyzed the advantages/disadvantages of nanomaterials in modulating amyloid aggregation. The modulation roles of nanomaterials in AD treatment mainly include intermolecular interaction, chelation, photothermal effects, photocatalytic oxidation, and drug delivery (Fig. 1A). As shown in Fig. 1B, we exhibited a number of research articles published each year on the application of nanomaterials in amyloid, neurodegenerative disease (ND), and Alzheimer's disease (AD). This exponential growth of research in the related field indicates that nanomaterials for modulating Alzheimer's related amyloid aggregation are not only an emerging research topic, but also possess huge application potential.
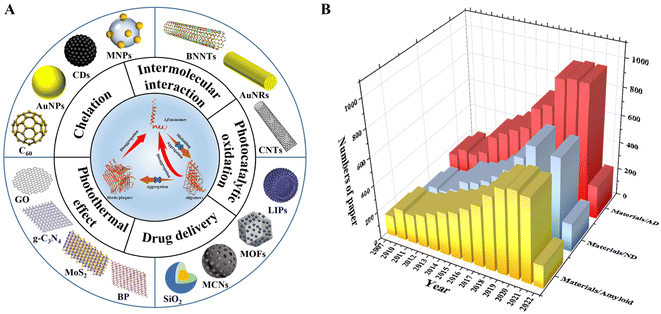 |
| Fig. 1 (A) Schematic illustration of nanomaterials with different functions and dimensions for modulating Aβ aggregation. (B) The number of published papers per year on the application of nanomaterials in amyloid, neurodegenerative disease (ND), and Alzheimer's disease (AD). Data are collected from the Web of Science on June 26, 2022, by advanced search with “Topics = (Materials and Amyloid; Materials and Neurodegenerative Disease; Materials and Alzheimer's disease; Language: (English)”. | |
2 One-dimensional nanomaterials
One-dimensional (1D) nanomaterials, including nanorods, nanotubes, nanoribbons, nanowires, and nanofibers, have been applied as drug carrier or synergistic drug materials.31,32 Due to their unique chemical structures, good biocompatibility, high specific surface area, and other related physicochemical properties, 1D nanomaterials were widely applied to the biological field.33 In recent years, some research showed that 1D nanomaterials with special structures, such as radial size seamless carbon tubes, can interact with amyloid protein and reduce the aggregation of amyloid protein.34
2.1 Carbon nanotubes
Carbon nanotubes with a special structure fabricated from graphene sheets are one-dimensional quantum materials.35 It is mainly composed of several to dozens of layers of coaxial circular tubes of carbon atoms arranged in a hexagonal shape.36 A fixed distance of about 0.34 nm is maintained between layers, and the diameter of nanotubes is generally 2–20 nm.37 According to the different orientations of the hexagon along the axial direction, it can be divided into zigzag, armchair and spiral.38 Single-walled carbon nanotubes (SWCNTs) have been applied in various biological systems because of their good biocompatibility, unique chemical structure, high specific surface area and strong optical absorbance in the near-infrared (NIR) region.39 As unique one-dimensional nanomaterials, SWCNTs have also been explored as novel delivery vehicles for drugs, proteins, and so on.40,41 Due to the strong optical absorbance of SWCNTs in the NIR region, SWCNTs could destroy the structure of cells by local thermal during NIR laser irradiation.42 As a nanocarrier, SWCNTs were used to deliver oligonucleotides into living cells, and oligos were translocated into cell nuclei upon endosomal rupture triggered by NIR laser pulses.43,44 It can be seen that the transporting capabilities of SWCNTs combined with chemical modification and their intrinsic optical properties can lead to new classes of novel nanomedicine for drug delivery and therapy. To the best of our knowledge, SWCNTs have also been developed for inhibiting amyloid fibrillation, disintegration of amyloid fibrils, and promoting the clearance of amyloid plaques. Luo et al.45 firstly studied the pH-dependent molecular interactions between SWCNTs and Aβ peptides by a variety of spectroscopy and atomic force microscopy techniques. They found that the secondary structural transition of Aβ peptides from a random coil to a β-sheet structure could be significantly affected by SWCNTs, and SWCNTs could inhibit the nucleation/elongation phase of Aβ peptide fibrillation by adsorbing Aβ peptides with a β-sheet structure (Fig. 2A). Their research also indicated that Aβ peptides might reduce the toxicity of SWCNTs by the reduction of the hydrophobic surface of SWCNTs. Wei's group46 showed that SWCNTs could inhibit the formation of β-sheet-rich oligomers in the central hydrophobic core fragment of Aβ (Aβ16–22). However, a potential problem with SWCNTs is their poor solubility in water and few functional groups, which will cause a huge hindrance to the inhibition of Aβ fibrillation and other biological applications. Therefore, Xie et al.47 fabricated a type of hydroxylated SWCNTs by modifying with 30 hydroxyl groups. Then they further investigated the influence of hydroxylated SWCNTs on the aggregation of Aβ16–22 peptides using all-atom explicit-water replica exchange molecular dynamics simulations. The results showed that the β-sheet formation, shift in the conformations and disordered aggregation of Aβ16–22 peptides can be significantly inhibited through hydroxylated SWCNTs, which mainly depend on the strong electrostatic, hydrophobic, and aromatic stacking interactions with the residue of Aβ16–22. In addition, Liu et al.48 also researched the ability of hydroxylated SWCNTs for inhibiting Aβ aggregation, disaggregating Aβ fibrils, and protecting Aβ-induced cytotoxicity. The authors found that SWCNT-OH could inhibit Aβ fibrillation and disaggregate mature fibrils in a dose-dependent manner (Fig. 2B). Moreover, the related experience showed that the ratio of hydroxyl groups in SWCNT-OH played an important role in inhibiting Aβ fibrillation. In detail, with the increase the ratio of hydroxyl groups, the inhibitory capacity of SWCNT-OH was greatly improved. Molecular dynamics (MD) simulations further revealed that the interactions between SWCNT-OH and the Aβ11–42 pentamer were found to be dominated by van der Waals interactions. In addition, the inter- and intra-peptide interactions of Aβ fibrillation were significantly weakened by hydrophobic interactions and π–π stacking of Aβ and SWCNT-OH, and SWCNT-OH mainly interact with the six residues of Aβ11–42 (H13, H14, Q15, V36, G37, and G38). In our group, the structure of the Aβ42 monomer affected by tuning the curvature of carbon nanotubes was deeply studied using MD simulations.49 The related research indicated that Aβ42 peptides had an extended structure and a larger number of contacts with the surface of C25. When the curvatures of the carbon nanotubes (CNTs) were high, the peptide wrapped around the CNTs and had less contact with the surfaces (Fig. 2C). Moreover, the CNTs with lower curvatures and the peptides had stronger interactions and induced the collapse of the initial secondary structures of the peptides. With decreasing curvatures, the peptides were arranged diagonally along the nanotube, and the percentages of α-helical structures were reduced. This research indicated that the structural stability, including the nucleation and self-assembly behavior of Aβ42 peptides on SWCNT surfaces, is dependent on the surface curvatures. The disaggregation mechanism of SWCNTs for mature Aβ fibrils was also investigated. For instance, Lin et al.50 explored the interplay between SWCNTs and Aβ fibrils by atomic force microscopy, ThT fluorescence, infrared spectroscopy, and MD simulations at the single SWCNT level. The results demonstrated that SWCNTs could partially destroy the mature Aβ fibrils and form Aβ-surrounded-SWCNT conjugates and cut down the β-sheet structures. Besides, MD simulation confirmed that the disaggregation ability was dependent on the binding sites of Aβ fibrils (Fig. 2D).
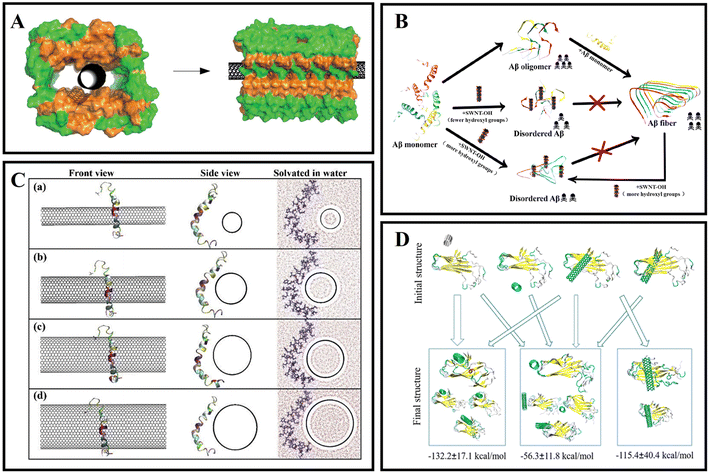 |
| Fig. 2 (A) Illustration of SWNTs located in the hollow core of Aβ fibrils.45 (B) Hydroxylated SWNTs inhibit Aβ42 fibrillogenic and disaggregate mature fibrils.48 (C) Initial configurations of the Aβ42 peptide with SWCNT chiralities of (a) (10, 10), (b) (15, 15), (c) (20, 20) and (d) (25, 25).49 (D) Disaggregation process of Aβ42 fibrils-SWCNTs in 200 ns.50 | |
Compared to SWCNTs, multiwalled carbon nanotubes (MWCNTs) possess obvious advantages, such as lower product cost, excellent chemical stability and drug adsorption potential.51 Lohan et al.52 designed a system of berberine (BRB)-loaded MWCNTs with polysorbate and phospholipid coating. BRB was known to possess neuroprotective actions. Polysorbates and phospholipids have been reported to improve the imaging and targeting utility of CNTs. The results showed that the phospholipid-coated and the polysorbate-coated MWCNTs exhibited remarkable recovery in the memory performance.
2.2 Gold nanorods
Gold nanorods are rod-shaped gold nanoparticles with a size ranging from a few nanometers to hundreds of nanometers.53 Gold is a precious metal material with very stable chemical properties. Gold nanoparticles inherit these properties of bulk materials, so they are relatively stable and have very rich physicochemical properties.54 The surface plasmon resonance wavelength of gold nanorods can be changed with the aspect ratio, continuously adjustable from visible (550 nm) to near-infrared (1550 nm), and an extremely high surface electric field strength enhancement effect.55,56 Gold nanorods have extremely high optical absorption, scattering cross-sections, and photothermal conversion efficiency that is continuously adjustable from 50% to 100%.57,58 Therefore, Au nanorods (AuNRs) exhibit strong localized surface plasmon resonance (LSPR) in the near-infrared spectrum and have good performance in photothermal (PTT) therapy.59,60
Gold nanorods as potential therapy nanomaterials have been utilized to modulate amyloid aggregation. AuNRs were functionalized with a metal-chelating group amide-nitrilotriacetic-CoII (ANTACo) to immobilize soluble RepA-WH1 selectively (Fig. 3A). In the presence of catalytic concentrations of anisotropic nanoparticles, H6-RepA-WH1 undergoes stable amyloid oligomerization.61 Then, such oligomers promote the growth of amyloid fibers of untagged RepA-WH1. Prionoid-functionalized AuNRs as nucleating agents for controlled protein amyloidosis in vitro. AuNR-mediated amyloid nucleation is based on a conformational change from the dimer protein precursor to the immobilized pre-amyloidogenic monomer at the nanoparticle surface, which effectively promotes the oligomerization and fibrillation of amyloid. Lin et al.62 introduced a novel method where AuNRs combined with Aβ fibrils can be efficiently destroyed under fs-laser irradiation without increasing the cytotoxicity. The fs-laser could trigger the nanoexplosion of AuNRs by LSPR and bring the Aβ fibrils into non-β-sheet structure components. Sudhakar et al.63 fabricated AuNRs and utilized them to inhibit the aggregation of Aβ by a NIR laser. Meanwhile, the shape-dependent plasmonic properties of AuNRs are exploited to facilitate faster disaggregation of mature Aβ fibrils. In addition, a related study found that 1,2-dimyristoyl-sn-glycero-3-phosphocholine (DMPC) stabilized AuNRs can inhibit the formation of fibrils due to selective binding to the positively charged amyloidogenic sequence of Aβ protein (Fig. 3B). This research exhibited a dual effect: inhibition of Aβ fibrillation and NIR laser facilitated the dissolution of mature Aβ fibrils. However, the role of heat generation by AuNRs, which promoted the disaggregation of fibrils, had not been explained from a molecular perspective.63 Then Liu et al.64 prepared CTAB-stabilized AuNRs with different sizes (CTAB as cetyltrimethylammonium bromide), and the effect of diameters and lengths of AuNRs on Aβ fibrillation was in-depth studied. A related fluorescence experiment indicated that in the presence of CTAB-stabilized AuNRs with different sizes, the formation of larger oligomers and fibrils was inhibited, and the inhibition efficiency decreased with the decrease of diameters of AuNRs (Fig. 3C). For the AuNRs with the same diameter, the inhibition efficiency decreased with the length of Au NRs. A CD experiment indicated that AuNRs with larger sizes inhibited the formation of a β-sheet structure to some extent. In summary, CTAB-stabilized AuNRs inhibited the kinetic process of Aβ fibrillation, and the inhibition efficiency of larger AuNRs was better. Meanwhile, the sizes of AuNRs played a key role in modulating the kinetic aggregation process of Aβ fibrillation. This work found that the rate constant had a positive relationship with the diameters or lengths of CTAB-stabilized AuNRs. Interestingly, Liu et al.65 studied the NIR absorption properties of AuNRs loaded with a single chain variable fragment and thermophilic acylpeptide hydrolase as a smart theranostic complex GAS, which possesses both rapid detection of Aβ aggregates and NIR photothermal treatment that effectively disaggregates Aβ aggregates and reduces Aβ-mediated toxicity (Fig. 3D). Morales-Zavala et al.66 synthetized a polyethylene glycol stabled and dual-peptide modified gold nanorod complex. A related study determined that the nanoconjugate does not affect neuronal viability. The nanoconjugate could penetrate the cells and decrease the Aβ peptide aggregation in vitro. Subsequently, Morales-Zavala et al.67 also developed a neurotheranostic platform based on AuNRs, which works as a therapeutic peptide delivery system. As a diagnostic tool, the platform could be detected in vivo through microcomputed tomography (micro-CT). Ang2 and D1 peptide modified AuNRs induced the diminution of both the amyloid load and inflammatory markers in the brain of the AD model. The differences in GNRs-D1/Ang2 between wild type (WT) and AD mice were observed in vivo. The two peptide modified AuNRs can improve the delivery and retention of this platform in the brain and reinforce the therapeutic benefits associated with the β-sheet breaker ability of the D1 peptide.
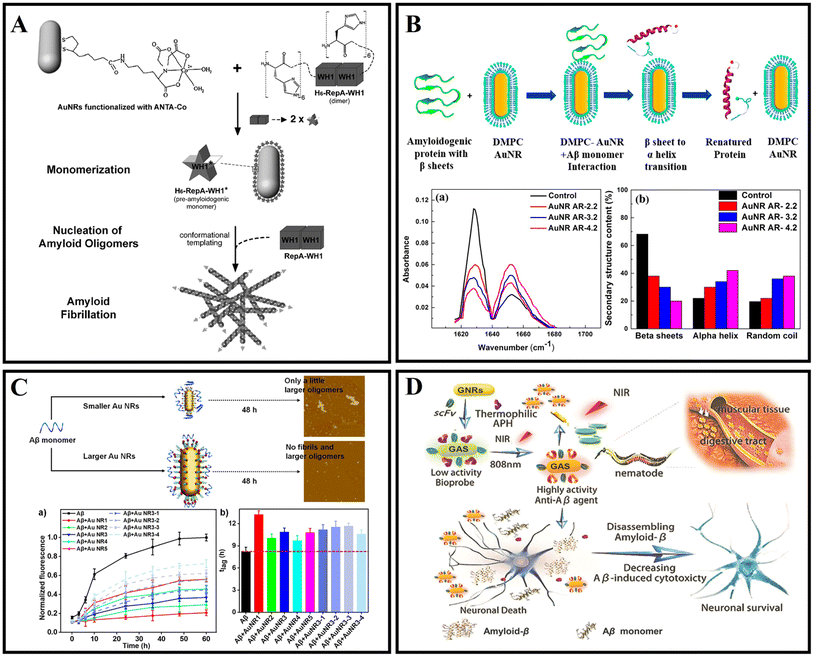 |
| Fig. 3 (A) Illustration of the nucleation of RepA-WH1 amyloidogenesis by prionoid-functionalized AuNRs.61 (B) Interaction between AuNRs and Aβ protein monomer and conversion of the β-sheet to an α-helix secondary structure.63 (C) CTAB-stabilized AuNRs with different sizes inhibiting Aβ peptide aggregation.64 (D) The GAS with NIR absorption is used for AD diagnosis and treatment.65 | |
3 Two-dimensional nanomaterials
Two-dimensional (2D) nanomaterials refer to nanomaterials that have only one dimension on the nanometer scale.68 Because of their huge specific surface area and special surface structure, 2D nanomaterials can adsorb and interact with various molecules such as drugs, nucleic acids, peptides, and proteins.69 Two-dimensional nanomaterials also have the ability to penetrate biological barriers.70 Therefore, as a drug carrier, 2D nanomaterials can load numerous drugs and cross various biological barriers.71,72 Meanwhile, 2D nanomaterials can also absorb and immobilize amyloid protein by interacting with interfaces.73 Some 2D nanomaterials possess light-responsive properties and have great potential in photothermal and photodynamic therapy.69,74 Two-dimensional nanomaterials have good peroxidase-like properties and can alleviate oxidative stress.75 Based on the advanced properties, 2D nanomaterials have been attractive in AD diagnosis and treatment.76 2D nanomaterials have been used in AD research, mainly including graphene nanosheets, carbon nitride nanosheets, black phosphorus nanosheets, and transition metal dichalcogenide nanosheets. Besides, some studies have shown that 2D MOFs, MXenes, hexagonal boron nitride and so on also have applications in AD diagnosis and treatment.
3.1 Graphene
Graphene or graphene oxide (GO), one of the two-dimensional nanomaterials, consists of mono-layer carbon atoms with conjugated π–π.77 Due to the excellent electrical conductivity, ultra-high specific surface area, high mechanical strength, good biocompatibility, and photothermal conversion characteristics, graphene has been widely used in biomedical fields such as bioimaging, biosensing, and drug delivery.78–80
Mahmoudi et al.81 indicated that GO and protein-coated GO can delay the Aβ fibrillization process via adsorption of amyloid monomers. Then Li et al.82 further confirmed that the binding between the peptide monomer and the surface of the GO sheets can redirect the assembly pathway of Aβ (Fig. 4A). Wang et al.83 examined the size effect of GO on modulating amyloid peptide assembly and found that GO with a large size has a relatively stronger modulation effect for the aggregation of Aβ33–42. The advantages of graphene nanocomposites are even more obvious. As shown in Fig. 4B, Ahmad et al.84 successfully fabricated nanocomposites of iron oxide and graphene oxide (GOIO) using solvothermal methods. Due to the high surface area of GOIO, GOIO can effectively interact with Aβ42, inhibit the formation of mature fibrils from Aβ42 monomers and maintain the secondary structure of Aβ42 into a random coil or α-helix-rich structure. Many researchers have worked to investigate the mechanism of action of graphene bias with Aβ. The penetration and extraction of graphene were identified as two main mechanisms for scavenging fibrils (Fig. 4C).85 This is because of the strong interaction between graphene and amyloid fibrils through π–π stacking and hydrophobic interaction due to the special sp2 structure of graphene. Graphene nanosheets can extract single peptide molecules from mature amyloid fibrils into their surface, and the absorption interaction is further enhanced by π–π stacking because of the aromatic residues of Aβ and the sp2 structure of graphene. Chen et al.86 investigated the oligomerization of Aβ33–42 by performing replica exchange MD simulations on Aβ33–42 peptide chains in the absence and presence of two different sizes of GO, and found that GO inhibited Aβ33–42 oligomerization by making Aβ33–42 peptides separate from each other. Jin et al.87 revealed the mechanism of GO nanosheets in inhibiting Aβ42 aggregation through MD simulations, and found that GO mostly suppressed the β-sheet formation of Aβ42 by weakening inter-peptide interactions mostly via the salt bridge, hydrogen bonding and cation–π interactions with charged residues D1, E3, R5, D7, E11, K16, E22, K28 and A42. The π–π and hydrophobic interactions between GO and Aβ42 also play a key role in the inhibition of Aβ aggregation. Meanwhile, Yin et al.88 indicated that the adsorption capacity with Aβ of graphene's surface varies significantly depending on its curvature. The negative curved surface is more likely to adsorb Aβ than the positive curved surface. These findings showed that the shape of the nanoparticle is important in determining its interaction with the peptide. He et al.89 investigated the thermodynamics and kinetics of fibril elongation on GO surfaces with different oxidative degrees. This study revealed that the behaviors of GO in fibril elongation depend on the balance between the promoting effect by templating the incoming of monomers and the retarding effect by capturing the monomer during docking and locking phases through hydrogen bonding. Subsequently, Li et al.90 also further demonstrated that GO could clear amyloids by inducing microglia and neuron autophagy. Photothermal therapy can be used to dissolve mature Aβ fibrils. As shown in Fig. 4D, Qu's group firstly reported the photothermal treatment for AD using graphene nanosheets. Thioflavin S (ThS) which can specifically bind to Aβ fibers was covalently linked to the surface of GO. The prepared GO–ThS nanocomposites have a uniform diameter of 100 to 200 nm, and the thickness of GO–ThS nanocomposites is about 1.5 nm. The related research showed that GO–ThS can cross the BBB, selectively interact with Aβ40 fibrils, and disaggregate Aβ40 fibrils under near-infrared (NIR) laser irradiation. Moreover, the decomposition of Aβ40 fibrils can be monitored by the fluorescence changes of ThS in real time.91 Xia and Maciel et al.92,93 have reported a potential drug carrier for loading drugs using GO through non-covalent interactions. Wang et al.94 prepared a novel nanocomposite GO@Dau from GO and dauricine (Dau), and the benzene ring on Dau can be adsorbed by GO by forming a non-covalent bond. GO@Dau will both have anti-inflammatory and anti-oxidative stress capabilities and inhibit Aβ misfolding. This study further found that GO@Dau can effectively enrich in the brain after intranasal administration and GO@Dau can be internalized into the olfactory bulb by endocytosis or pinocytosis of olfactory neurons, and then released and distributed into the brain. More interestingly, researchers found that GO@Dau could increase superoxide dismutase levels, decrease reactive oxygen species and malondialdehyde levels in vitro, and attenuate cognitive memory deficits and glial cell activation for AD mice.
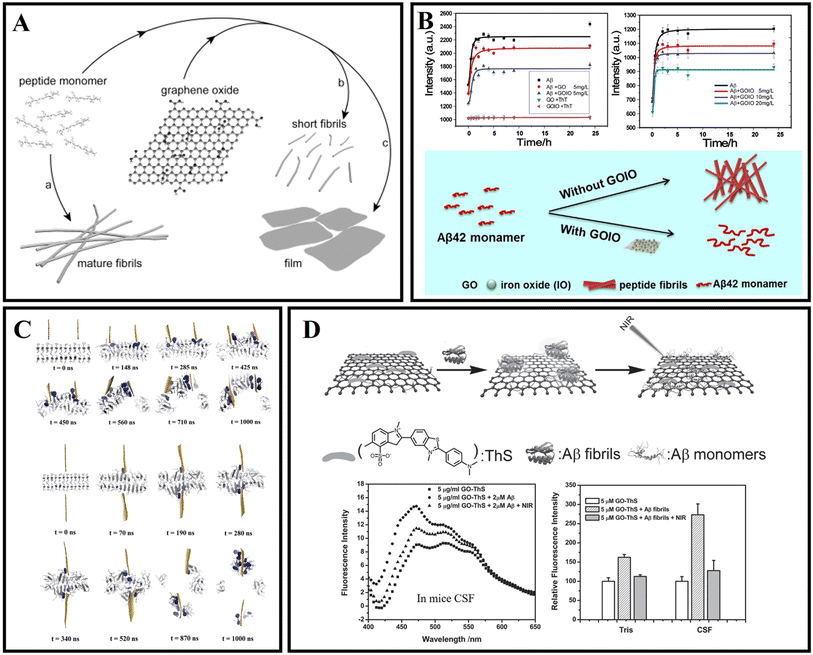 |
| Fig. 4 (A) The surface of graphene-oxide sheets redirects the amyloid–peptide assembly process.82 (B) Kinetics of Aβ42 fibrillation and illustration of modulation of Aβ42 aggregation by using GOIO.84 (C) Graphene nanosheet penetration and Aβ peptide extraction. Featuring two graphene sheets attacking a pre-formed Aβ amyloid fibril from the same side, and the two graphene sheets attacking from both sides.85 (D) GO–ThS effectively dissolve the amyloid deposits of Aβ40 upon NIR laser irradiation.91 | |
Overall, graphene nanosheets and their nanocomposites have been reported for use in AD therapy. However, the specific-targeted issue and drug delivery modalities of graphene still need to be elucidated. Especially, the BBB penetration of graphene is needed to be deeply researched. Through functionalization and size or shape adjustment for nanomaterials, utilizing paracellular pathway, transcellular lipophilic pathway, transport proteins, receptor-mediated transcytosis, and adsorptive-mediated transcytosis could achieve penetration of the BBB.95,96 Although many investigators have studied and summarized the biodistribution characteristics, in vivo clearance, toxicity, and interactions with biological systems of GO, there is still much to be unveiled that would allow safe and effective therapy.97,98
3.2 g-C3N4
Graphitic carbon nitride (g-C3N4) is the most stable allotrope of carbon nitride under ambient conditions.99 g-C3N4 has thermodynamic stability, good biocompatibility, low toxicity, and unique photocatalytic properties.100–102 It has received extensive attention in biological applications in recent years.103
In 2016, Li et al. firstly used g-C3N4 as an Aβ inhibitor for AD treatment.104 As shown in Fig. 5A, g-C3N4 nanosheets could effectively inhibit the formation of Aβ aggregates, separate the preformed Aβ–Cu2+ aggregates, and reduce the intracellular reactive oxygen species (ROS) levels. Then, Li et al.105 combined the advantages of g-C3N4 nanosheets with some metal complexes to fabricate platinum(II)-coordinated g-C3N4 nanosheets (g-C3N4@Pt), and g-C3N4@Pt was able to inhibit Aβ fibrillation. As shown in Fig. 5B, g-C3N4@Pt could effectively inhibit the aggregation of Aβ through non-covalent interaction and photooxidation. As shown in Fig. 5C, Wang et al.106 prepared a nanocomposite which is named GO/g-C3N4 by the sonochemical method. Under UV light irradiation, GO/g-C3N4 could disaggregate mature Aβ fibrils. GO could act as an Aβ collector by adsorption interaction and g-C3N4 could serve as a cleaner by photodegradation. Notably, the photodegradation efficiency of the composite could be kept high because the heterojunction between GO and g-C3N4 helps to separate the photoexcited electron–hole pairs. In 2020, Wang et al.107 reported a kind of novel gold nanoparticle modified g-C3N4 (Au/g-C3N4), which can effectively degrade preformed amyloid aggregates, and the photodegradation of amyloid aggregates mainly depends on the generation of oxygen radicals, especially hydroxyl radicals. As shown in Fig. 5D, Chung et al.108 verified that g-C3N4 can effectively inhibit the aggregation of Aβ under light illumination. Under visible light irradiation, g-C3N4 nanosheets could generate ROS through photo-induced electron transfer, and oxidize Aβ protein, preventing Aβ misfolding and fibrillation. The inhibition efficiency of g-C3N4 for Aβ aggregation will be increased with the concentration and absorbance intensity of g-C3N4 under LED irradiation. Doping metal ions, such as iron, can help g-C3N4 nanosheets accelerate the charge transfer activity, resulting in high ROS generation for inhibiting Aβ aggregation.109
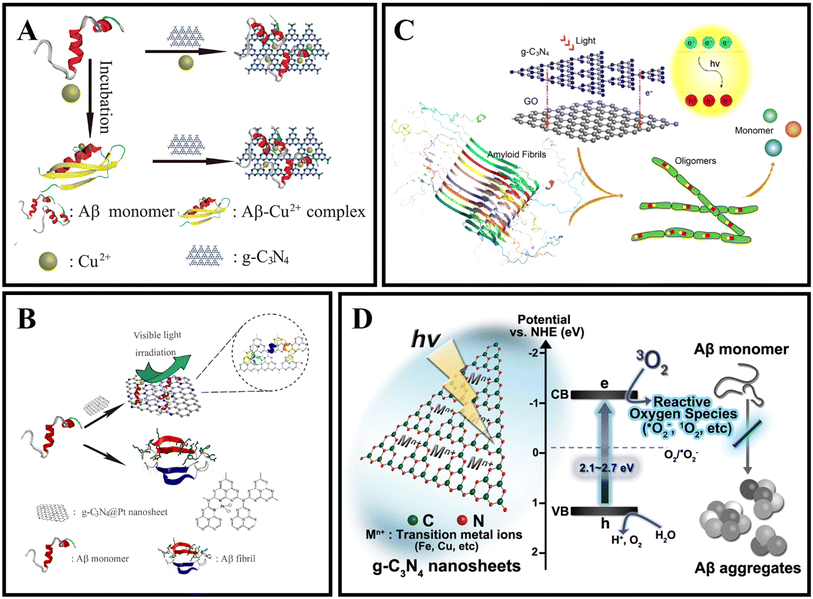 |
| Fig. 5 (A) The ultrathin g-C3N4 nanosheets can effectively inhibit Cu2+ induced Aβ aggregation and disaggregate the preformed Aβ–Cu2+ aggregates.104 (B) g-C3N4@Pt was used for AD treatment.105 (C) The disaggregation of Aβ aggregates by GO/g-C3N4 under light irradiation.106 (D) Highly reactive ROS trigger peptide oxidation that suppresses further fibril formation of Aβ.108 | |
g-C3N4 has some inherent disadvantages, such as poor water solubility, relatively large particle size, and lack of absorption above 460 nm, but its reliable biocompatibility at certain doses proves its potential for biological applications.110–112 For g-C3N4 applications in living organisms, issues such as autofluorescence, optical therapeutic efficiency, and in vivo clearance rates still need to be addressed.103
3.3 Black phosphorus
Black phosphorus (BP) nanosheets, a novel two-dimensional layered semiconductor nanomaterial, have attracted extensive attention due to their good optical, thermal properties, photocatalytic properties, and biological compatibility.113,114 BP can be degraded into non-toxic phosphate and phosphite anions under physiological conditions.115 BP nanosheets can efficiently and selectively capture Cu2+ to protect neuronal cells from Cu2+-induced neurotoxicity.116 Moreover, due to the photothermal transition efficiency, BP nanosheets can cross the BBB by relying on NIR laser irradiation.117
In 2019, Lim et al.118 synthesized two kinds of typical BP nanomaterials with different sizes, titanium ligand-modified BP nanosheets (TiL4@BPNSs) and titanium ligand-modified BP quantum dots (TiL4@BPQDs). The results showed that TiL4@BPNSs and TiL4@BPQDs inhibited Aβ40 aggregate by adsorbing Aβ40 monomers. Then, Yang et al.119 designed a PEG-stabilized BP nano-system PEG-LK7@BP, which can effectively inhibit the formation of Aβ42 fibrils (Fig. 6A). In addition, as a peptide inhibitor, LK7 was coupled to the BP surface via electrostatic and p–π interactions. PEG was used to enhance the stability of BP. PEG-LK7@BP inhibited Aβ42 fibrillation in a dose-dependent manner. Importantly, PEG-LK7@BP has no cytotoxicity to normal cells and can effectively alleviate the cytotoxicity induced by Aβ. The inhibition ability of PEG-LK7@BP can be attributed to multiple effects: (1) PEG-LK7@BP can bind with Aβ through electrostatic and hydrophobic interactions. (2) LK7 can enhance the targeted properties of PEG-LK7@BP for Aβ amyloid. (3) PEG enhanced the stability and dispersibility of the nanomaterials. Cu2+ can catalyze the production of ROS and cause neuronal apoptosis.120 Therefore, it is needed to design novel nanomaterials for not only capturing excess metals but also crossing the BBB. As shown in Fig. 6B, Chen et al.121 demonstrated that BP nanosheets can efficiently and selectively chelate Cu2+ to inhibit neurotoxicity induced by Cu2+. Importantly, under the irradiation of a NIR laser, the BBB permeability of BP nanosheets is significantly improved due to the photothermal effect.
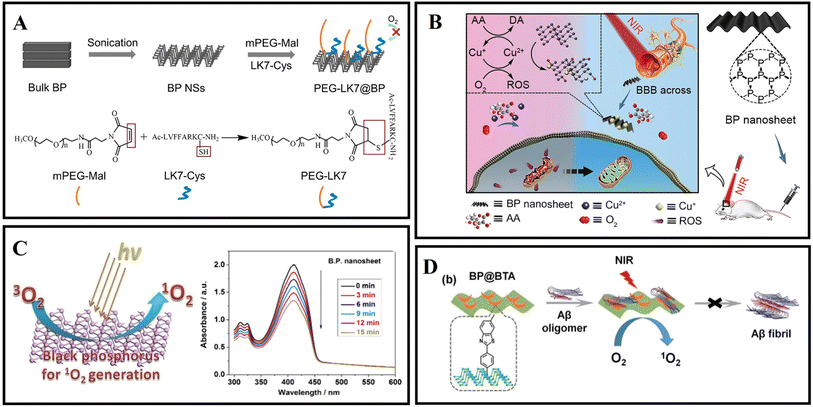 |
| Fig. 6 (A) The preparation of PEG-LK7@BP and the reaction of mPEG-Mal with LK7-Cys during PEG-LK7@BP formation.119 (B) BP nanosheets as a BBB penetrable nanocaptor to reduce oxidative stress production through capturing Cu ions.121 (C) Ultrathin BP nanosheets for efficient singlet oxygen generation.125 (D) BP@BTA produced 1O2 under NIR to inhibit Aβ aggregation.126 | |
Due to the properties of precise treatment and fewer side effects for various diseases, photodynamic therapy (PDT) has attracted extensive attention in the biomedical field.122,123 However, some photosensitizers suffer from low catalytic efficiency, a short absorption wavelength, poor biocompatibility, and non-degradability in living tissues.124 In 2015, Wang et al.125 first demonstrated that exfoliated BP nanosheets are effective photosensitizers for generating 1O2, and the quantum yield is about 0.91 (Fig. 6C). These excellent properties make BP nanosheets photocatalysis nanomaterials in PDT therapy. As shown in Fig. 6D, Qu's group designed a near-infrared responsive nanomaterial based on BP nanosheets.126 The authors also utilized BTA (one of the thioflavin-T derivatives) to modify black phosphorus, aiming to recognize Aβ and enhance BP stability. BP@BTA could generate 1O2 efficiently and the inhibition efficiency of Aβ fibrillation was effectively heightened.
Compared with other 2D materials, BP exhibits a tunable energy bandgap from about 0.3 eV (bulk) to 2.0 eV (monolayer), allowing broad absorption across the entire ultraviolet and infrared regions.127,128 Moreover, the degradable character of BP from element to nontoxic and biocompatible phosphorus oxides is endowed with good biocompatibility in vivo.129
3.4 Transition metal dichalcogenides
Different from carbon or phosphorus-based two-dimensional (2D) nanomaterials, transition metal dichalcogenide nanosheets have become alternative candidates, such as MoS2 and WS2. MoS2 and WS2 are sandwich structures composed of hexagonal metal atoms sandwiched between two layers of chalcogens.130 Transition metal dichalcogenide nanosheets were shown to address biological and medical fields due to their novel nanoscale structures, rich physics, and high mobility.131–133 The basal plane of transition metal disulfide nanosheets can adsorb or conjugate various aromatic hydrocarbons (such as pyridine and purine) and other compounds.134 In recent years, transition metal dichalcogenide nanosheets have been reported for drug delivery and tissue ablation.135
In 2013, Chou et al.136 prepared MoS2 by a chemical exfoliation method and obtained a two-dimensional amphiphilic compound with good colloidal stability in aqueous media. Wang et al.137 explored the effect of MoS2 on the fibrillation process of Aβ fragments and human islet amyloid polypeptide (hIAPP) fragments. A related study found that MoS2 allows for concentration-dependent modulation of amyloid aggregation. Mudedla et al.138 applied MD simulations to deeply study the interaction mechanism between amyloid fibrils and MoS2-based nanomaterials. MoS2-based nanomaterials cause the disruption of the secondary structure and change the β-sheet conformation to a flipped form. The results exhibited that the intermolecular force of peptides, including hydrophobic and hydrophilic interactions, was reduced due to the interaction between peptide and molybdenum disulfide materials. More destabilization of the fibril under nanotubes is observed compared to the nanosurfaces due to the difference in binding modes (Fig. 7A). Regrettably, no corresponding in vivo studies were performed. Liu et al.139 studied the effect of gold nanoparticle-doped molybdenum disulfide (AuNP-MoS2) nanocomposites on the aggregation of Aβ40. Low concentrations of AuNP-MoS2 can enhance the nucleation of Aβ40 and accelerate the aggregation of Aβ40. Although high concentrations of AuNP-MoS2 can enhance the nucleation of Aβ40 protein, it ultimately inhibits the Aβ40 aggregation process (Fig. 7B). It may be attributed to the interaction between AuNP-MoS2 and Aβ40 protein. A low concentration of AuNP-MoS2 can act as a nucleus. As the concentration of AuNP-MoS2 was increased, the structural transformation of the Aβ40 peptide was limited, leading to efficient inhibition of Aβ40 aggregation. MoS2 can rapidly heat up under NIR irradiation so that MoS2 can be used for photothermal therapy. Wang et al.140 designed multifunctional MoS2/AuNRs through the combination of MoS2 nanosheets and AuNRs. MoS2/AuNR can disrupt mature fibrils under NIR irradiation and prevent Aβ protein-induced neurotoxicity. It is worth mentioning that both MoS2 nanosheets and AuNRs can be used as NIR photothermal agents, and the MoS2/AuNR nanocomposites enhance the ability to destroy Aβ fibrils and enhance cell viability by generating localized heat under NIR irradiation (Fig. 7C). Because the specific cleavage sites of Aβ are often embedded in the β-sheet structure, artificial enzyme inhibition efficiency is severely hindered in practical applications. Qu's group constructs a NIR controllable artificial metalloprotease (MoS2-Co) using a MoS2 nanosheet and a cobalt complex of 1,4,7,10-tetraazacyclododecane-1,4,7,10-tetraacetic acid (Codota).141 MoS2-Co circumvented the β-sheet structural restrictions by simultaneous inhibition of the conformational switch from the random-coil to β-sheet structures and modulation of β-sheet structures of the preformed Aβ fibrils (Fig. 7D).
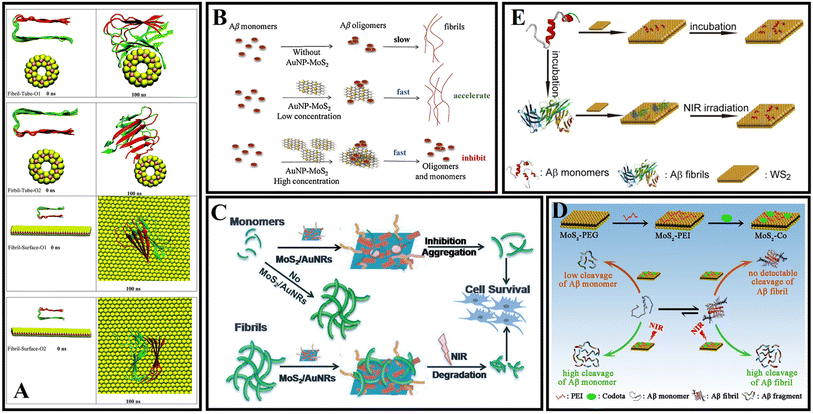 |
| Fig. 7 (A) Initial and final snapshots of the interaction between amyloid fibrils and MoS2 nanomaterials.138 (B) Concentration-dependent mechanism of AuNP-MoS2 nanocomposites in Aβ40 aggregation.139 (C) MoS2/AuNR nanocomposites with high NIR absorption were used for inhibiting β-amyloid aggregation.140 (D) MoS2-Co improved the hydrolytic activity toward Aβ monomers and enhanced the hydrolytic capacity toward Aβ fibrils in the presence of a NIR laser.141 (E) WS2 nanosheets with high NIR absorbance are used for AD treatment.142 | |
Li et al.142 found that WS2 nanosheets could effectively inhibit Aβ40 aggregation. Under van der Waals forces and electrostatic interactions, Aβ40 monomers can be selectively adsorbed on the nanosheet surface. WS2 has high NIR absorption properties, which can dissociate Aβ40 fibrils under NIR irradiation (Fig. 7E). Compared with traditional small molecular Aβ inhibitors, WS2 nanosheets can cross the BBB and exhibit excellent physicochemical characteristics.
The synthesis and modification methods of transition metal dichalcogenide nanosheets need to be further optimized. The preparation of nanosheets of specific thickness and size is essential. In addition, targeting issues and the biodegradation behavior of nanosheets need to be further explored.
3.5 Others
2D COFs, MXenes, hexagonal boron nitride and so on have also been reported for use in AD diagnosis and treatment.120,143,144
Covalent organic frameworks (COFs) are a new generation of nanoparticles consisting of carbon, oxygen, nitrogen and hydrogen atoms with excellent biocompatibility.145 2D COFs have a highly tunable structure and can be designed to cross the blood–brain barrier and inhibit Aβ aggregation. Maleki et al.143 combined experimental and molecular simulation tools to investigate the interaction of novel two-dimensional COF materials with Aβ. The results indicate that amine-functionalized COFs with large surface areas have the potential to inhibit Aβ aggregation. Amine-functionalized groups were also found to enhance the ability of COFs to break the BBB. Two-dimensional transition metal carbides and/or nitriles (MXenes) possess a variety of enzyme-mimetic activities such as superoxide dismutase (SOD), catalase (CAT) and peroxidase (POD), which can be used for ROS scavenging against oxidative stress-induced inflammation and neurotoxicity. MXenes have good photothermal properties and improve the permeability of the BBB. Du et al.120 engineered 2D ultrathin Nb2C nanosheets to chelate metal ions and alleviate oxidative stress. In vitro experiments and theoretical calculations have demonstrated the antioxidant properties of Nb2C MXenzyme nanosheets and their specific chelating effect on Cu2+. In addition, the photothermal conversion properties of Nb2C MXenzyme nanosheets give them the ability to cross the BBB non-invasively.
Boron nitride nanomaterials have good chemical stability, antioxidant properties and biocompatibility. Unlike carbon nanomaterials, boron nitride nanomaterials are less hydrophobic and can maintain the conformation of Aβ rather than change it. Sorout et al.146 found that the interpeptide contacts are largely reduced in the presence of (3,3) boron nitride nanotube (BNNT) and that the nanoparticle interacts with the trimer in such a way that the initial helical secondary structure of the Aβ peptide is retained. The effect of different curvatures of boron nitride on Aβ aggregation was then continued to be investigated. And it was found that the planar boron nitride nanosheet (BNNS) with zero curvature is found to prevent β-sheet formation by converting the secondary structure of the peptide to dominant coil and turn conformations.144 The total number of peptide-nanoparticle contacts increases with a decrease in the curvature and a corresponding increase in the nanoparticle surface area. In addition, boron nitride nanoparticles have been reported as nanocarriers/agents to ameliorate Aβ-induced cytotoxicity.147,148 Currently for boron nitride nanomaterials differences from carbon nanomaterials have been revealed. Further research is expected to lead to a new generation of AD therapeutic nano-agents. Table 1 lists the mechanism and effect of two-dimensional inhibitors on the modulation of amyloid aggregation.
Table 1 A list of two-dimensional inhibitors for the modulation mechanism and effect of amyloid aggregation
Nanomaterials |
Modulation mechanism |
Effect |
Ref. |
GO |
Adsorption/size effect |
Delay |
85
|
GO–ThS |
Photothermal |
Disaggregation |
91
|
GOIO |
Adsorption |
Inhibition |
84
|
GO@Dau |
Adsorption/anti-oxidation |
Inhibition/disaggregation |
94
|
g-C3N4 |
Chelation |
Inhibition/disaggregation |
108
|
g-C3N4@Pt |
Noncovalent interactions/platinum coordination/photooxygenation |
Inhibition/disaggregation |
105
|
Au/g-C3N4 |
Photooxygenation |
Disaggregation |
104
|
GO/g-C3N4 |
Photooxygenation |
Disaggregation |
106
|
g-C3N4 |
Photooxygenation |
Inhibition |
107
|
BP |
Adsorption |
Regulate the aggregation |
118
|
PEG-LK7@BP |
Electrostatic/hydrophobic interactions |
Inhibition |
119
|
BP@BTA |
Photooxygenation |
Inhibition |
126
|
MoS2 |
Adsorption |
Modulation |
137
|
MoS2-Co |
Photothermal |
Inhibition/disaggregation |
141
|
MoS2/AuNR |
Photothermal |
Modulation/disaggregation |
140
|
AuNPs-MoS2 |
Concentration |
Acceleration/inhibition |
139
|
WS2 |
Photothermal/van der Waals/electrostatic interactions |
Inhibition/disaggregation |
142
|
2D COFs |
van der Waals/electrostatic interactions/hydrogen bonds |
Acceleration/inhibition |
143
|
MXene |
Chelation |
Reducing ROS levels |
120
|
BNNS |
Adsorption |
Modulation |
144
|
4 Zero-dimensional nanomaterials
Zero-dimensional (0D) nanomaterials, including gold nanoparticles (GNPs), gold nanoclusters, organic and inorganic quantum dots, metal oxide nanoparticles, and carbon-based nanomaterials, have attracted extensive research interest in the field of biomedicine in recent years.149 The edge effect, quantum confinement effect, ultra-small size and good biocompatibility of 0D nanomaterials endow them with many functions and special performance, such as photoluminescence (PL), tissue penetration, bioactivity, and drug loading capability.149 Therefore, various 0D nanomaterials have been applied to diagnose and treat diseases, such as neurodegenerative disease, cancer and infection.150 Moreover, some advanced 0D nanomaterials can overcome the BBB and inhibit AD-related amyloid aggregation, so they are utilized to treat Alzheimer's disease.151–153 In this part, we summarized diverse treatment methods for amyloid and related neurodegenerative diseases by using different 0D nanomaterials.
4.1 Gold nanoparticles
Gold nanoparticles (AuNPs) have attracted great interest as a novel platform in catalysis, drug delivery, and disease diagnosis/treatment owing to their biocompatibility, intriguing optical properties, surface functionalization, and immunological properties.154,155 Also, due to the diverse sizes, shapes, and surface properties, AuNPs have also been constructed to treat diverse central nervous system diseases. Moreover, AuNPs have been applied to modulate AD-related Aβ fibrillation under intracellular/extracellular spaces.156 Liao et al.157 studied the surface charge of AuNPs by different surface functionalization modifications for effecting Aβ fibrillation. Interestingly, although bare and negatively charged AuNPs both could effectively inhibit Aβ fibrillization and disaggregate Aβ fibrils and spherical oligomers compared with positively charged AuNPs, the negatively charged AuNPs exhibited higher inhibition ability than bare AuNPs during Aβ fibrillization-reduced neurotoxicity. Moreover, the neurotoxicity decreased only when incubated with bare and negatively charged AuNPs in a concentration-dependent manner (Fig. 8A). Apart from that, Wang et al.158 also studied the different shapes and effects of AuNPs on the aggregation of Aβ. The authors firstly prepared gold nanospheres (AuNSs) and gold nanocubes (AuNCs). The results of thioflavin T fluorescence assay showed that both AuNSs and AuNCs could inhibit Aβ fibrillation, but the effect efficiency of AuNSs is stronger than that of AuNCs. As shown in Fig. 8B, the shape of AuNPs influences the fibrillation kinetics of Aβ and the morphologies of Aβ fibrils. As a possible mechanism of shape-dependent AuNP–Aβ interactions, the authors analyzed that the surface energy of AuNPs is key for driving interaction between peptides and NPs. The AuNPs with an enormous specific surface area will inevitably adsorb peptide molecules on their surface. Compared to AuNCs, the spherical surface produces a large density of low-coordinated atoms situated on the edges and corners of AuNSs. Therefore, AuNSs have a stronger interaction with Aβ than AuNCs. Coincidentally, Tapia-Arellano et al.159 also found that the shape of the AuNPs could affect the aggregation kinetics of Aβ. They researched the effect of flat gold nanoprisms (AuNPr) and curved gold nanospheres (AuNSs) on Aβ aggregation kinetics and found that AuNPr accelerated the aggregation process and AuNSs slow down this process.
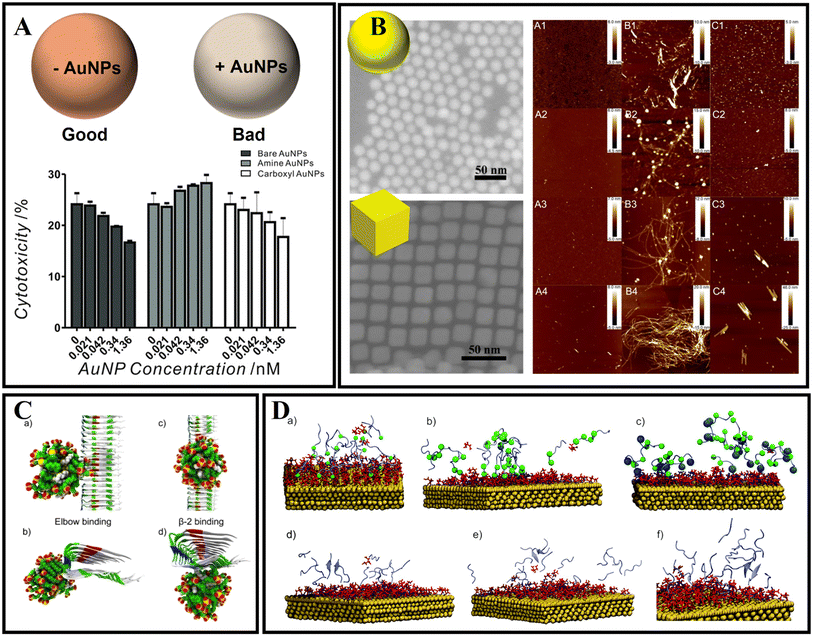 |
| Fig. 8 (A) Cytotoxicity of the end-point products of Aβ fibrillization incubated with and without bare, amine-conjugated, and carboxyl-conjugated AuNPs. Bare and negatively charged AuNPs both could effectively inhibit Aβ fibrillization and disaggregate Aβ fibrils and spherical oligomers compared with positively charged AuNPs.157 (B) AFM images (5 × 5 μm2) of the aggregates of Aβ40 (A1–4), Aβ40 and AuNS (B1–4), and Aβ40 and AuNC (C1–4) systems at different incubation times: 12 h (A1, B1, and C1), 24 h (A2, B2, and C2), 48 h (A3, B3, and C3), and 72 h (A4, B4, and C4).158 (C): (a) and (b) the elbow binding of a 2 nm 70% MUS-30% OT AuNP on the protofibril; (c) and (d) the β-2 binding as seen from the top and front of the fibrils.160 (D) Snapshots of MD simulations of amyloid peptides (purple) and gold surfaces (gold) covered with a citrate layer (red). (a) GNNQQNY peptide monomers bound to the gold surface. The terminal glycine (green ball) illustrated the favored N-terminal binding of the peptide to the citrate-stabilized gold nanoparticle surface. (b) NNFGAIL peptide monomers bound to the citrate-stabilized gold surface with the asparagine residues shown as green balls (N-terminus and position 2) to illustrate the N-terminal binding of the peptide. (c) VQIVYK peptide monomers (valine residues at the N-terminus and position 5 shown as green balls) at the gold surface with the C-terminal lysine (blue ball). The positively charged lysine at the C-terminus leads to binding of the peptide to the surface via both the N-terminus and the lysine side chain. The peptide monomers (VQIVYK) form parallel (d and e) and antiparallel (f) aligned dimers in solution and after binding to the gold surface.161 | |
The interaction mechanism between the surface of gold nanoparticles and Aβ fibrils also needs to be studied with MD simulations. As shown in Fig. 8C, the AuNPs can interact with the amino-acid sequence of 31IIGLMVGGVVI41.160 After 10 ns, the AuNPs can move along the region of the β-sheet. Amino acids including Ile31, Gly33, Met35, Gly37, Val39, and Ile41 in Aβ fibrils were involved in binding with AuNPs. John's group also investigated the influence of AuNPs on peptide aggregation by studying the amyloid model peptides (Fig. 8D).161 They designed citrate-modified AuNPs and used MD simulations to confirm the structure-forming properties of the citrate-gold surface. They found that peptide monomers presented favored N-terminal adsorption to the surface of citrate-modified AuNPs by electrostatic attraction. Based on MD simulations, it was concluded that the initial contact of charged groups with the gold surface resulted in a local elevation and alignment of peptide monomers on the surface.
Besides studying citrate-modified AuNPs, biomolecular functionalized AuNPs have also been investigated. Scutellaria barbata leaf extract mediated AuNPs and mimosine functionalized AuNPs have also been identified to suppress AD-related β-amyloid aggregation and neuronal toxicity.162,163 However, the interactions between AuNPs and Aβ are typically nonspecific, and thus it is a great challenge to specifically target Aβ by using AuNPs. In addition, most studies have only focused on the simple surface–interface interactions between Aβ and AuNPs, the potential function needs to be deeply tapped. Therefore, Xiong et al.164 designed a kind of dual peptide coupled AuNPs. As one of the functional peptides, the VVIA (Aβ39–42) fragment can specifically target Aβ and efficiently reduce Aβ-induced toxicity by generating nontoxic heterooligomers. Meanwhile, LPFFD can efficiently interact with the KLVFFAE of the central hydrophobic cluster of the Aβ sequence. As a result, the inhibition ability of the corresponding peptide@AuNPs against Aβ aggregation and cytotoxicity is greatly improved. Thereafter, the dual peptide modified AuNPs (VVIACLPFFD (VCD10)@AuNP) are the most effective in inhibiting Aβ oligomerization and the cytotoxicity caused by the aggregation species.
4.2 Gold nanoclusters
Unlike AuNPs, gold nanoclusters (AuNCs) with a core size below 2 nm consist of a few to several hundred Au atoms.165 Thanks to their unusual properties, including strong photoluminescence, significant Stokes shift, good biocompatible, and biodegradation characteristics, AuNCs have been applied to disease-related diagnosis and treatment.165 Especially as an innovative nanomedicine, AuNCs also have significant promise in amyloid-related disease applications.
As shown in Fig. 9A, Gao et al.166 reported nanoclusters (AuNCs) for the inhibition of amyloid aggregation. The authors prepared L-glutathione stabilized AuNCs and found that AuNCs with smaller sizes could completely inhibit amyloid aggregation and efficiently prevented Aβ from aggregation to larger oligomers, thus avoiding nucleation to form fibrils. As shown in Fig. 9B, Shi et al.167 designed a novel dual-responsive “cage metal chelator” release system based on AuNCs for non-invasive remote control to promote clioquinol (CQ) release and solubilize Aβ deposition. As a redox- and temperature-sensitive molecule, arylboronic esters were utilized to modify AuNCs for functionalized AuNCs. Therefore, the arylboronic ester-modified AuNCs could serve as a delivery system for H2O2-responsive controlled release. In addition, AuNCs possess a high near-infrared absorption and can further enhance the release of chelators under NIR light. As a result, this system can effectively inhibit Aβ aggregation and protect neurons from Aβ-reduced toxicity. Moreover, the photothermal effect of AuNCs can also serve as an effective means to dissolve Aβ amyloid deposits. Zhang et al.168 reported one type of Cys–Arg (CR) dipeptide modified Au nanocluster (Au23(CR)14) that was able to effectively dissolve pre-formed Aβ fibrils into monomers and recover the natural unfolded state of Aβ peptides from misfolded β-sheets (Fig. 9C). In addition, Au23(CR)14 was able to cross the BBB and cleared endogenous Aβ plaques in the brain of transgenic AD model mice. However, the interactions between traditional AuNCs and Aβ are also typically nonspecific, and thus it is also a great challenge to specifically target Aβ by using AuNPs. Recently, Hao et al.169 used a peptide fragment (CLVFFA) to modify AuNCs (AuNCs-CLVFFA) and CLVFFA could target binding the central hydrophobic region LVFFA of Aβ (Fig. 9D). Because the LVFFA is the central hydrophobic fragment of Aβ and can inhibit the aggregation of Aβ, AuNCs-CLVFFA was able to effectively inhibit Aβ aggregation and prolongation and disaggregate mature fibrils. Moreover, AuNCs-CLVFFA inhibited the transformation of Aβ from a random coil to a β-sheet structure.
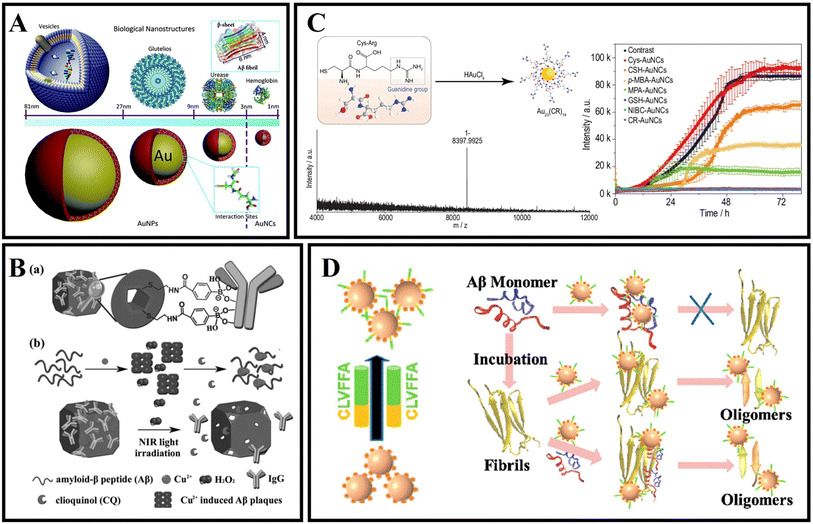 |
| Fig. 9 (A) Biomolecule-modified AuNPs and AuNCs to simulate different size biological entities to study the size effect of bio-nanointerfaces when they interact with Aβ.166 (B): (a) Illustration of IgG capped AuNC (AuNC-IgG). (b) H2O2-fueled and photothermal-responsive release of CQ from AuNC-IgG. CQ can chelate Cu2+ to disaggregate amyloid-β peptide (Aβ) plaques and inhibit H2O2 production.167 (C) Synthesis of CR-AuNCs and characterization of CR-AuNCs by ESI-MS and fibrillation kinetics for 20 μmol L−1 Aβ40 in the absence or presence of 25 mg L−1 Cys-AuNCs, CSH-AuNCs, p-MBA-AuNCs, MPA-AuNCs, GSH-AuNCs, NIBC-AuNCs or CR-AuNCs.168 (D) AuNCs-CLVFFA inhibited Aβ40 aggregation and prolongation, and disaggregated mature fibrils.169 | |
We can also imagine the future development of functionalized AuNCs for amyloid aggregation-related diseases. With the deepening of research, we expect versatile AuNCs to become an essential platform for AD research.
4.3 Metal oxide nanoparticles
Metal oxide nanoparticles such as CeO2 NPs, ZnO NPs, CuO NPs, and Fe3O4 NPs have a variety of functional properties such as UV-barrier, antimicrobial, antioxidative, catalytic, and magnetic properties.170,171 Therefore, they have been extensively used in the field of drug delivery, disease diagnosis, disease treatment, and enzyme immobilization.172 Among them, CeO2 NPs, ZnO NPs, and Fe3O4 NPs have also been researched in amyloid aggregation-related neurodegenerative disorders.
Due to their nontoxic nature, excellent biocompatibility and significant antioxidant activity at physiological pH values, cerium oxide nanoparticles (CeO2 NPs) have been given special attention.173 In addition, CeO2 NPs have both superoxide dismutase (SOD) mimetic activity and catalase mimetic activity by the Ce3+/Ce4+ valence transition, which also provides CeO2 NPs with an extra antioxidant function.174 Recently, CeO2 NPs have been used to protect neuron cells from Aβ-induced damage and treat neurocentral disease. In addition, CeO2 NPs can cross the BBB. Therefore, CeO2 NPs can be a promising candidate for treating AD. Recently, Li et al.173 designed a novel double delivery platform, which combined the advantages of controlled-release systems with those of glucose-coated CeO2 NPs (G-CeO2NPs). G-CeO2NPs could specially release the CeO2NPs and Cu2+ chelators by H2O2 stimulation. Therefore, the G-CeO2 NPs possess anti-aggregation properties and anti-oxidation properties. In addition, Li et al. adopted mesoporous silica nanoparticles as the carrier vehicles for loading G-CeO2NPs and 5-chloro-7-iodo-8-hydroxyquinoline. The research result showed that G-CeO2NPs could effectively inhibit Aβ aggregation, decrease cellular ROS and protect neurons from Aβ-induced toxicity. Guan et al.174 designed a bifunctional nanozyme (namely CeONP@POMs) by coating CeONP with POMs. The authors found that CeONP@POMs effectively inhibited Aβ aggregation, degraded Aβ aggregates, and reduced ROS levels. Moreover, CeONP@POMs is able to cross the BBB, regulate microglia, and protect neuronal cells from Aβ-related cytotoxicity. Coincidentally, a multifunctional AD therapeutic system, namely CeNP@MnMoS4, was designed and used to maintain metal ion homeostasis, reduce oxidative stress levels, and promote cell differentiation.175 Furthermore, due to the SOD activity, CeNP@MnMoS4 can protect cells from oxidative stress. Based on the catalase and superoxide dismutase activity of CeO2 and the hot electrons produced by gold nanorods, Ge et al.176 designed dumbbell-shaped nanocomposites (Au-CeO2) by coating both ends of gold nanorods with CeO2 NPs, and endowed Au-CeO2 with photocatalysis and photothermal effects in the NIR (Fig. 10A). To further improve the therapeutic efficiency of Au-CeO2, the authors used Aβ-targeted peptides (KLVFF) to modify Au-CeO2 and obtained an Aβ-targeted nanocomposite (K-CAC). The related results exhibited that K-CAC could improve the cognitive function of AD mice.
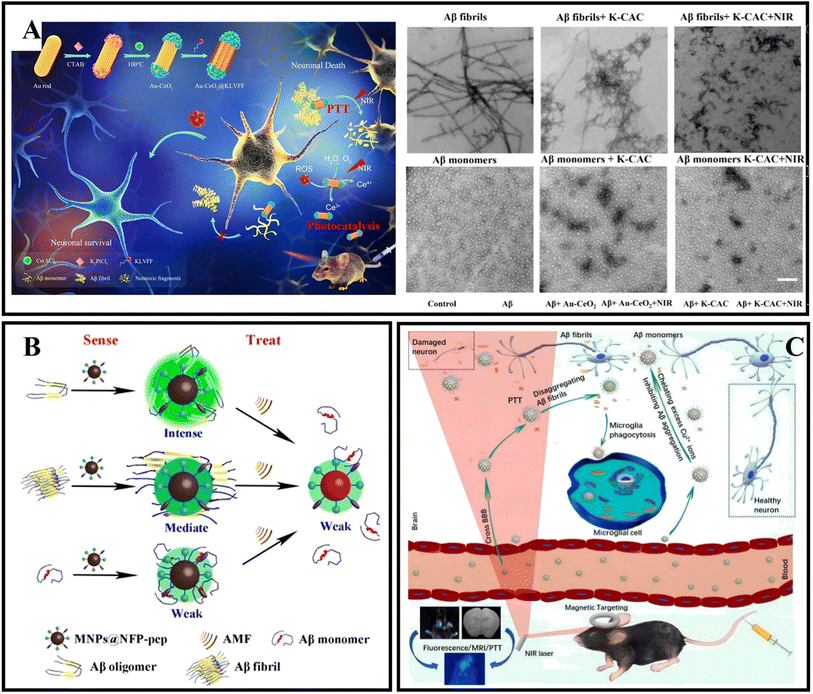 |
| Fig. 10 (A) Au-CeO2 exert antioxidant stress and target inhibition of Aβ through photocatalysis and the photothermal effect.176 (B) MNP@NFP-pep-based “sense and treat” system.180 (C) The B-FeCN nanosystem as a multifunctional nanocaptor with high BBB permeability to capture superfluous Cu ions and inhibit Aβ aggregation for magnetic targeting phototherapy.18 | |
As a type of magnetic nanoparticles (MNPs), iron oxide nanoparticles (IONs) are considered promising materials due to their high biocompatibility, unique magnetic properties, and ability to function as multimodal contrast agents.177,178 In addition, IONs have potential high affinity for circulating Aβ forms to induce a “sink effect” and potentially ameliorate AD.179 Mahmoudi et al.178 found that lower concentrations of superparamagnetic iron oxide nanoparticles (SPIONs) inhibited fibrillation, while higher concentrations increased the rate of Aβ fibrillation. And it was evident that the positively charged SPIONs could promote fibrillation compared with negatively charged or uncharged SPIONs. Currently, the surface functionalization of nanoparticles by using chemical methods is becoming more and more popular. Qu's group designed a multi-functional nanosystem (MNP@NFP-pep) by modifying a naphthalimide-based fluorescent probe and KLVFF peptide on the surface of magnetic nanoparticles, which can both specifically detect Aβ oligomers and achieve the wireless deep magnetothermally mediated disaggregation of Aβ aggregates with an alternating magnetic field.180 MNP@NFP-pep can interact with the exposed hydrophobic residues of Aβ oligomers based on π–π stacking and hydrophobic interaction (Fig. 10B). MNP@NFP-pep was able to specifically target Aβ aggregates and break down Aβ aggregates. Recently, our group presented drug-based magnetic imprinted nanoparticles (MINs@EGCG) combined with epigallocatechin-3-gallate (EGCG) and magnetic nanoparticles.19 MINs@EGCG exhibited triple functions for amyloid inhibition, drug delivery and fiber separation under an external magnet. MINs@EGCG inhibited the formation of amyloid fibrils with a high efficiency for 80%. Moreover, with the help of an external magnetic field, the cleaning efficiency is up to 80%. In addition, Halevas et al.181 prepared a nanocarrier (MMSNPs) by the sol–gel method using a magnetic core of Fe3O4 and a mesoporous silica shell and modified the flavonoid quercetin on the surface of MMSNPs for obtaining QCMMSNPs. QCMMSNPs exhibited potential anti-amyloid and antioxidant abilities. Moreover, QCMMSNPs reduced Aβ-induced cellular toxicity and minimized Aβ-induced ROS generation. Recently, Dyne et al.182 found that mild magnetic nanoparticle hyperthermia could destroy mature Aβ fibers by local heat and facilitate the phagocytic clearance of Aβ as well as attenuating pro-inflammatory responses by microglial cells. As shown in Fig. 10C, Gong et al.18 reported an intelligent nanosystem (B-FeCN) by modifying carbon nitride nanodots and benzothiazole aniline on the surface of Fe3O4@mesoporous silica nanospheres. Among them, B-FeCN effectively traps excessed Cu2+ and inhibits the formation of Cu2+–Aβ complexes. In addition, B-FeCN generated local heat to promote the depolymerization of fiber precipitates. Interestingly, the BBB permeability of B-FeCN was significantly improved under NIR irradiation. Thanks to the advantages of the Fe3O4 cores, B-FeCN entered the brain and targeted the Aβ region with the help of a magnetic field. Benzothiazole aniline (BTA) makes B-FeCN a detection agent for specifically targeting Aβ plaques and imaging the Aβ species by fluorescence. However, B-FeCN has a certain biological toxicity, and the research on the metabolic mechanism in vivo is not perfect, which hinders further applications.
4.4 Organic and inorganic quantum dots
Therapeutic agents should be completely cleared from the body in a reasonable time, and usually, effective renal and hepatic clearance requires drugs less than 10 nm, and the development of nanoparticles with excellent biocompatibility is of great importance.183
Sun et al.184 prepared BPQDs with excellent NIR photothermal properties and biocompatibility using the liquid phase exfoliation method. The size distribution of the prepared BPQDs was only 2.6 nm. BPQDs were conjugated with PEG and exhibited high stability in the physiological medium and low toxicity for different cell types. More importantly, BPQDs induced the death of C6 and MCF7 cancer cells under NIR illumination, indicating that the BPQDs have great potential as photothermal agents with implications for the treatment of amyloid-related diseases. Wang et al.185 found that BPQDs at 100 ng mL−1 inhibited insulin aggregation and disaggregated mature fibers, and the inhibitory effect persisted through all stages of insulin aggregation (Fig. 11A). Molecular dynamics simulations showed that BPQDs could stabilize the α-helix structure of insulin and reduce the β-sheet content. Bu et al.186 reported using BPQDs as a photoactive material and heme as an electron acceptor sensor to monitor the Aβ protein content, and these properties make BPQDs a promising candidate for the treatment of amyloidosis and neurodegenerative disease.
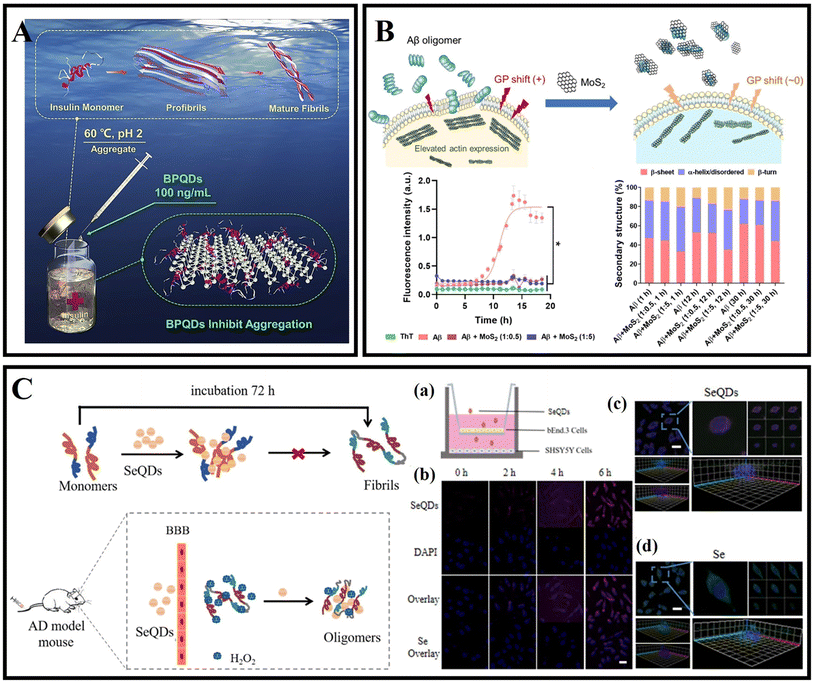 |
| Fig. 11 (A) Amyloid aggregation of insulin in the absence and presence of BPQDs.185 (B) Cell membrane disruption by Aβ oligomers and its rescue by ultrasmall MoS2 QDs. ThT fluorescence kinetic assay and attenuated total reflection (ATR)-FTIR indicated the inhibitory effects of ultrasmall MoS2 QDs on Aβ.190 (C) Se QDs for dissociating Aβ fibrils and crossing the BBB. (a and b) Transwell experiment and cellular uptake. Scale bar, 25 μm. (c and d) 3D SH-SY5Y cell fluorescence image. Scale bar, 30 μm.194 | |
Molybdenum disulfide quantum dots (MoS2 QDs) have been widely used for live bioimaging and nanomedicine because of their low toxicity, excellent cell permeability and biocompatibility, and strong luminescence properties.187,188 Sun et al.189 used a one-pot hydrothermal method to synthesize cysteamine functionalized MoS2 QDs, which effectively inhibited the fibrillation and destabilized preformed fibrils of bovine serum albumin in a concentration-dependent manner. Li et al.190 observed cell membrane perturbation and actin reorganization, which were induced by Aβ oligomers. Further research revealed that the ultra-small MoS2 QDs restored membrane fluidity and inhibited Aβ amyloid aggregation (Fig. 11B). Based on the calculation of discrete molecular dynamics simulations, it was found that MoS2 QDs were bound to the N-terminal of Aβ peptides through hydrophilic interactions. In addition, surface-coated Aβ oligomers by MoS2 QDs could not further associate with cell membranes. Tian et al.191 pointed out the promising application of MoS2 QDs in photodynamic therapy. MoS2 QDs promote the creation and separation of electron–hole pairs more effectively than MoS2 nanosheets. Therefore, MoS2 QDs are able to generate a variety of ROS under illumination. Results related to MoS2 QDs broaden the application of molybdenum disulfide-based nanomaterials.
As drugs or nanocarriers, selenium nanoparticles have made important progress in cancer, AD and other diseases because of their excellent physicochemical characteristics.192 It has been reported that selenium nanoparticles have a high affinity for Aβ, which can inhibit Aβ aggregation and treat AD as a potential nanomedicine.193 As shown in Fig. 11C, Guo et al.194 synthesized selenium quantum dots (Se QDs), which could quickly penetrate the BBB because of their ultrasmall size and excellent biocompatibility. Se QDs had a strong free-radical scavenging activity and could protect cells from oxidative stress damage. Se QDs could not only inhibit Aβ aggregation and reduce Aβ-mediated cytotoxicity, but also effectively reduce tau protein phosphorylation, further improve oxidative stress, and maintain nerve cell stability. In conclusion, Se QDs had great advantages compared with traditional single-target drugs in the treatment of AD.
4.5 Carbon-based zero-dimensional nanomaterials
Carbon nanomaterials are widely used to inhibit Aβ aggregation due to the various surface and interface interactions between the Aβ peptide and carbon nanomaterials.34 Carbon dots (CDs), as a new type of carbon-based zero-dimensional nanomaterial, have attracted extensive research in recent years because of their low cost, easy synthesis, good biocompatibility, photoluminescence, easy surface modification, and high stability.195 It's important to note that CDs include graphene quantum dots (GQDs), carbide polymer dots (CPDs), and carbon quantum dots (CQDs).196
GQDs are single- or few-layered graphene sheets of 10 nm or less in size.197 Most CDs possess size-dependent auto-fluorescence originating from quantum confinement and edge effects, compared with carbon nanotubes, fullerenes, and graphene nanomaterials.198 According to previous studies, GQDs have a good ability to cross the BBB, effectively modulate the Aβ aggregation process and reduce Aβ-induced neurotoxicity.95 Therefore, GQDs are often combined with Aβ aggregation inhibitors or neuroprotective peptides to enhance efficacy. In 2015, Liu et al.199 prepared GQDs by a hydrothermal method, demonstrating that GQDs effectively inhibited Aβ42 peptide aggregation (Fig. 12A). Moreover, Xiao et al.200 prepared a novel nanomaterial GQDG by conjugating GQDs with glycine–proline–glutamate (Gly–Pro–Glu). In vitro assays proved that both GQDs and GQDG could inhibit the aggregation of Aβ42. In vivo assays indicated that GQDG enhanced AD model mice's learning and memory capacity, increased dendritic spine amounts, and decreased several pro-inflammatory cytokine content. Subsequently, several studies reported the application of nitrogen-doped graphene quantum dots (N-GQDs) and fluorine-functionalized graphene quantum dots (FGQDs) in amyloid aggregation.201,202 Liu et al.203 covalently combined GQDs with tramiprosate to design a novel Aβ aggregation inhibitor, namely GQD-T. GQD-T showed the capability of inhibiting Aβ aggregation and rescuing Aβ-induced cytotoxicity due to the synergistic effect of the GQDs and tramiprosate (Fig. 12B). Moreover, GQDs can effectively disperse mature amyloid-rich Staphylococcus aureus biofilms and interfere with the self-assembly of amyloid fibers.204 Liu et al.205 studied the regulatory effects and mechanism of GQDs on Aβ42 aggregation and found that electrostatic interaction was the major driving force in the co-assembly process of Aβ42 and GQDs. Tak et al.206 used Clitoria ternatea as a precursor with the help of a one-pot microwave-assisted method to prepare novel graphene quantum dots ctGQDs. The transport efficiency of ctGQDs across the BBB was increased significantly and showed high inhibition efficiency of the acetyl cholinesterase enzyme. Meanwhile, Perini et al.207 reviewed the potential of GQDs in biomedicine and neuroscience and discussed the ability of GQDs to cross the BBB and reach the brain. Ghareghozloo et al.208 studied the inhibiting effect of graphene oxide quantum dots (GOQDs) on bovine insulin and hen egg white lysozyme (HEWL) aggregation. GOQDs were prepared through pyrolysis of citric acid, and the reduction step was carried out using ascorbic acid. The results showed that GOQDs could inhibit the related protein fibrillation, and the presence of reduced GOQDs was found to promote protein assembly via shortening the nucleation phase. The content of oxygen-containing functional groups from the GOQD surface may be the key factor in affecting fibrillation (Fig. 12C).
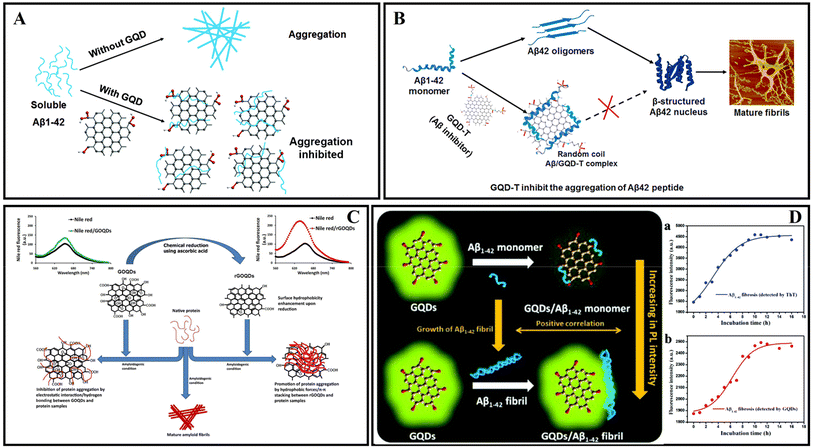 |
| Fig. 12 (A) The GQDs used for inhibiting the aggregation of Aβ42 peptides.199 (B) GQD-T inhibits the aggregation of Aβ42 peptides.203 (C) GOQDs have the capacity to inhibit the fibrillation of protein.208 (D) Proposed mechanism for the different fluorescence behaviors of GQDs on Aβ42 monomers and fibrils.209 | |
The detection of the concentration of amyloid monomer is of great importance in diagnosing AD. Huang et al.209 proposed a method to detect Aβ monomer concentration using the fluorescent properties of GQDs (Fig. 12D). The positively charged groups, the aromatic structure and moieties with hydrogen bonding ability on Aβ42 monomers provided suitable conditions for the interaction between Aβ42 monomers and GQDs. This strong combination promoted the excited-state electron transfer from GQDs to Aβ42, resulting in quenching of the PL intensity of GQDs. The Aβ fibers consume abundant interaction sites and contact surface areas through a self-assembly process, and the interaction between Aβ fibers and GQDs is much weaker to quench GQD fluorescence. Yousaf et al.210 reported the detection of monomers and oligomers using specific fluorescence and a magnetic resonance imaging (MRI) multimodal probe based on bovine–serum–albumin-capped fluorine functionalized GQDs (BSA@FGQDs). BSA@FGQDs could monitor amyloid fibrillation and was more sensitive than conventional ThT stain. Monitoring amyloid aggregation dynamics and monomers/oligomers using BSA@FGQD probes is based on hydrophobic, electrostatic, hydrogen bonding, and π–π stacking interactions. Tang et al.211 examined the influences of GQDs on the obstruction of the membrane axis of Aβ in its three forms of monomers (Aβ-m), oligomers (Aβ-o), and amyloid fibrils (Aβ-f), and demonstrated the mitigation potential of GQDs in reverting SH-SY5Y cells to their native fluidic state. It was found that Aβ-m is bound to the GQDs via strong electrostatic and hydrophobic interactions. The nanostructures reshaped the potential of mean force (PMF) of Aβ-o to inhibit the β-sheet propensity of the peptide residues, and GQDs adhered to the sides and ends of an Aβ-f, thereby hindering their elongation.
CQDs are a new class of 0D carbonaceous nanomaterials with a diameter less than 10 nm.212 CQDs can be produced using diverse bioorganic compounds through solvent-free pyrolysis, hydrothermal treatment, or microwave treatment. These treatment methods and bioorganic compounds allow for the synthetic flexibility of CQDs without intricate set-ups.213 CQDs have outstanding features such as low cost, easy synthesis, excellent biocompatibility, and photoluminescence.212 The absorption and emission spectra of CQDs can be tuned by adjusting the precursor type, preparation method, degree of carbonization, surface state, and element doping.214 In addition, CQDs have abundant functional groups, such as hydroxyl, amino, and carboxyl groups, which are easy to modify.215 Moreover, CQDs can interact with Aβ peptides and aggregates through electrostatic, hydrogen bonding, π–π stacking, and hydrophobic interactions.15,216
Many studies have reported the inhibition of human insulin fibrosis by using carbon dots.217,218 Malishev et al.219 prepared enantiomeric carbon dots (L-Lys-C-dots and D-Lys-C-dots) using L-lysine or D-lysine. The results demonstrated that L-Lys-C-dots exhibited higher affinity to Aβ42 (either monomeric and/or pre-fibrillar species) compared with D-Lys-C-dots, modulated the fibril assembly process of Aβ42 (Fig. 13A). The authors speculated that the different properties of L-Lys-C-dots and D-Lys-C-dots were caused by residual lysine moieties which exposed to the C-dots’ surface and residual lysine possibly interfered with the electrostatic interactions of the peptide. Zhou et al.15 used o-phenylenediamine and citric acid as precursors to synthesize amphiphilic yellow-emissive CDs (Y-CDs) by an ultrasonication-mediated methodology. The amphiphilicity of Y-CDs didn't change with different coatings. In addition, it was proved that Y-CDs could cross the BBB of zebrafish via passive diffusion. The related research suggested that Y-CDs could inhibit the overexpression of APP and Aβ peptides. Koppel et al.220 used brown coal to prepare novel CQDs. CQDs were able to inhibit IAPP and Aβ aggregation induced by lipopolysaccharide (LPS) through hydrogen bonding and hydrophobic interactions. This study contributed to understanding the pathological link between bacterial metabolites and amyloid diseases. Due to the excellent antioxidant capacity of selenium nanoparticles, Zhou et al.221 designed selenium-doped carbon quantum dots (Se-CQDs) via a simple hydrothermal treatment of selenocystine, which were successfully applied to inhibit Aβ aggregation and scavenge the redundant ROS in the brain (Fig. 13B). Se-CQDs maintained the intrinsic properties of both selenium and CQDs. Se-CQDs have paired α-carboxyl and amino groups at their edges, which trigger multivalent interactions with Aβ. Li et al.222 fabricated Se-CQDs using selenocysteine through hydrothermal treatment under mild conditions. ROS could be effectively scavenged by the Se-CQDs. Once Se-CQDs are internalized into cells, high levels of ROS in cells are reduced. These properties enable Se-CQDs to protect biological systems from oxidative stress. Guerrero et al.223 used Na-citrate as a precursor to prepare CQDs. Pulse-chase lysozyme fibril-forming assay and ThT fluorescence showed that CQDs prevented the monomers and oligomers into mature fibrils, while could provoke the disaggregation of mature HEWL fibrils. Li et al.224 fabricated ultra-small CQDs with a uniform size by pulsed laser ablation. Results demonstrated that CQDs could efficiently inhibit Aβ42 aggregation. Moreover, the quenching of tyrosine and ANS fluorescence of the Aβ42 solutions with CQDs indicated that there existed an interaction between the CQDs and Aβ42 peptides. Our group prepared glycosylated carbon dots (g-CDs) using glucose as a precursor. gCDs-E has been prepared by self-assembly of gCDs and epigallocatechin-3-gallate (EGCG). gCDs-E could not only suppress the fibrillation of Aβ and disaggregate Aβ fibrils, but also effectively inhibit the activity of Candida albicans.13 In addition, the capability of gCDs-E for BBB penetration was also observed using a normal mice model.
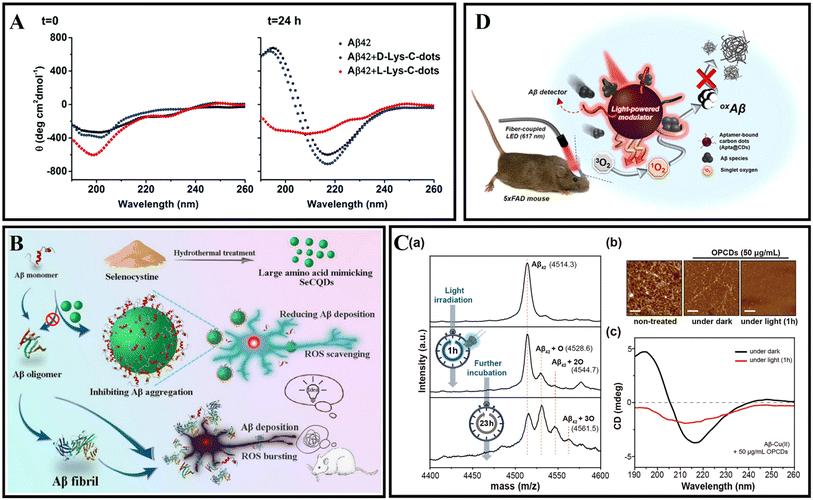 |
| Fig. 13 (A) Secondary structures of 25 mM Aβ42 monitored by CD spectroscopy in the absence or presence of D-Lys-C-dots or L-Lys-C-dots.219 (B) Synthesis of SeCQDs and the inhibition effects of SeCQDs on Aβ aggregation and ROS production.221 (C): (a) The mass spectra of Aβ–Cu(II) aggregates incubated with OPCDs for 24 hours at 37 °C. Light was irradiated only for one hour. (b) AFM images and (c) CD spectra of Aβ–Cu(II) aggregates incubated with OPCDs under dark and one-hour-light illuminating conditions.232 (D) Aβ-targeting, CD-mediated photodynamic modulation for spatiotemporal inhibition of Aβ aggregation in vivo.229 | |
As a highly active substance, ROS can be used as a disease treatment agent.225 At present, there are many research studies about photodynamic therapy for tumor diseases.226 In addition, photodynamic therapy also has good application potential in amyloid-related diseases.227 The band-to-band transition of CDs' electron carriers generates ROS through an electron- (type I) or energy-transfer (type II) process, mediating photo-modulation to denature target biotoxins.228,229 Like a type II photosensitizer, CQDs can react with oxygen after absorbing energy, promote the production of singlet oxygen, and oxidize the amino acid residues of Aβ peptides, thereby destroying the interaction between peptides.230
For example, Chung et al.231 synthesized CDs using ammonium citrate through one-pot hydrothermal treatment and obtained branched polyethylenimine modified CDs (bPEI@CDs) by passivating the surface of the prepared CDs using branched polyethylenimine. bPEI@CDs exhibited hydrophilic and cationic surface properties, which could effectively interact with negatively charged residues of Aβ peptides. Under light illumination, bPEI@CDs displayed a strong effect on Aβ aggregation and on the disaggregation of fibrils by generating ROS. Building on previous work, Chung et al.232 prepared multifunctional carbon-dots (OPCDs) using o-phenylenediamine. The N-containing polyaromatic surface of OPCDs is the reason why the self-assembly of Cu(II)-Aβ is hindered, thereby weakening Cu(II) catalyzed oxidative stress and Aβ aggregation propensity. Illumination treatment further enhanced the inhibitory effect of OPCDs, which produced ROS to oxidize the key residues (His and Met) of Aβ (Fig. 13C). Recently, Chung et al.229 designed Aβ-targeted, red light-responsive apta@CD based on previous work, which inhibited Aβ aggregation in space and time and reduced the overall Aβ burden in the brain. Under red light, apta@CDs effectively inhibited the formation of Aβ aggregates by oxidizing Aβ residues, exhibiting a light-modulating effect on Aβ aggregation (Fig. 13D). The application of apta@CDs to 5xFAD mice further demonstrated the anti-amyloid aggregation ability of apta@CDs in vivo.
CPDs have also been reported for AD diagnosis and treatment. Gao et al.233 prepared multifunctional nitrogen-doped CPDs by using o-phenylenediamine for targeting Aβ aggregations. CPDs inhibited Aβ fibrillation and disaggregated Aβ fibrils through electrostatic interactions, hydrogen bonds, and hydrophobic interactions. CPDs could emit enhanced red fluorescence upon interaction with Aβ fibrils, clear amyloid plaques in vivo and prolong the lifespan of CL2006 strain by alleviating Aβ-induced toxicity. C60 has been demonstrated to interact and prevent Aβ fibrillation. However, there are significant problems such as low solubility and toxicity that need to be solved.
Fullerenes and their derivatives have been reported for use in amyloid diseases. Melchor et al.234 synthesized diethyl fullerenemalonates and the corresponding sodium salts using the Bingel reaction, adducts of C60 bearing 1 to 3 diethyl malonyl and disodium malonyl substituents (C60+n(COOR)2n, where n = 1–3 and R = –CH2CH3, –Na). The inhibition efficiency of bisadduct salts (C62(COONa)4) and trisadduct (C63(COONa)6) is 98% and 83% respectively. The 6.7 mM C62(COONa)4 mixture has been confirmed the capacities for anti-amyloid deposition. The anti-aggregation effect of C62(COONa)4 is mainly attributed to the hydrophobic surface and the number of substituents bound on the surface of fullerene. C60 acts as both a ROS producer under UV-visible light and a ROS scavenger in the dark.235 Du et al.236 designed UCNP@C60-pep nanoparticles, which generated ROS under NIR light and oxidized Aβ. Moreover, UCNP@C60-pep could also alleviate the excessive ROS in the organization. Both the ROS generation and ROS quenching abilities of UCNP@C60-pep were beneficial to reduce Aβ-induced neurotoxicity. Bobylev et al.237 studied the ability of water-soluble fullerene derivatives with different types of solubilizing addends for anti-amyloid aggregation. The three derivatives were found to exhibit strong anti-amyloid effects in vitro and low cytotoxicity in vivo. The fullerene derivatives have a strong anti-amyloid effect and low toxicity. Their ability for crossing the BBB and the inhibition ability of amyloid fibrillation make fullerenes potential drug candidates. Table 2 lists the mechanism and effect of carbon-based zero-dimensional nanomaterials on the modulation of amyloid aggregation.
Table 2 A list of carbon-based zero-dimensional nanomaterials with the modulation mechanism and effect of amyloid aggregation
Nanomaterials |
Modulation mechanisms |
Effects |
Ref. |
GQDs |
Adsorption/hydrophobic interactions |
Inhibition |
199
|
GQDG |
Adsorption |
Inhibition |
200
|
FGQDs |
Adsorption |
Inhibition/disaggregation |
201
|
GQD-T |
Adsorption/synergistic |
Inhibition |
203
|
ctGQDs |
Inhibition acetyl cholinesterase enzyme |
Treating disorganization of cells |
206
|
GOQDs |
Adsorption/hydrophobic interactions |
Inhibition |
208
|
BSA@FGQDs |
Hydrophobic/electrostatic/H-bonded/π–π stacking interactions |
Monitor |
210
|
L-Lys-C-dots |
Electrostatic interactions |
Modulation |
219
|
Y-CDs |
Amphiphilic |
Inhibiting overexpression of APP and Aβ peptides |
15
|
CQDs |
H-Bonded/hydrophobic interactions |
Inhibition |
220
|
Se-CQDs |
Adsorption/hydrophobic interactions/anti-oxidation |
Inhibiting Aβ aggregation and scavenging ROS |
221
|
Se-CQDs |
Anti-oxidation |
Scavenging ROS |
222
|
gCDs-E |
Hydrophobic interactions/anti-oxidation |
Suppressing fibrillation/disaggregation/inhibition fungi |
13
|
bPEI@CDs |
Electrostatic interactions/photooxygenation |
Inhibition/disaggregation |
231
|
OPCDs |
Chelation/hydrophobic interactions/photooxygenation |
Inhibition |
232
|
Apta@CDs |
Photooxygenation/target |
Inhibition |
229
|
CPDs |
Electrostatic interactions/hydrogen bonds/hydrophobic interactions |
Inhibition/disaggregation |
233
|
C60 |
Hydrophobic interactions |
Inhibition |
234
|
UCNP@C60-pep |
Photooxygenation/anti-oxidation |
Inhibition/scavenging ROS |
236
|
5 Others
In addition, other nanoparticles have also been reported for diagnosis and treatment of AD, including metal–organic frameworks (MOFs), polyoxometalates (POMs), liposomes, SiO2, upconverting nanoparticles and so on.238–241
5.1 Metal–organic frameworks
Metal–organic frameworks (MOFs) are crystalline entities composed of metal ions or clusters and polydentate organic ligands.242 As an emerging family of hybrid nanomaterials, MOFs have attracted much attention due to their porous structures, good biocompatibility, and tunable sizes, and are widely used in catalytic, sensing and biological applications.243–246
Wang et al.238 prepared NIR responsive nanoparticles PCN-224 for inhibiting Aβ aggregation. PCN-224 was hydrothermally synthesized by coordinating tetrakis(4-carboxyphenyl)porphyrin (TCPP) ligands with zirconium (Fig. 14A). Under NIR irradiation, PCN-224 significantly reduced Aβ induced cytotoxicity. The functional porphyrin linkers are separated by Zr clusters in the MOF framework, which could avoid the self-quenching of excited states, maintain the photo-oxidative properties of the porphyrin linkers, and improve the 1O2 generation capacity. Yu et al.247 selected four kinds of POMFs (Zr-MOF, Al-MOF, Ni-MOF, and Hf-MOF) for further investigation, which are stable under physiological conditions and exhibit excellent biocompatibility (Fig. 14B). It was found that Hf-MOF was the most efficient Aβ photooxidant based on the experimental results and DFT calculations. LPFFD modified Hf-MOFs not only effectively targeted Aβ peptides and reduced Aβ-induced cytotoxicity, but also improved photooxidation in complicated environments. Yan et al.248 synthesized a core–shell nanocomposite CeONP-Res-PCM@ZIF-8/PDA/Apt through an in situ encapsulation strategy. Resveratrol (Res), ceria nanoparticles (CeONPs) and PCM (tetradecanol) were embedded in a ZIF-8/PDA matrix by a water-based mild method (Fig. 14C). These nanocomposites can be activated to release the encapsulated Res upon NIR illumination through PCM regulation. Moreover, CeONP-Res-PCM@ZIF-8/PDA/Apt nanocomposites exhibited multifunctional effects on inhibiting Aβ aggregation, disaggregating Aβ fibrils, and decreasing Aβ-induced oxidative stress and neural apoptosis. The therapeutic effect of nanocomposites could be enhanced under NIR irradiation because of the excellent photothermal properties of PDA. In 2021, Zeng et al.249 built an electron-deficient MOF from the ligand of naphthalene diimide (NDI) and metal nodes of biocompatible Ca2+. Then pyrene as an electron donor molecule was encapsulated to form a host–guest MOF self-assembled co-crystal Py@Ca-NDI. A concomitant superior charge transfer interaction between pyrene and NDI could be attained and the photothermal conversion efficiency of Py@Ca-NDI in aqueous solution could reach up to 41.8%. The treatment of neurodegenerative disease by using MOF-based materials is a challenging study, and more elaborative studies on biostability, biocompatibility and BBB penetration are still needed.250
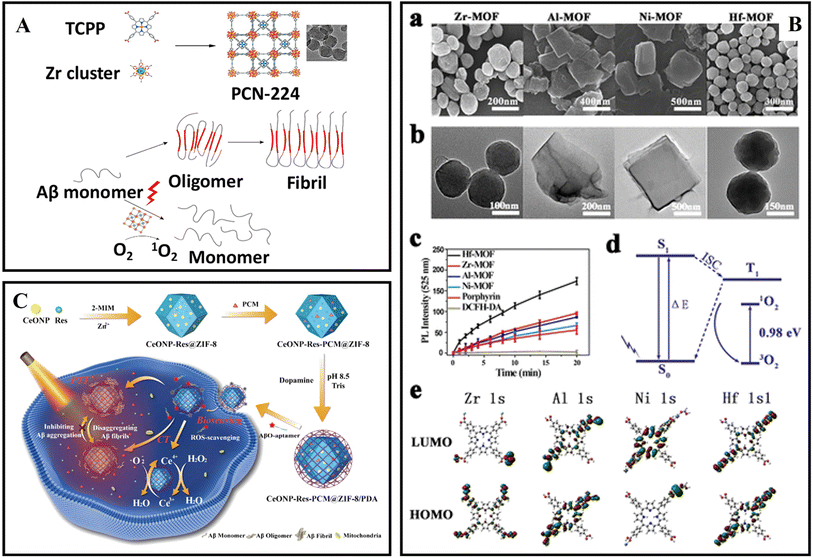 |
| Fig. 14 (A) The synthesis of PCN-224 nanoparticles and photo-inhibition of Aβ42 aggregation by using PCN-224 nanoparticles.238 (B): (a) SEM images. (b) TEM images of Zr-MOF, Al-MOF, Ni-MOF, and Hf-MOF, respectively. (c) Fluorescence intensity of DCF after photooxidation by using PMOFs at different time points. (d) Jablonski diagram describing the underlying photophysics and photochemistry of photodynamic therapy. (e) Electronic density contours for the frontier molecular orbitals of the four kinds of PMOFs.247 (C) CeONP-Res-PCM@ZIF-8/PDA preparation and its applications in Aβ oligomer sensing and treatment.248 | |
5.2 Polyoxometalates
Polyoxometalates (POMs) are a special group of inorganic redox-active materials consisting of multiple metal oxide ions linked together by oxygen atoms to form nanoclusters within an ordered three-dimensional framework.251 Due to the tunable structures, excellent physicochemical properties and good biocompatibility of POMs, many researchers have explored their application in biomedicine. POMs can act as Aβ aggregation inhibitors and can be seen as candidates for the treatment of AD because of their similarity to water-soluble fullerene derivatives.252
In 2011, Qu's group reported the inhibitory effect of POMs on Aβ aggregation and found that POMs with a Wells–Dawson structure had a better effect.239 Then Li et al.253 reported that POMs could not only inhibit Aβ aggregation but also photodegrade Aβ aggregates, such as Aβ oligomers. Meanwhile, Li et al.254 designed bifunctional nanoparticles POM@P through the self-assembly of Aβ15–20 peptides and POM (Fig. 15A). The aggregation process of Aβ was researched by monitoring the fluorescence of Congo red's after adding POM@P. Moreover, the prepared POM@P could effectively target amyloid aggregation in mouse cerebrospinal fluid. The interaction between POMs and Aβ species relies on an electrostatic effect. Gao et al.255 designed a variety of transition-metal-substituted POMds that had better inhibition efficiency of Aβ aggregation than POMs. Results demonstrated that POMds with histidine-binding sites could not only specifically target the polypeptide sequence (HHQK) of Aβ, but also show stronger inhibitory effects through enhancing binding affinity between Aβ and POMds (Fig. 15B). POMds-Dawson-Ni and POMds-Dawson-Co exhibited better effects for decreasing Aβ-haem peroxidase-like activity. In addition, POMds could across the BBB and were metabolized completely after 48 hours. Then Gao continuously designed artificial enzymes AuNPs@POMD-8pep that exhibited protease activities, SOD-like functionality, and metal-ion chelation capabilities (Fig. 15C).256 AuNPs facilitated electron transfer and served as a scaffold to create a coupled POMD-peptide compound.
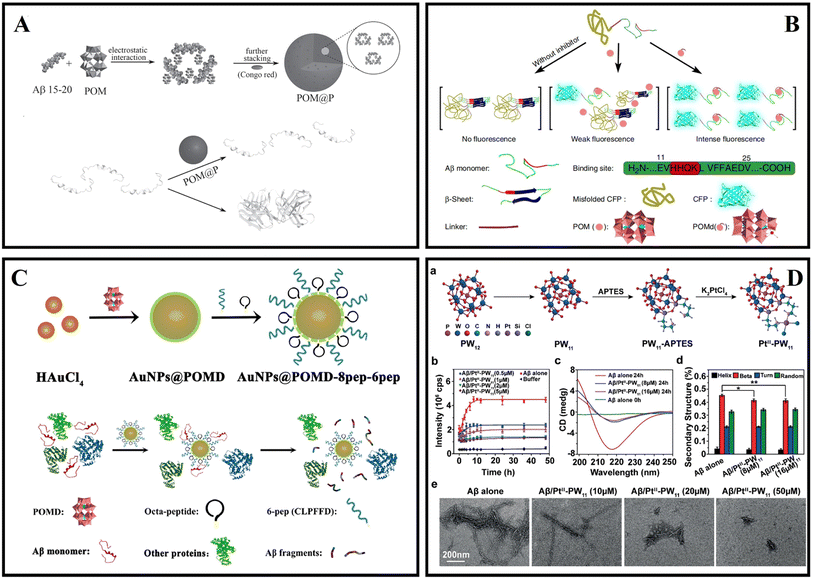 |
| Fig. 15 (A) The schematic illustration of self-assembly of Aβ15–20 and POM to hybrid spheres and the assembled peptide and POM nanoparticles can effectively inhibit Aβ1–40 aggregation.254 (B) The high-throughput screening method for identifying effective Aβ-aggregation inhibitors.255 (C) Synthetic route of the Aβ-targeted nanozyme and AuNPs@POMD-8pep-6pep acted as an Aβ targeted nanozyme to specifically hydrolyze Aβ.256 (D) Inhibition effect of PtII-PW11 on Aβ42 aggregation. (a) The synthesis of PtII-PW11. (b) Aggregation kinetics of Aβ42 without or with PtII-PW11 monitored by ThT fluorescence assay. (c) CD spectra and (d) secondary structure analysis of Aβ42 without or with co-incubation of PtII-PW11. (e) TEM images of Aβ42 without or with co-incubation of PtII-PW11 at 37 °C for 48 h.29 | |
Aβ fibrils and ROS are closely related to AD pathogenesis. The reduced POMs (rPOMs) had a strong NIR absorption and ability for anti-oxidant activity.257–259 Ma et al. designed a NIR-responsive rPOM-based agent rPOMs@MSNs@copolymer that consists of mesoporous silica nanoparticles (MSNs), rPOMs, and thermal responsive copolymer poly(N-isopropylacrylamide-co-acrylamide).252 The copolymer could melt under 808 nm irradiation and led to the release of rPOMs. Therefore, preformed Aβ fibrils could be disaggregated by local heat. Zhao et al. reported an organic platinum-substituted POM with a Kegging structure (Me4N)3[PW11O40(SiC3H6NH2)2PtCl2] (abbreviated as PtII-PW11) (Fig. 15D).29 The negatively charged PtII-PW11 anions could bind to the cationic cluster (HHQK) of Aβ through electrostatic interaction, and PtII-PW11 interacted with other residues through van der Waals force, hydrogen bonding and desolvation energy. PtII-PW11 also reduced Aβ42 aggregation-induced cytotoxicity. When the dosage reached 8 μM, cell viability increased from 49% to 67%. In 2022, Gao innovatively combined post-translational modification (PTM) technology with POMs, rationally designed and synthesized a Wells–Dawson POM-based PTM agent POMD-TZ (thiazolidinethione as TZ) for chemical modification of amyloid peptides.260 POMD-TZ could selectively bind to the Lys16 site, inhibit Aβ aggregation, and reduce the cytotoxicity caused by the Aβ peptide.
5.3 Liposomes
Liposomes have the advantages of non-toxicity, strong drug-carrying capacity, and ease of synthesis and modification, and have been widely used in the field of drug delivery.261,262
Gobbi et al.263 reported that nanoliposomes containing phosphatidic acid (PA) and cardiolipin (CL) targeted aggregated forms of Aβ42 fibrils (22–60 nM) with high binding affinity. Mourtas et al.240 successfully used click chemistry to decorate the surface of nanoliposomes with curcumin, and the curcumin-modified liposomes (maintaining the planarity) had extremely high affinity for Aβ42 fibers (1–5 nM) and had sufficient stability for in vivo applications. This high-affinity binding may be due to a multivalent interaction between click curcumin liposomes and Aβ. Taylor et al.264 designed and formulated different types of nanosized liposomes incorporating or decorated with curcumin, a curcumin derivative, or lipid ligands (PA, CL, or GM1 ganglioside), and then evaluated their ability to influence Aβ42 peptide aggregation based on ThT and a sandwich immunoassay. The results showed that the click-curcumin type was by far the most effective. Bana et al.265 prepared phosphatidic acid and ApoE-derived peptide bi-functionalized mApoE–PA–LIP. mApoE–PA–LIP strongly bound the Aβ peptide (kD = 0.6 μM), inhibited peptide aggregation and triggered preformed aggregates. The permeation rate across the BBB of mApoE–PA–LIP was 5-fold higher with respect to mono-functional liposomes. Papadia et al.266 developed multifunctional LUV liposomes (mf-LIPs) having three ligands, one of which is a curcumin-lipid ligand (TREG) and the other two ligands target the transferrin and the LDL receptors of the BBB. Further research found that the multiple ligands of mf-LIPs did not interfere with each other, and mf-LIPs have multiple functions such as targeting the BBB and inhibiting amyloid aggregation. Meanwhile, in vivo experiments found that the curcumin ligand increases the stealth properties of liposomes by reducing their uptake by the liver and spleen.267 Kuo et al.268 designed a drug carrier system of ApoE-modified liposomes conjugated with PA. This system was used to improve BBB penetration and release quercetin (QU) and rosmarinic acid (RA) to inhibit Aβ42. ApoE–QU–RA–PA–liposomes could penetrate the BBB because of strong attraction between low-density lipoprotein receptors and ApoE.
5.4 SiO2
Silica nanostructures, due to their synthetic flexibility, molecular properties, multifunctionality, and biocompatibility, have long been used in biomedical applications.269,270
In 2016, Hulsemann et al.241 reported a highly stable standard in the size range of native Aβ oligomers consisted of a silica nanoparticle, which is functionalized with Aβ peptides on its surface (Aβ-SiNaP). The detection limit corresponded to an Aβ concentration of 1.9 ng L−1. Zhang et al.271 synthesized β-NaYF4:Yb/Er@SiO2@RB by combining upconversion nanoparticles (UCNPs) with photosensitizers to disaggregate the preformed Aβ aggregates under NIR light. UCNPs were able to transfer energy to RB at 980 nm and Aβ42 fibrils were disaggregated via photo-induced ROS. Jung et al.30 designed Aβ nanodepletors consisting of ultralarge mesoporous silica nanostructures and anti-Aβ single-chain variable fragments (anti-Aβ scFvs). The Aβ nanodepletors suppressed Aβ self-assembly, decreased the amount of Aβ aggregates, and increased cell viability.
In addition, many other nanomaterials have been reported for AD diagnosis and treatment, such as carbon nanospheres, hydrogen-bonded organic frameworks (HOFs), polystyrene nanoparticles and so on. Ma et al.272 designed NIR-II photothermally responsive mesoporous carbon nanospheres KD8@N-MCNs. The graphitic N dopants introduced abundant electrons into the p* orbital between the HOMO and LUMO gaps, thus enhancing the light absorption properties. Under 1064 nm light irradiation, the nanospheres disaggregated Aβ42 aggregates because of photothermal conversion ability. Meanwhile, KD8@N-MCNs alleviated oxidative stress due to the SOD and CAT enzymatic activities. Due to the covalently grafted KLVFFAED, KD8@N-MCNs could cross the BBB and specifically recognize Aβ42 aggregates. HOF materials exhibit considerable biocompatibility and low toxicity attributed to their metal-free nature, thus being an excellent candidate for drug delivery and biological applications.273 Zhang et al. designed a two-photon NIR-II-activated photooxygenation catalyst DSM@n-HOF-6 (DSM = 4-[p-(dimethylamino) styryl]-1-methylpyridinium). TCPP(meso-tetrakis(carboxy phenyl)porphyrin) was periodically incorporated into HOFs, while the targeting peptide KLVFFAED (KD8) was conjugated to DSM@n-HOF-6 (DSM@n-HOF-6@KD8).274 The up-conversion fluorescence of DSM could be absorbed by TCPP to generate 1O2 for Aβ oxygenation, and DSM@n-HOF-6@KD8 could inhibit the fibrillation of Aβ monomers and reduce the cytotoxicity of Aβ by photooxygenation. The application of polystyrene nanoparticles and upconverting nanoparticles in amyloid diseases has also been reported.275,276
Many nanomaterials have been reported for AD diagnosis and treatment. Targeting issues and the interaction between nanomaterials and peptides are the main issues that need to be considered. At the same time, issues such as inhibition efficiency, reversibility of fibrosis, and nanoparticle metabolism also need to be considered.
6 Conclusions and outlook
Due to the intensification of the aging of the population, neurodegenerative diseases, especially AD, have become one of the most serious obstacles to social development. This review comprehensively summarized the recent research for modulating amyloid aggregation associated with neurodegenerative diseases, including AD based on nanomaterials and nanotechnology. In this review, nanomaterials exhibited multiple roles in the treatment of AD. Firstly, nanomaterials can directly interact with Aβ peptides and accelerate or slow down amyloid aggregation. Secondly, as nanocarriers, nanomaterials can also be used to load various drugs and assist drugs to across the BBB and inhibit amyloid. In addition, nanomaterials and drugs can synergistically resist a series of problems arising from amyloid aggregation. Moreover, some advanced nanomaterials with photosensitivity can strongly affect amyloid aggregation through PTT or PDT.277 Multiple interaction mechanisms such as electrostatic interaction, hydrophobic interaction, π–π stacking, and metal ion chelation are the main reasons for amyloid fibrillation, and taking advantage of these interactions in the design process and application process, nanomaterials can effectively adsorb amyloids on their surface and block the amyloid aggregation. As novel treatment methods, advanced nanomaterials with the function of PTT and PDT have the advantages of accuracy, ease of administration, high efficiency, and few side effects. In PDT treatment, nanomaterials can generate ROS and oxidize the amino acid residue of amyloid, and then the aggregation process of amyloid is inhibited. In PTT treatment, because the amyloid formation is highly dependent on temperature, the change of localized temperature can affect amyloid aggregation. Currently, PDT and PTT have attracted more and more attention from researchers, and these methods may be the focus of follow-up research. In the future, a deep and comprehensive understanding of the functional design of nanomaterials and the properties of these nanomaterials is still necessary. The mechanism of action of nanomaterials in AD treatment, especially the in-depth research mechanism of amyloid, including interaction and photo-inhibition process, also needs to be further explored. From the current point of view, the development of nanomaterials has shown a new chapter in the treatment of AD.
Regarding the application of nanomaterials for inhibiting amyloid, there are several aspects to note: (1) in order to successfully achieve clinical applications, it is necessary to further understand and explore the in vivo distribution and metabolism of nanomaterials. In future research, it is necessary to continue in-depth research on the biological properties, preparation processes, and surface modification of nanomaterials to achieve a more safe and more efficient inhibition of amyloid aggregation and disaggregation of amyloid fibrils. (2) The BBB permeability of nanomaterials can be improved through some conjugates such as transferrin (via transferrin receptor-mediated endocytosis to cross the BBB), and transiently disrupting the blood–brain barrier by physical methods such as photothermal and intranasal administration may also be an effective method. (3) To predict the interactions between amyloid/fibrils and nanoparticles in advance, it is also necessary to build suitable computational models and deeply explore how amyloid/fibrils and nanoparticles interact. (4) Most nanomaterials interact with amyloid through non-covalent interactions, which are weak and may cause reversible aggregation/disaggregation processes, and covalent modulators can prolong their duration of action. PDT and PTT also can directly irreversibly modulate the amyloid fibrosis process. (5) The role of nanomaterials and amyloid at the cellular level and in vivo is worth further research in the future, which will provide a strong basis for a biological experiment for nanomaterials to transform nanomedicines. (6) For precise inhibition, the problem of targeting needs to be solved urgently. Targeting ligands have been introduced, such as antibodies, peptides, and aptamers. The amyloid targeting ligand may lose or weaken its binding affinity to amyloid during PDT or PTT, so it is crucial to develop targeting ligands with high stability and strong affinity under harsh conditions. (7) Many studies have pointed to oligomers, and the subsequent application of nanomaterials in oligomers will become the focus and be further explored. (8) Although studies have shown that nanomaterials have a good inhibition efficiency in vitro, the inhibition efficiency needs to be further improved in vivo. To prove whether the addition of nanomaterials can restore the normal function of nerve cells, various experiments in vivo including clinical research still need to be carried out.
Conflicts of interest
The authors declare no conflict of interest.
Acknowledgements
Xu Shao and Chaoren Yan contributed equally to this work. The authors in NPU acknowledge the financial support from the National Natural Science Foundation of China (32171388 and 81702246), Innovation Capability Support Plan of Shaanxi Province (2020TD-041), Shaanxi Key Research & Development Program Foundation (2020GY-285), Shaanxi Natural Science Foundation (2021JM-238), Shaanxi Key Research & Development Program Foundation (2019SF-069), and Innovation Foundation for Doctor Dissertation of Northwestern Polytechnical University (CX2021112), and partial funding by the Key Research and Development Program of Shaanxi (Program No. 2022GY-198).
References
- C. Q. Liang and Y. M. Li, Peptides for disrupting and degrading amyloids, Curr. Opin. Chem. Biol., 2021, 64, 124–130 CrossRef CAS PubMed.
- S. i. Ikeda, Amyloid neuropathy and autonomic dysfunction, Neurol. Clin. Neurosci., 2022, 10(3), 137–146 CrossRef.
-
Alzheimer's Disease International and McGill University, World Alzheimer Report 2021 Search PubMed.
- Y.-C. Pan, H. Wang, X. Xu, H.-W. Tian, H. Zhao, X.-Y. Hu, Y. Zhao, Y. Liu, G. Ding, Q. Meng, B. J. Ravoo, T. Zhang and D.-S. Guo, Coassembly of Macrocyclic Amphiphiles for Anti-β-Amyloid Therapy of Alzheimer's Disease, CCS Chem., 2021, 3(9), 2485–2497 CrossRef.
- W. Liu, X. Dong, Y. Liu and Y. Sun, Photoresponsive materials for intensified modulation of Alzheimer's amyloid-β protein aggregation: a review, Acta Biomater., 2021, 123, 93–109 CrossRef PubMed.
- Z. Lyu, S. Ding, N. Zhang, Y. Zhou, N. Cheng, M. Wang, M. Xu, Z. Feng, X. Niu, Y. Cheng, C. Zhang, D. Du and Y. Lin, Single-Atom Nanozymes Linked Immunosorbent Assay for Sensitive Detection of Abeta 1-40: A Biomarker of Alzheimer's Disease, Research, 2020, 2020, 4724505 CrossRef PubMed.
- K. Ono and T. Watanabe-Nakayama, Aggregation and structure of amyloid beta-protein, Neurochem. Int., 2021, 151, 105208 CrossRef PubMed.
- H. Liu, C. Qian, T. Yang, Y. Wang, J. Luo, C. Zhang, X. Wang, X. Wang and Z. Guo, Small molecule-mediated co-assembly of amyloid-beta oligomers reduces neurotoxicity through promoting non-fibrillar aggregation, Chem. Sci., 2020, 11(27), 7158–7169 RSC.
- V. Oliveri and G. Vecchio, Bis(8-hydroxyquinoline) Ligands: Exploring their Potential as Selective Copper-Binding Agents for Alzheimer's Disease, Eur. J. Inorg. Chem., 2021, 2021(21), 1993–1999 CrossRef.
- H. Geng, Y. c. Pan, R. Zhang, D. Gao, Z. Wang, B. Li, N. Li, D. s. Guo and C. Xing, Binding to Amyloid-β Protein by Photothermal Blood–Brain Barrier-Penetrating Nanoparticles for Inhibition and Disaggregation of Fibrillation, Adv. Funct. Mater., 2021, 31(41), 2102953 CrossRef CAS.
- H. Geng, D. Gao, Z. Wang, X. Liu, Z. Cao and C. Xing, Strategies for Inhibition and Disaggregation of Amyloid-β Fibrillation, Chin. J. Chem., 2021, 40(4), 524–538 CrossRef.
- K. J. Y. Low, A. Venkatraman, J. S. Mehta and K. Pervushin, Molecular mechanisms of amyloid disaggregation, J. Adv. Res., 2022, 36, 113–132 CrossRef CAS PubMed.
- C. Yan, C. Wang, X. Shao, Q. Shu, X. Hu, P. Guan, Y. Teng and Y. Cheng, Dual-targeted carbon-dot-drugs nanoassemblies for modulating Alzheimer's related amyloid-β aggregation and inhibiting fungal infection, Mater. Today Bio, 2021, 12, 100167 CrossRef CAS PubMed.
- A. Li, J. Tyson, S. Patel, M. Patel, S. Katakam, X. Mao and W. He, Emerging Nanotechnology for Treatment of Alzheimer's and Parkinson's Disease, Front. Bioeng. Biotechnol., 2021, 9, 672594 CrossRef PubMed.
- Y. Zhou, P. Y. Liyanage, D. Devadoss, L. R. Rios Guevara, L. Cheng, R. M. Graham, H. S. Chand, A. O. Al-Youbi, A. S. Bashammakh, M. S. El-Shahawi and R. M. Leblanc, Nontoxic amphiphilic carbon dots as promising drug nanocarriers across the blood–brain barrier and inhibitors of beta-amyloid, Nanoscale, 2019, 11(46), 22387–22397 RSC.
- S. Ding, A. I. Khan, X. Cai, Y. Song, Z. Lyu, D. Du, P. Dutta and Y. Lin, Overcoming blood–brain barrier transport: advances in nanoparticle-based drug delivery strategies, Mater. Today, 2020, 37, 112–125 CrossRef PubMed.
- P. C. Ke, E. H. Pilkington, Y. Sun, I. Javed, A. Kakinen, G. Peng, F. Ding and T. P. Davis, Mitigation of Amyloidosis with Nanomaterials, Adv. Mater., 2020, 32(18), e1901690 CrossRef PubMed.
- L. Gong, X. Zhang, K. Ge, Y. Yin, J. O. Machuki, Y. Yang, H. Shi, D. Geng and F. Gao, Carbon nitride-based nanocaptor: an intelligent nanosystem with metal ions chelating effect for enhanced magnetic targeting phototherapy of Alzheimer's disease, Biomaterials, 2021, 267, 120483 CrossRef PubMed.
- C. Yan, N. Zhang, P. Guan, P. Chen, S. Ding, T. Hou, X. Hu, J. Wang and C. Wang, Drug-based magnetic imprinted nanoparticles: enhanced lysozyme amyloid fibrils cleansing and anti-amyloid fibrils toxicity, Int. J. Biol. Macromol., 2020, 153, 723–735 CrossRef PubMed.
- S. Ding, N. Zhang, Z. Lyu, W. Zhu, Y.-C. Chang, X. Hu, D. Du and Y. Lin, Protein-based nanomaterials and nanosystems for biomedical applications: a review, Mater. Today, 2021, 43, 166–184 CrossRef CAS.
- Z. Du, M. Li, J. Ren and X. Qu, Current Strategies for Modulating Abeta Aggregation with Multifunctional Agents, Acc. Chem. Res., 2021, 54(9), 2172–2184 CrossRef CAS PubMed.
- F. Yuan, Y. Xia, Q. Lu, Q. Xu, Y. Shu and X. Hu, Recent advances in inorganic functional nanomaterials based flexible electrochemical sensors, Talanta, 2022, 244, 123419 CrossRef CAS.
- S. Ding, Z. Lyu, L. Fang, T. Li, W. Zhu, S. Li, X. Li, J. C. Li, D. Du and Y. Lin, Single-Atomic Site Catalyst with Heme Enzymes-Like Active Sites for Electrochemical Sensing of Hydrogen Peroxide, Small, 2021, 17(25), e2100664 CrossRef PubMed.
- S. Liu, X. Pan and H. Liu, Two-Dimensional Nanomaterials for Photothermal Therapy, Angew. Chem., Int. Ed., 2020, 59(15), 5890–5900 CrossRef PubMed.
- X. Wu, X. Jiang, T. Fan, Z. Zheng, Z. Liu, Y. Chen, L. Cao, Z. Xie, D. Zhang, J. Zhao, Q. Wang, Z. Huang, Z. Chen, P. Xue and H. Zhang, Recent advances in photodynamic therapy based on emerging two-dimensional layered nanomaterials, Nano Res., 2020, 13(6), 1485–1508 CrossRef.
- S. Wang, L. Zhou, Y. Zheng, L. Li, C. Wu, H. Yang, M. Huang and X. An, Synthesis and biocompatibility of two-dimensional biomaterials, Colloids Surf., A, 2019, 583, 124004 CrossRef.
- I. S. Raja, S. J. Song, M. S. Kang, Y. B. Lee, B. Kim, S. W. Hong, S. J. Jeong, J. C. Lee and D. W. Han, Toxicity of Zero- and One-Dimensional Carbon Nanomaterials, Nanomaterials, 2019, 9(9), 1214 CrossRef PubMed.
- S. Banerjee, C. T. Lollar, Z. Xiao, Y. Fang and H.-C. Zhou, Biomedical Integration of Metal–Organic Frameworks, Trends Chem., 2020, 2(5), 467–479 CrossRef.
- J. Zhao, K. Li, K. Wan, T. Sun, N. Zheng, F. Zhu, J. Ma, J. Jiao, T. Li, J. Ni, X. Shi, H. Wang, Q. Peng, J. Ai, W. Xu and S. Liu, Organoplatinum-Substituted Polyoxometalate Inhibits beta-amyloid Aggregation for Alzheimer's Therapy, Angew. Chem., Int. Ed., 2019, 58(50), 18032–18039 CrossRef PubMed.
- H. Jung, Y. J. Chung, R. Wilton, C. H. Lee, B. I. Lee, J. Lim, H. Lee, J. H. Choi, H. Kang, B. Lee, E. A. Rozhkova, C. B. Park and J. Lee, Silica Nanodepletors: Targeting and Clearing Alzheimer's β-Amyloid Plaques, Adv. Funct. Mater., 2020, 30(15), 1910475 CrossRef CAS.
- M. Samykano, Progress in one-dimensional nanostructures, Mater. Charact., 2021, 179, 111373 CrossRef CAS.
- Z. Tang, J. Zhang and Y. Zhang, Renaissance of One-Dimensional Nanomaterials, Adv. Funct. Mater., 2022, 32(11), 2113192 CrossRef CAS.
- H. Lin, L. Yang, X. Zhang, G. Liu, S. Zhuo, J. Chen and J. Song, Emerging Low-Dimensional Nanoagents for Bio-Microimaging, Adv. Funct. Mater., 2020, 30(40), 2003147 CrossRef CAS.
- S. Wang, J. Zheng, L. Ma, R. B. Petersen, L. Xu and K. Huang, Inhibiting protein aggregation with nanomaterials: the underlying mechanisms and impact factors, Biochim. Biophys. Acta, Gen. Subj., 2022, 1866(2), 130061 CrossRef PubMed.
- M. Kolahdouz, B. Xu, A. F. Nasiri, M. Fathollahzadeh, M. Manian, H. Aghababa, Y. Wu and H. H. Radamson, Carbon-Related Materials: Graphene and Carbon Nanotubes in Semiconductor Applications and Design, Micromachines, 2022, 13(8), 1257 CrossRef PubMed.
- A. Rode, S. Sharma and K. D. Mishra, Carbon Nanotubes: Classification, Method of Preparation and Pharmaceutical Application, Curr. Drug Delivery, 2018, 15(5), 620–629 CrossRef PubMed.
- P. S. Shiv Charan, S. Shanmugam and V. Kamaraj, Carbon Nanotubes—Synthesis and Application, Trans. Indian Ceram. Soc., 2015, 68(4), 163–172 CrossRef.
- B. Li, Formation of helicity in an armchair single-walled carbon nanotube during tensile loading, Comput. Mater. Sci., 2013, 74, 27–32 CrossRef.
- K. Huth, M. Glaeske, K. Achazi, G. Gordeev, S. Kumar, R. Arenal, S. K. Sharma, M. Adeli, A. Setaro, S. Reich and R. Haag, Fluorescent Polymer-Single-Walled Carbon Nanotube Complexes with Charged and Noncharged Dendronized Perylene Bisimides for Bioimaging Studies, Small, 2018, 14(28), e1800796 CrossRef PubMed.
- S. Erbas, A. Gorgulu, M. Kocakusakogullari and E. U. Akkaya, Non-covalent functionalized SWNTs as delivery agents for novel Bodipy-based potential PDT sensitizers, Chem. Commun., 2009, 33, 4956–4958 RSC.
- Z. Li, A. L. B. de Barros, D. C. F. Soares, S. N. Moss and L. Alisaraie, Functionalized single-walled carbon nanotubes: cellular uptake, biodistribution and applications in drug delivery, Int. J. Pharm., 2017, 524(1–2), 41–54 CrossRef PubMed.
- B. Oruc and H. Unal, Fluorophore-Decorated Carbon Nanotubes with Enhanced Photothermal Activity as Antimicrobial Nanomaterials, ACS Omega, 2019, 4(3), 5556–5564 CrossRef.
- S. Kam Nadine Wong, M. O'Connell, A. Wisdom Jeffrey and H. Dai, Carbon nanotubes as multifunctional biological transporters and near-infrared agents for selective cancer cell destruction, Proc. Natl. Acad. Sci. U. S. A., 2005, 102(33), 11600–11605 CrossRef.
- G. Hong, S. Diao, A. L. Antaris and H. Dai, Carbon Nanomaterials for Biological Imaging and Nanomedicinal Therapy, Chem. Rev., 2015, 115(19), 10816–10906 CrossRef.
- J. Luo, S. K. Warmlander, C. H. Yu, K. Muhammad, A. Graslund and J. Pieter Abrahams, The Abeta peptide forms non-amyloid fibrils in the presence of carbon nanotubes, Nanoscale, 2014, 6(12), 6720–6726 RSC.
- H. Li, Y. Luo, P. Derreumaux and G. Wei, Carbon nanotube inhibits the formation of beta-sheet-rich oligomers of the Alzheimer's amyloid-beta(16-22) peptide, Biophys. J., 2011, 101(9), 2267–2276 CrossRef PubMed.
- L. Xie, D. Lin, Y. Luo, H. Li, X. Yang and G. Wei, Effects of hydroxylated carbon nanotubes on the aggregation of Abeta16-22 peptides: a combined simulation and experimental study, Biophys. J., 2014, 107(8), 1930–1938 CrossRef PubMed.
- F. Liu, W. Wang, J. Sang, L. Jia and F. Lu, Hydroxylated Single-Walled Carbon Nanotubes Inhibit Abeta42 Fibrillogenesis, Disaggregate Mature Fibrils, and Protect against Abeta42-Induced Cytotoxicity, ACS Chem. Neurosci., 2019, 10(1), 588–598 CrossRef PubMed.
- N. Zhang, J. Yeo, Y. Lim, P. Guan, K. Zeng, X. Hu and Y. Cheng, Tuning the structure of monomeric amyloid beta peptide by the curvature of carbon nanotubes, Carbon, 2019, 153, 717–724 CrossRef.
- D. Lin, J. Lei, S. Li, X. Zhou, G. Wei and X. Yang, Investigation of the Dissociation Mechanism of Single-Walled Carbon Nanotube on Mature Amyloid-beta Fibrils at Single Nanotube Level, J. Phys. Chem. B, 2020, 124(17), 3459–3468 CrossRef PubMed.
- J. H. Lucas, Q. Wang, T. Muthumalage and I. Rahman, Multi-Walled Carbon Nanotubes (MWCNTs) Cause Cellular Senescence in TGF-beta Stimulated Lung Epithelial Cells, Toxics, 2021, 9(6), 144 CrossRef PubMed.
- S. Lohan, K. Raza, S. K. Mehta, G. K. Bhatti, S. Saini and B. Singh, Anti-Alzheimer's potential of berberine using surface decorated multi-walled carbon nanotubes: a preclinical evidence, Int. J. Pharm., 2017, 530(1–2), 263–278 CrossRef PubMed.
- Q. Zong, N. Dong, X. Yang, G. Ling and P. Zhang, Development of gold nanorods for cancer treatment, J. Inorg. Biochem., 2021, 220, 111458 CrossRef PubMed.
- T. Patil, R. Gambhir, A. Vibhute and A. P. Tiwari, Gold Nanoparticles: Synthesis Methods, Functionalization and Biological Applications, J. Cluster Sci., 2022, 1–21 Search PubMed.
- M. Li, H. Gong, R. Zhou, T. Zhou and Q. Tao, Synthesis of gold nanorods with longitudinal surface plasmon resonance wavelength up to 1245 nm using gallic acid as a reductant in the presence of a binary surfactant system, Micro Nano Lett., 2015, 10(9), 456–459 CrossRef.
- D. Shajari, A. Bahari, P. Gill and M. Mohseni, Synthesis and tuning of gold nanorods with surface plasmon resonance, Opt. Mater., 2017, 64, 376–383 CrossRef.
- L. Tong, Q. Wei, A. Wei and J.-X. Cheng, Gold Nanorods as Contrast Agents for Biological Imaging: Optical Properties, Surface Conjugation and Photothermal Effects, Photochem. Photobiol., 2009, 85(1), 21–32 CrossRef PubMed.
- Q. Zong, N. Dong, X. Yang, G. Ling and P. Zhang, Development of gold nanorods for cancer treatment, J. Inorg. Biochem., 2021, 220, 111458 CrossRef PubMed.
- J. Wang, H. Z. Zhang, R. S. Li and C. Z. Huang, Localized surface plasmon resonance of gold nanorods and assemblies in the view of biomedical analysis, TrAC, Trends Anal. Chem., 2016, 80, 429–443 CrossRef.
- S. Liao, W. Yue, S. Cai, Q. Tang, W. Lu, L. Huang, T. Qi and J. Liao, Improvement of Gold Nanorods in Photothermal Therapy: Recent Progress and Perspective, Front. Pharmacol., 2021, 12, 664123 CrossRef PubMed.
- C. Fernandez, G. Gonzalez-Rubio, J. Langer, G. Tardajos, L. M. Liz-Marzan, R. Giraldo and A. Guerrero-Martinez, Nucleation of Amyloid Oligomers by RepA-WH1-Prionoid-Functionalized Gold Nanorods, Angew. Chem., Int. Ed., 2016, 55(37), 11237–11241 CrossRef PubMed.
- D. Lin, R. He, S. Li, Y. Xu, J. Wang, G. Wei, M. Ji and X. Yang, Highly Efficient Destruction of Amyloid-beta Fibrils by Femtosecond Laser-Induced Nanoexplosion of Gold Nanorods, ACS Chem. Neurosci., 2016, 7(12), 1728–1736 CrossRef PubMed.
- S. Sudhakar, P. B. Santhosh and E. Mani, Dual Role of Gold Nanorods: Inhibition and Dissolution of Abeta Fibrils Induced by Near IR Laser, ACS Chem. Neurosci., 2017, 8(10), 2325–2334 CrossRef PubMed.
- Y. Liu, G. He, Z. Zhang, H. Yin, H. Liu, J. Chen, S. Zhang, B. Yang, L.-P. Xu and X. Zhang, Size-effect of gold nanorods on modulating the kinetic process of amyloid-β aggregation, Chem. Phys. Lett., 2019, 734, 136702 CrossRef CAS.
- D. Liu, W. Li, X. Jiang, S. Bai, J. Liu, X. Liu, Y. Shi, Z. Kuai, W. Kong, R. Gao and Y. Shan, Using near-infrared enhanced thermozyme and scFv dual-conjugated Au nanorods for detection and targeted photothermal treatment of Alzheimer's disease, Theranostics, 2019, 9(8), 2268–2281 CrossRef CAS PubMed.
- F. Morales-Zavala, H. Arriagada, N. Hassan, C. Velasco, A. Riveros, A. R. Alvarez, A. N. Minniti, X. Rojas-Silva, L. L. Munoz, R. Vasquez, K. Rodriguez, M. Sanchez-Navarro, E. Giralt, E. Araya, R. Aldunate and M. J. Kogan, Peptide multifunctionalized gold nanorods decrease toxicity of beta-amyloid peptide in a Caenorhabditis elegans model of Alzheimer's disease, Nanomedicine, 2017, 13(7), 2341–2350 CrossRef CAS PubMed.
- F. Morales-Zavala, P. Jara-Guajardo, D. Chamorro, A. L. Riveros, A. Chandia-Cristi, N. Salgado, P. Pismante, E. Giralt, M. Sanchez-Navarro, E. Araya, R. Vasquez, G. Acosta, F. Albericio, R. A. Alvarez and M. J. Kogan,
In vivo micro computed tomography detection and decrease in amyloid load by using multifunctionalized gold nanorods: a neurotheranostic platform for Alzheimer's disease, Biomater. Sci., 2021, 9(11), 4178–4190 RSC.
- J. Zhao, S. Huang, P. Ravisankar and H. Zhu, Two-Dimensional Nanomaterials for Photoinduced Antibacterial Applications, ACS Appl. Bio Mater., 2020, 3(12), 8188–8210 CrossRef CAS PubMed.
- S. Liu, X. Pan and H. Liu, Two-Dimensional Nanomaterials for Photothermal Therapy, Angew. Chem., Int. Ed., 2020, 59(15), 5890–5900 CrossRef PubMed.
- Z. Li, X. Zhu, J. Li, J. Zhong, J. Zhang and J. Fan, Molecular insights into the resistance of phospholipid heads to the membrane penetration of graphene nanosheets, Nanoscale, 2022, 14(14), 5384–5391 RSC.
- X. Mei, T. Hu, Y. Wang, X. Weng, R. Liang and M. Wei, Recent advancements in two-dimensional nanomaterials for drug delivery, Wiley Interdiscip. Rev.: Nanomed. Nanobiotechnol., 2020, 12(2), e1596 Search PubMed.
- A. Halim, K.-Y. Qu, X.-F. Zhang and N.-P. Huang, Recent Advances in the Application of Two-Dimensional Nanomaterials for Neural Tissue Engineering and Regeneration, ACS Biomater. Sci. Eng., 2021, 7(8), 3503–3529 CrossRef PubMed.
- A. Murali, G. Lokhande, K. A. Deo, A. Brokesh and A. K. Gaharwar, Emerging 2D Nanomaterials for Biomedical Applications, Mater. Today, 2021, 50, 276–302 CrossRef PubMed.
- J. Chen, T. Fan, Z. Xie, Q. Zeng, P. Xue, T. Zheng, Y. Chen, X. Luo and H. Zhang, Advances in nanomaterials for photodynamic therapy applications: status and challenges, Biomaterials, 2020, 237, 119827 CrossRef CAS PubMed.
- Z. Lyu, S. Ding, D. Du, K. Qiu, J. Liu, K. Hayashi, X. Zhang and Y. Lin, Recent advances in biomedical applications of 2D nanomaterials with peroxidase-like properties, Adv. Drug Delivery Rev., 2022, 185, 114269 CrossRef PubMed.
- S. Song, J. Wu, Y. Cheng, L. Ma, T. Liu, J. Liu, J. Liu, J. Sotor and P. Luan, Emerging two-dimensional materials-enabled diagnosis and treatments of Alzheimer's disease: status and future challenges, Appl. Mater. Today, 2021, 23, 101028 CrossRef.
- S. Priyadarsini, S. Mohanty, S. Mukherjee, S. Basu and M. Mishra, Graphene and graphene oxide as nanomaterials for medicine and biology application, J. Nanostruct. Chem., 2018, 8(2), 123–137 CrossRef.
- T. V. Patil, D. K. Patel, S. D. Dutta, K. Ganguly and K.-T. Lim, Graphene Oxide-Based Stimuli-Responsive Platforms for Biomedical Applications, Molecules, 2021, 26(9), 2797 CrossRef PubMed.
- S. Han, J. Sun, S. He, M. Tang and R. Chai, The application of graphene-based biomaterials in biomedicine, Am. J. Transl. Res., 2019, 11(6), 3246–3260 Search PubMed.
- G. Yildiz, M. Bolton-Warberg and F. Awaja, Graphene and graphene oxide for bio-sensing: general properties and the effects of graphene ripples, Acta Biomater., 2021, 131, 62–79 CrossRef CAS PubMed.
- M. Mahmoudi, O. Akhavan, M. Ghavami, F. Rezaee and S. M. Ghiasi, Graphene oxide strongly inhibits amyloid beta fibrillation, Nanoscale, 2012, 4(23), 7322–7325 RSC.
- Q. Li, L. Liu, S. Zhang, M. Xu, X. Wang, C. Wang, F. Besenbacher and M. Dong, Modulating Aβ33–42 Peptide Assembly by Graphene Oxide, Chem.–Eur. J., 2014, 20(24), 7236–7240 CrossRef CAS PubMed.
- J. Wang, Y. Cao, Q. Li, L. Liu and M. Dong, Size Effect of Graphene Oxide on Modulating Amyloid Peptide Assembly, Chemistry, 2015, 21(27), 9632–9637 CrossRef CAS PubMed.
- I. Ahmad, A. Mozhi, L. Yang, Q. Han, X. Liang, C. Li, R. Yang and C. Wang, Graphene oxide-iron oxide nanocomposite as an inhibitor of Abeta42 amyloid peptide aggregation, Colloids Surf., B, 2017, 159, 540–545 CrossRef CAS PubMed.
- Z. Yang, C. Ge, J. Liu, Y. Chong, Z. Gu, C. A. Jimenez-Cruz, Z. Chai and R. Zhou, Destruction of amyloid fibrils by graphene through penetration and extraction of peptides, Nanoscale, 2015, 7(44), 18725–18737 RSC.
- Y. Chen, Z. Chen, Y. Sun, J. Lei and G. Wei, Mechanistic insights into the inhibition and size effects of graphene oxide nanosheets on the aggregation of an amyloid-β peptide fragment, Nanoscale, 2018, 10(19), 8989–8997 RSC.
- Y. Jin, Y. Sun, Y. Chen, J. Lei and G. Wei, Molecular dynamics simulations reveal the mechanism of graphene oxide nanosheet inhibition of Abeta1–42 peptide aggregation, Phys. Chem. Chem. Phys., 2019, 21(21), 10981–10991 RSC.
- X. Yin, B. Li, S. Liu, Z. Gu, B. Zhou and Z. Yang, Effect of the surface curvature on amyloid-beta peptide adsorption for graphene, RSC Adv., 2019, 9(18), 10094–10099 RSC.
- H. He, J. Xu, C. Q. Li, T. Gao, P. Jiang, F. L. Jiang and Y. Liu, Insights into Mechanism of Abeta42 Fibril Growth on Surface of Graphene Oxides: Oxidative Degree Matters, Adv. Healthcare Mater., 2021, 10(16), e2100436 CrossRef PubMed.
- X. Li, K. Li, F. Chu, J. Huang and Z. Yang, Graphene oxide enhances beta-amyloid clearance by inducing autophagy of microglia and neurons, Chem.-Biol. Interact., 2020, 325, 109126 CrossRef CAS PubMed.
- M. Li, X. Yang, J. Ren, K. Qu and X. Qu, Using graphene oxide high near-infrared absorbance for photothermal treatment of Alzheimer's disease, Adv. Mater., 2012, 24(13), 1722–1728 CrossRef CAS PubMed.
- J. Xia, Y. Zhu, Z. He, F. Wang and H. Wu, Superstrong Noncovalent Interface between Melamine and Graphene Oxide, ACS Appl. Mater. Interfaces, 2019, 11(18), 17068–17078 CrossRef CAS PubMed.
- E. V. S. Maciel, K. Mejia-Carmona, M. Jordan-Sinisterra, L. F. da Silva, D. A. Vargas Medina and F. M. Lancas, The Current Role of Graphene-Based Nanomaterials in the Sample Preparation Arena, Front. Chem., 2020, 8, 664 CrossRef CAS PubMed.
- K. Wang, L. Wang, L. Chen, C. Peng, B. Luo, J. Mo and W. Chen, Intranasal administration of dauricine loaded on graphene oxide: multi-target therapy for Alzheimer's disease, Drug Delivery, 2021, 28(1), 580–593 CrossRef CAS PubMed.
- T. A. Tabish and R. J. Narayan, Crossing the blood–brain barrier with graphene nanostructures, Mater. Today, 2021, 51, 393–401 CrossRef CAS.
- S. Su, J. Wang, J. Qiu, R. Martinez-Zaguilan, S. R. Sennoune and S. Wang,
In vitro study of transportation of porphyrin immobilized graphene oxide through blood–brain barrier, Mater. Sci. Eng., C, 2020, 107, 110313 CrossRef CAS.
- T. M. Magne, T. de Oliveira Vieira, L. M. R. Alencar, F. F. M. Junior, S. Gemini-Piperni, S. V. Carneiro, L. Fechine, R. M. Freire, K. Golokhvast, P. Metrangolo, P. B. A. Fechine and R. Santos-Oliveira, Graphene and its derivatives: understanding the main chemical and medicinal chemistry roles for biomedical applications, J. Nanostruct. Chem., 2022, 12(5), 693–727 CrossRef CAS.
- S. Syama, W. Paul, A. Sabareeswaran and P. V. Mohanan, Raman spectroscopy for the detection of organ distribution and clearance of PEGylated reduced graphene oxide and biological consequences, Biomaterials, 2017, 131, 121–130 CrossRef CAS.
- S. K. Gaddam, R. Pothu and R. Boddula, Graphitic carbon nitride (g-C3N4) reinforced polymer nanocomposite systems—a review, Polym. Compos., 2020, 41(2), 430–442 CrossRef CAS.
- J. Wen, J. Xie, X. Chen and X. Li, A review on g-C3N4-based photocatalysts, Appl. Surf. Sci., 2017, 391, 72–123 CrossRef CAS.
- Q. Huang, L. Hao, R. Zhou, B. Zhu, H. Zhao and X. Cai, Synthesis, Characterization, and Biological Study of Carboxyl- and Amino-Rich g-C3N4 Nanosheets by Different Processing Routes, J. Biomed. Nanotechnol., 2018, 14(12), 2114–2123 CrossRef CAS PubMed.
- Y. Cao, R. Wu, Y. Zhou, D. Jiang and W. Zhu, A Bioinspired Photocatalysis and Electrochemiluminescence Scaffold for Simultaneous Degradation and In Situ Evaluation, Adv. Funct. Mater., 2022, 32(31), 2203005 CrossRef CAS.
- G. Liao, F. He, Q. Li, L. Zhong, R. Zhao, H. Che, H. Gao and B. Fang, Emerging graphitic carbon nitride-based materials for biomedical applications, Prog. Mater. Sci., 2020, 112, 100666 CrossRef CAS.
- M. Li, Y. Guan, C. Ding, Z. Chen, J. Ren and X. Qu, An ultrathin graphitic carbon nitride nanosheet: a novel inhibitor of metal-induced amyloid aggregation associated with Alzheimer's disease, J. Mater. Chem. B, 2016, 4(23), 4072–4075 RSC.
- M. Li, Y. Guan, Z. Chen, N. Gao, J. Ren, K. Dong and X. Qu, Platinum-coordinated graphitic carbon nitride nanosheet used for targeted inhibition of amyloid β-peptide aggregation, Nano Res., 2016, 9(8), 2411–2423 CrossRef CAS.
- J. Wang, Z. Zhang, H. Zhang, C. Li, M. Chen, L. Liu and M. Dong, Enhanced Photoresponsive Graphene Oxide-Modified g-C3N4 for Disassembly of Amyloid beta Fibrils, ACS Appl. Mater. Interfaces, 2019, 11(1), 96–103 CrossRef CAS.
- J. Wang, Y. Feng, X. Tian, C. Li and L. Liu, Disassembling and degradation of amyloid protein aggregates based on gold nanoparticle-modified g-C3N4, Colloids Surf., B, 2020, 192, 111051 CrossRef CAS PubMed.
- Y. J. Chung, B. I. Lee, J. W. Ko and C. B. Park, Photoactive g-C3N4 Nanosheets for Light-Induced Suppression of Alzheimer's beta-Amyloid Aggregation and Toxicity, Adv. Healthcare Mater., 2016, 5(13), 1560–1565 CrossRef CAS PubMed.
- A. Durairaj, T. Sakthivel, A. Obadiah and S. Vasanthkumar, Enhanced photocatalytic activity of transition metal ions doped g-C3N4 nanosheet activated by PMS for organic pollutant degradation, J. Mater. Sci.: Mater. Electron., 2018, 29(10), 8201–8209 CrossRef CAS.
- F. Wei, S. Kuang, T. W. Rees, X. Liao, J. Liu, D. Luo, J. Wang, X. Zhang, L. Ji and H. Chao, Ruthenium(II) complexes coordinated to graphitic carbon nitride: oxygen self-sufficient photosensitizers which produce multiple ROS for photodynamic therapy in hypoxia, Biomaterials, 2021, 276, 121064 CrossRef PubMed.
- T. Li, J. Zhang, K. Zheng and C. Xu, A supramolecule-based shape-controllable preparation of carbon nitride nanotubes for the visible light driven photodegradation, Surf. Interfaces, 2022, 30, 101894 CrossRef.
- M. Talukdar and P. Deb, Recent progress in research on multifunctional graphitic carbon nitride: an emerging wonder material beyond catalyst, Carbon, 2022, 192, 308–331 CrossRef.
- Y. Qing, R. Li, S. Li, Y. Li, X. Wang and Y. Qin, Advanced Black Phosphorus Nanomaterials for Bone Regeneration, Int. J. Nanomed., 2020, 15, 2045–2058 CrossRef.
- Y. Xu, W. Zhang, G. Zhou, M. Jin and X. Li,
In situ growth of metal phosphide-black phosphorus heterojunction for highly selective and efficient photocatalytic carbon dioxide conversion, J. Colloid Interface Sci., 2022, 616, 641–648 CrossRef.
- L. Cheng, Z. Cai, J. Zhao, F. Wang, M. Lu, L. Deng and W. Cui, Black phosphorus-based 2D materials for bone therapy, Bioact. Mater., 2020, 5(4), 1026–1043 CrossRef PubMed.
- W. Chen, J. Ouyang, X. Yi, Y. Xu, C. Niu, W. Zhang, L. Wang, J. Sheng, L. Deng, Y.-N. Liu and S. Guo, Black Phosphorus Nanosheets as a Neuroprotective Nanomedicine for Neurodegenerative Disorder Therapy, Adv. Mater., 2018, 30(3), 1703458 CrossRef PubMed.
- Y. Zhu, Y. Liu, Z. Xie, T. He, L. Su, F. Guo, G. Arkin, X. Lai, J. Xu and H. Zhang, Magnetic black phosphorus microbubbles for targeted tumor theranostics, Nanophotonics, 2021, 10(12), 3339–3358 CrossRef CAS.
- Y. J. Lim, W. h. Zhou, G. Li, Z. W. Hu, L. Hong, X. F. Yu and Y. M. Li, Black Phosphorus Nanomaterials Regulate the Aggregation of Amyloid-β, ChemNanoMat, 2019, 5(5), 606–611 CrossRef CAS.
- J. Yang, W. Liu, Y. Sun and X. Dong, LVFFARK-PEG-Stabilized Black Phosphorus Nanosheets Potently Inhibit Amyloid-beta Fibrillogenesis, Langmuir, 2020, 36(7), 1804–1812 CrossRef CAS PubMed.
- C. Du, W. Feng, X. Dai, J. Wang, D. Geng, X. Li, Y. Chen and J. Zhang, Cu(2+)-Chelatable and ROS-Scavenging MXenzyme as NIR-II-Triggered Blood–Brain Barrier-Crossing Nanocatalyst against Alzheimer's Disease, Small, 2022, 18(39), e2203031 CrossRef PubMed.
- W. Chen, J. Ouyang, X. Yi, Y. Xu, C. Niu, W. Zhang, L. Wang, J. Sheng, L. Deng, Y. N. Liu and S. Guo, Black Phosphorus Nanosheets as a Neuroprotective Nanomedicine for Neurodegenerative Disorder Therapy, Adv. Mater., 2018, 30(3), 1703458 CrossRef.
- P. Chinna Ayya Swamy, G. Sivaraman, R. N. Priyanka, S. O. Raja, K. Ponnuvel, J. Shanmugpriya and A. Gulyani, Near Infrared (NIR) absorbing dyes as promising photosensitizer for photo dynamic therapy, Coord. Chem. Rev., 2020, 411, 213233 CrossRef CAS.
- Y. Wang, J. Li, X. Li, J. Shi, Z. Jiang and C. Y. Zhang, Graphene-based nanomaterials for cancer therapy and anti-infections, Bioact. Mater., 2022, 14, 335–349 CrossRef CAS PubMed.
- Q. Kong, B. Ma, T. Yu, C. Hu, G. Li, Q. Jiang and Y. Wang, A two-photon AIE fluorophore as a photosensitizer for highly efficient mitochondria-targeted photodynamic therapy, New J. Chem., 2020, 44(22), 9355–9364 RSC.
- H. Wang, X. Yang, W. Shao, S. Chen, J. Xie, X. Zhang, J. Wang and Y. Xie, Ultrathin Black Phosphorus Nanosheets for Efficient Singlet Oxygen Generation, J. Am. Chem. Soc., 2015, 137(35), 11376–11382 CrossRef CAS PubMed.
- Y. Li, Z. Du, X. Liu, M. Ma, D. Yu, Y. Lu, J. Ren and X. Qu, Near-Infrared Activated Black Phosphorus as a Nontoxic Photo-Oxidant for Alzheimer's Amyloid-beta Peptide, Small, 2019, 15(24), e1901116 CrossRef.
- Y. Liu, Z. Qiu, A. Carvalho, Y. Bao, H. Xu, S. J. Tan, W. Liu, A. H. Castro Neto, K. P. Loh and J. Lu, Gate-Tunable Giant Stark Effect in Few-Layer Black Phosphorus, Nano Lett., 2017, 17(3), 1970–1977 CrossRef PubMed.
- R. Gusmao, Z. Sofer and M. Pumera, Black Phosphorus Rediscovered: From Bulk Material to Monolayers, Angew. Chem., Int. Ed., 2017, 56(28), 8052–8072 CrossRef.
- X. Yang, G. Liu, Y. Shi, W. Huang, J. Shao and X. Dong, Nano-black phosphorus for combined cancer phototherapy: recent advances and prospects, Nanotechnology, 2018, 29(22), 222001 CrossRef PubMed.
- Y. Chen and M. Sun, Two-dimensional WS2/MoS2 heterostructures: properties and applications, Nanoscale, 2021, 13(11), 5594–5619 RSC.
- Y. Zhu, Y. Wang, G. R. Williams, L. Fu, J. Wu, H. Wang, R. Liang, X. Weng and M. Wei, Multicomponent Transition Metal Dichalcogenide Nanosheets for Imaging-Guided Photothermal and Chemodynamic Therapy, Adv. Sci., 2020, 7(23), 2000272 CrossRef PubMed.
- H. Chen, T. Liu, Z. Su, L. Shang and G. Wei, 2D transition metal dichalcogenide nanosheets for photo/thermo-based tumor imaging and therapy, Nanoscale Horiz., 2018, 3(2), 74–89 RSC.
- H. Zhu, R. Jin, Y. C. Chang, J. J. Zhu, D. Jiang, Y. Lin and W. Zhu, Understanding the Synergistic Oxidation in Dichalcogenides through Electrochemiluminescence Blinking at Millisecond Resolution, Adv. Mater., 2021, 33(48), e2105039 CrossRef PubMed.
- X. Zhang, Z. Lai, Q. Ma and H. Zhang, Novel structured transition metal dichalcogenide nanosheets, Chem. Soc. Rev., 2018, 47(9), 3301–3338 RSC.
- Q. Peng, Z. Qian, H. Gao and K. Zhang, Recent Advances in Transition-Metal Based Nanomaterials for Noninvasive Oncology Thermal Ablation and Imaging Diagnosis, Front. Chem., 2022, 10, 899321 CrossRef CAS PubMed.
- S. S. Chou, B. Kaehr, J. Kim, B. M. Foley, M. De, P. E. Hopkins, J. Huang, C. J. Brinker and V. P. Dravid, Chemically exfoliated MoS2 as near-infrared photothermal agents, Angew. Chem., Int. Ed., 2013, 52(15), 4160–4164 CrossRef CAS PubMed.
- J. Wang, L. Liu, D. Ge, H. Zhang, Y. Feng, Y. Zhang, M. Chen and M. Dong, Differential Modulating Effect of MoS2 on Amyloid Peptide Assemblies, Chemistry, 2018, 24(14), 3397–3402 CrossRef CAS PubMed.
- S. K. Mudedla, N. A. Murugan, V. Subramanian and H. Agren, Destabilization of amyloid fibrils on interaction with MoS2-based nanomaterials, RSC Adv., 2019, 9(3), 1613–1624 RSC.
- Y. Liu, Y. Zheng, S. Li, J. Li, X. Du, Y. Ma, G. Liao, Q. Wang, X. Yang and K. Wang, Contradictory effect of gold nanoparticle-decorated molybdenum sulfide nanocomposites on amyloid-β-40 aggregation, Chin. Chem. Lett., 2020, 31(12), 3113–3116 CrossRef CAS.
- X. Wang, Q. Han, X. Liu, C. Wang and R. Yang, Multifunctional inhibitors of beta-amyloid aggregation based on MoS2/AuNR nanocomposites with high near-infrared absorption, Nanoscale, 2019, 11(18), 9185–9193 RSC.
- M. Ma, Y. Wang, N. Gao, X. Liu, Y. Sun, J. Ren and X. Qu, A Near-Infrared-Controllable Artificial Metalloprotease Used for Degrading Amyloid-beta Monomers and Aggregates, Chemistry, 2019, 25(51), 11852–11858 CrossRef PubMed.
- M. Li, A. Zhao, K. Dong, W. Li, J. Ren and X. Qu, Chemically exfoliated WS2 nanosheets efficiently inhibit amyloid β-peptide aggregation and can be used for photothermal treatment of Alzheimer's disease, Nano Res., 2015, 8(10), 3216–3227 CrossRef.
- R. Maleki, M. Khedri, S. Rezvantalab, F. Afsharchi, K. Musaie, S. Shafiee and M. A. Shahbazi, beta-Amyloid Targeting with Two-Dimensional Covalent Organic Frameworks: Multi-Scale In-Silico Dissection of Nano-Biointerface, Chembiochem, 2021, 22(13), 2306–2318 CrossRef PubMed.
- N. Sorout and A. Chandra, Interactions of the Abeta(1-42) Peptide with Boron Nitride Nanoparticles of Varying Curvature in an Aqueous Medium: Different Pathways to Inhibit beta-Sheet Formation, J. Phys. Chem. B, 2021, 125(40), 11159–11178 CrossRef PubMed.
- O. S. Bull, I. Bull, G. K. Amadi and C. O. Odu, Covalent Organic Frameworks (COFs): A Review, J. Appl. Sci. Environ. Manage., 2022, 26(1), 145–179 Search PubMed.
- N. Sorout and A. Chandra, Effects of Boron Nitride Nanotube on the Secondary Structure of Abeta(1-42) Trimer: Possible Inhibitory Effect on Amyloid Formation, J. Phys. Chem. B, 2020, 124(10), 1928–1940 CrossRef CAS PubMed.
- O. C. Yildirim, M.
E. Arslan, S. Oner, I. Cacciatore, A. Di Stefano, A. Mardinoglu and H. Turkez, Boron Nitride Nanoparticles Loaded with a Boron-Based Hybrid as a Promising Drug Carrier System for Alzheimer’s Disease Treatment, Int. J. Mol. Sci., 2022, 23(15), 8249 CrossRef PubMed.
- N. Aydin, H. Turkez, O. O. Tozlu, M. E. Arslan, M. Yavuz, E. Sonmez, O. F. Ozpolat, I. Cacciatore, A. Di Stefano and A. Mardinoglu, Ameliorative Effects by Hexagonal Boron Nitride Nanoparticles against Beta Amyloid Induced Neurotoxicity, Nanomaterials, 2022, 12(15), 2690 CrossRef CAS PubMed.
- Z. Wang, T. Hu, R. Liang and M. Wei, Application of Zero-Dimensional Nanomaterials in Biosensing, Front. Chem., 2020, 8, 320 CrossRef CAS PubMed.
- Y. Liu, Q. Yu, J. Chang and C. Wu, Nanobiomaterials: from 0D to 3D for tumor therapy and tissue regeneration, Nanoscale, 2019, 11(29), 13678–13708 RSC.
- J. Xie, Z. Shen, Y. Anraku, K. Kataoka and X. Chen, Nanomaterial-based blood–brain-barrier (BBB) crossing strategies, Biomaterials, 2019, 224, 119491 CrossRef CAS PubMed.
- M. A. Busquets, A. Espargaró, R. Sabaté and J. Estelrich, Magnetic Nanoparticles Cross the Blood–Brain Barrier: When Physics Rises to a Challenge, Nanomaterials, 2015, 5(4), 2231–2248 CrossRef PubMed.
- S. M. Lombardo, M. Schneider, A. E. Türeli and N. Günday Türeli, Key for crossing the BBB with nanoparticles: the rational design, Beilstein J. Nanotechnol., 2020, 11, 866–883 CrossRef PubMed.
- Z. Yang, D. Wang, C. Zhang, H. Liu, M. Hao, S. Kan, D. Liu and W. Liu, The Applications of Gold Nanoparticles in the Diagnosis and Treatment of Gastrointestinal Cancer, Front. Oncol., 2021, 11, 819329 CrossRef PubMed.
- W. Zhu, R. Michalsky, O. Metin, H. Lv, S. Guo, C. J. Wright, X. Sun, A. A. Peterson and S. Sun, Monodisperse Au nanoparticles for selective electrocatalytic reduction of CO2 to CO, J. Am. Chem. Soc., 2013, 135(45), 16833–16836 CrossRef PubMed.
- G. d. B. Silveira, A. P. Muller, R. A. Machado-de-Ávila and P. C. L. Silveira, Advance in the use of gold nanoparticles in the treatment of neurodegenerative diseases: new perspectives, Neural Regener. Res., 2021, 16(12), 2425–2426 CrossRef PubMed.
- Y. H. Liao, Y. J. Chang, Y. Yoshiike, Y. C. Chang and Y. R. Chen, Negatively charged gold nanoparticles inhibit Alzheimer's amyloid-beta fibrillization, induce fibril dissociation, and mitigate neurotoxicity, Small, 2012, 8(23), 3631–3639 CrossRef CAS PubMed.
- W. Wang, Y. Han, Y. Fan and Y. Wang, Effects of Gold Nanospheres and Nanocubes on Amyloid-beta Peptide Fibrillation, Langmuir, 2019, 35(6), 2334–2342 CrossRef CAS PubMed.
- A. Tapia-Arellano, E. Gallardo-Toledo, F. Celis, R. Rivera, I. Moglia, M. Campos, N. Carulla, M. Baez and M. J. Kogan, The curvature of gold nanoparticles influences the exposure of amyloid-beta and modulates its aggregation process, Mater. Sci. Eng., C, 2021, 128, 112269 CrossRef CAS PubMed.
- F. Tavanti, A. Pedone, M. C. Menziani and A. Alexander-Katz, Computational Insights into the Binding of Monolayer-Capped Gold Nanoparticles onto Amyloid-beta Fibrils, ACS Chem. Neurosci., 2020, 11(19), 3153–3160 CrossRef CAS PubMed.
- T. John, A. Gladytz, C. Kubeil, L. L. Martin, H. J. Risselada and B. Abel, Impact of nanoparticles on amyloid peptide and protein aggregation: a review with a focus on gold nanoparticles, Nanoscale, 2018, 10(45), 20894–20913 RSC.
- G. Wang, J. Dai and X. Lu, Scutellaria barbata Leaf Extract Mediated Gold Nanoparticles for Alzheimer's Disease Treatment by Metal-Induced Amyloid β Aggregation Inhibition, J. Cluster Sci., 2019, 31(6), 1269–1273 CrossRef.
- B. G. Anand, Q. Wu, G. Karthivashan, K. P. Shejale, S. Amidian, H. Wille and S. Kar, Mimosine functionalized gold nanoparticles (mimo-AuNPs) suppress beta-amyloid aggregation and neuronal toxicity, Bioact. Mater., 2021, 6(12), 4491–4505 CrossRef CAS PubMed.
- N. Xiong, Y. Zhao, X. Dong, J. Zheng and Y. Sun, Design of a Molecular Hybrid of Dual Peptide Inhibitors Coupled on AuNPs for Enhanced Inhibition of Amyloid beta-Protein Aggregation and Cytotoxicity, Small, 2017, 13(13), 1601666 CrossRef PubMed.
- Y. Zheng, J. Wu, H. Jiang and X. Wang, Gold nanoclusters for theranostic applications, Coord. Chem. Rev., 2021, 431, 213689 CrossRef CAS.
- G. Gao, M. Zhang, D. Gong, R. Chen, X. Hu and T. Sun, The size-effect of gold nanoparticles and nanoclusters in the inhibition of amyloid-beta fibrillation, Nanoscale, 2017, 9(12), 4107–4113 RSC.
- P. Shi, M. Li, J. Ren and X. Qu, Gold Nanocage-Based Dual Responsive “Caged Metal Chelator” Release System: Noninvasive Remote Control with Near Infrared for Potential Treatment of Alzheimer's Disease, Adv. Funct. Mater., 2013, 23(43), 5412–5419 CrossRef CAS.
- W. Zhang, G. Gao, Z. Ma, Z. Luo, M. He and T. Sun, Au23(CR)14 nanocluster restores fibril Abeta's unfolded state with abolished cytotoxicity and dissolves endogenous Abeta plaques, Natl. Sci. Rev., 2020, 7(4), 763–774 CrossRef CAS PubMed.
- S. Hao, X. Li, A. Han, Y. Yang, G. Fang, J. Liu and S. Wang, CLVFFA-Functionalized Gold Nanoclusters Inhibit Abeta40 Fibrillation, Fibrils' Prolongation, and Mature Fibrils' Disaggregation, ACS Chem. Neurosci., 2019, 10(11), 4633–4642 CrossRef CAS PubMed.
- S. Saleem, M. H. Jameel, N. Akhtar, N. Nazir, A. Ali, A. Zaman, A. Rehman, S. Butt, F. Sultana, M. Mushtaq, J. H. Zeng, M. Amami and K. Althubeiti, Modification in structural, optical, morphological, and electrical properties of zinc oxide (ZnO) nanoparticles (NPs) by metal (Ni, Co) dopants for electronic device applications, Arabian J. Chem., 2022, 15(1), 103518 CrossRef CAS.
- A. A. Oun, S. Shankar and J. W. Rhim, Multifunctional nanocellulose/metal and metal oxide nanoparticle hybrid nanomaterials, Crit. Rev. Food Sci. Nutr., 2020, 60(3), 435–460 CrossRef CAS PubMed.
- S. Anjum, M. Hashim, S. A. Malik, M. Khan, J. M. Lorenzo, B. H. Abbasi and C. Hano, Recent Advances in Zinc Oxide Nanoparticles (ZnO NPs) for Cancer Diagnosis, Target Drug Delivery, and Treatment, Cancers, 2021, 13(18), 4570 CrossRef CAS PubMed.
- M. Li, P. Shi, C. Xu, J. Ren and X. Qu, Cerium oxide caged metal chelator: anti-aggregation and anti-oxidation integrated H2O2-responsive controlled drug release for potential Alzheimer's disease treatment, Chem. Sci., 2013, 4(6), 2536–2542 RSC.
- Y. Guan, M. Li, K. Dong, N. Gao, J. Ren, Y. Zheng and X. Qu, Ceria/POMs hybrid nanoparticles as a mimicking metallopeptidase for treatment of neurotoxicity of amyloid-beta peptide, Biomaterials, 2016, 98, 92–102 CrossRef CAS PubMed.
- Y. Guan, N. Gao, J. Ren and X. Qu, Rationally Designed CeNP@MnMoS4 Core–Shell Nanoparticles for Modulating Multiple Facets of Alzheimer's Disease, Chemistry, 2016, 22(41), 14523–14526 CrossRef PubMed.
- K. Ge, Y. Mu, M. Liu, Z. Bai, Z. Liu, D. Geng and F. Gao, Gold Nanorods with Spatial Separation
of CeO2 Deposition for Plasmonic-Enhanced Antioxidant Stress and Photothermal Therapy of Alzheimer's Disease, ACS Appl. Mater. Interfaces, 2022, 14(3), 3662–3674 CrossRef CAS PubMed.
- E. Esmaeili, M. Khalili, A. N. Sohi, S. Hosseinzadeh, B. Taheri and M. Soleimani, Dendrimer functionalized magnetic nanoparticles as a promising platform for localized hyperthermia and magnetic resonance imaging diagnosis, J. Cell. Physiol., 2019, 234(8), 12615–12624 CrossRef CAS PubMed.
- M. Mahmoudi, F. Quinlan-Pluck, M. P. Monopoli, S. Sheibani, H. Vali, K. A. Dawson and I. Lynch, Influence of the Physiochemical Properties of Superparamagnetic Iron Oxide Nanoparticles on Amyloid β Protein Fibrillation in Solution, ACS Chem. Neurosci., 2013, 4(3), 475–485 CrossRef CAS PubMed.
- D. Brambilla, B. Le Droumaguet, J. Nicolas, S. H. Hashemi, L. P. Wu, S. M. Moghimi, P. Couvreur and K. Andrieux, Nanotechnologies for Alzheimer's disease: diagnosis, therapy, and safety issues, Nanomedicine, 2011, 7(5), 521–540 CrossRef CAS PubMed.
- Z. Du, N. Gao, Y. Guan, C. Ding, Y. Sun, J. Ren and X. Qu, Rational design of a “sense and treat” system to target amyloid aggregates related to Alzheimer's disease, Nano Res., 2018, 11(4), 1987–1997 CrossRef CAS.
- E. Halevas, B. Mavroidi, C. M. Nday, J. Tang, G. C. Smith, N. Boukos, G. Litsardakis, M. Pelecanou and A. Salifoglou, Modified magnetic core-shell mesoporous silica nano-formulations with encapsulated quercetin exhibit anti-amyloid and antioxidant activity, J. Inorg. Biochem., 2020, 213, 111271 CrossRef CAS PubMed.
- E. Dyne, P. S. Prakash, J. Li, B. Yu, T.-L. Schmidt, S. Huang and M.-H. Kim, Mild magnetic nanoparticle hyperthermia promotes the disaggregation and microglia-mediated clearance of beta-amyloid plaques, Nanomedicine, 2021, 34, 102397 CrossRef CAS PubMed.
- D. Oleshchuk, P. Šálek, J. Dvořáková, J. Kučka, E. Pavlova, P. Francová, L. Šefc and V. Proks, Biocompatible polypeptide nanogel: effect of surfactants on nanogelation in inverse miniemulsion, in vivo biodistribution and blood clearance evaluation, Mater. Sci. Eng. C, 2021, 126, 111865 CrossRef CAS PubMed.
- Z. Sun, H. Xie, S. Tang, X. F. Yu, Z. Guo, J. Shao, H. Zhang, H. Huang, H. Wang and P. K. Chu, Ultrasmall Black Phosphorus Quantum Dots: Synthesis and Use as Photothermal Agents, Angew. Chem., Int. Ed., 2015, 54(39), 11526–11530 CrossRef CAS PubMed.
- S. Wang, C. Li, Y. Xia, S. Chen, J. Robert, X. Banquy, R. Huang, W. Qi, Z. He and R. Su, Nontoxic Black Phosphorus Quantum Dots Inhibit Insulin Amyloid Fibrillation at an Ultralow Concentration, iScience, 2020, 23(5), 101044 CrossRef CAS PubMed.
- Y. Bu, M. Zhang, J. Fu, X. Yang and S. Liu, Black phosphorous quantum dots for signal-on cathodic photoelectrochemical aptasensor monoitoring amyloid beta peptide, Anal. Chim. Acta, 2022, 1189, 339200 CrossRef CAS PubMed.
- C. Sweet, A. Pramanik, S. Jones and P. C. Ray, Two-Photon Fluorescent Molybdenum Disulfide Dots for Targeted Prostate Cancer Imaging in the Biological II Window, ACS Omega, 2017, 2(5), 1826–1835 CrossRef CAS PubMed.
- J. Chen, Y. Li, Y. Huang, H. Zhang, X. Chen and H. Qiu, Fluorometric dopamine assay based on an energy transfer system composed of aptamer-functionalized MoS2 quantum dots and MoS2 nanosheets, Mikrochim. Acta, 2019, 186(2), 58 CrossRef PubMed.
- L. J. Sun, L. Qu, R. Yang, L. Yin and H. J. Zeng, Cysteamine functionalized MoS2 quantum
dots inhibit amyloid aggregation, Int. J. Biol. Macromol., 2019, 128, 870–876 CrossRef CAS PubMed.
- Y. Li, H. Tang, H. Zhu, A. Kakinen, D. Wang, N. Andrikopoulos, Y. Sun, A. Nandakumar, E. Kwak, T. P. Davis, D. T. Leong, F. Ding and P. C. Ke, Ultrasmall Molybdenum Disulfide Quantum Dots Cage Alzheimer's Amyloid Beta to Restore Membrane Fluidity, ACS Appl. Mater. Interfaces, 2021, 13(25), 29936–29948 CrossRef CAS PubMed.
- X. Tian, Y. Sun, S. Fan, M. D. Boudreau, C. Chen, C. Ge and J. J. Yin, Photogenerated Charge Carriers in Molybdenum Disulfide Quantum Dots with Enhanced Antibacterial Activity, ACS Appl. Mater. Interfaces, 2019, 11(5), 4858–4866 CrossRef CAS PubMed.
- L. Y. Cruz, D. Wang and J. Liu, Biosynthesis of selenium nanoparticles, characterization and X-ray induced radiotherapy for the treatment of lung cancer with interstitial lung disease, J. Photochem. Photobiol., B, 2019, 191, 123–127 CrossRef CAS PubMed.
- Y. Qi, P. Yi, T. He, X. Song, Y. Liu, Q. Li, J. Zheng, R. Song, C. Liu, Z. Zhang, W. Peng and Y. Zhang, Quercetin-loaded selenium nanoparticles inhibit amyloid-β aggregation and exhibit antioxidant activity, Colloids Surf., A, 2020, 602, 125058 CrossRef CAS.
- X. Guo, Q. Lie, Y. Liu, Z. Jia, Y. Gong, X. Yuan and J. Liu, Multifunctional Selenium Quantum Dots for the Treatment of Alzheimer's Disease by Reducing Abeta-Neurotoxicity and Oxidative Stress and Alleviate Neuroinflammation, ACS Appl. Mater. Interfaces, 2021, 13(26), 30261–30273 CrossRef CAS PubMed.
- J. Liu, R. Li and B. Yang, Carbon Dots: A New Type of Carbon-Based Nanomaterial with Wide Applications, ACS Cent. Sci., 2020, 6(12), 2179–2195 CrossRef CAS PubMed.
- Q. Zeng, T. Feng, S. Tao, S. Zhu and B. Yang, Precursor-dependent structural diversity in luminescent carbonized polymer dots (CPDs): the nomenclature, Light: Sci. Appl., 2021, 10(1), 142 CrossRef CAS PubMed.
- Y. R. Kumar, K. Deshmukh, K. K. Sadasivuni and S. K. K. Pasha, Graphene quantum dot based materials for sensing, bio-imaging and energy storage applications: a review, RSC Adv., 2020, 10(40), 23861–23898 RSC.
- D. Iannazzo, I. Ziccarelli and A. Pistone, Graphene quantum dots: multifunctional nanoplatforms for anticancer therapy, J. Mater. Chem. B, 2017, 5(32), 6471–6489 RSC.
- Y. Liu, L. P. Xu, W. Dai, H. Dong, Y. Wen and X. Zhang, Graphene quantum dots for the inhibition of beta amyloid aggregation, Nanoscale, 2015, 7(45), 19060–19065 RSC.
- S. Xiao, D. Zhou, P. Luan, B. Gu, L. Feng, S. Fan, W. Liao, W. Fang, L. Yang, E. Tao, R. Guo and J. Liu, Graphene quantum dots conjugated neuroprotective peptide improve learning and memory capability, Biomaterials, 2016, 106, 98–110 CrossRef CAS PubMed.
- M. Yousaf, H. Huang, P. Li, C. Wang and Y. Yang, Fluorine Functionalized Graphene Quantum Dots as Inhibitor against hIAPP Amyloid Aggregation, ACS Chem. Neurosci., 2017, 8(6), 1368–1377 CrossRef CAS PubMed.
- H.-j. Zeng, M. Miao, Z. Liu, R. Yang and L.-b. Qu, Effect of nitrogen-doped graphene quantum dots on the fibrillation of hen egg-white lysozyme, Int. J. Biol. Macromol., 2017, 95, 856–861 CrossRef CAS PubMed.
- Y. Liu, L. P. Xu, Q. Wang, B. Yang and X. Zhang, Synergistic Inhibitory Effect of GQDs-Tramiprosate Covalent Binding on Amyloid Aggregation, ACS Chem. Neurosci., 2018, 9(4), 817–823 CrossRef CAS PubMed.
- Y. Wang, U. Kadiyala, Z. Qu, P. Elvati, C. Altheim, N. A. Kotov, A. Violi and J. S. VanEpps, Anti-Biofilm Activity of Graphene Quantum Dots via Self-Assembly with Bacterial Amyloid Proteins, ACS Nano, 2019, 13(4), 4278–4289 CrossRef CAS PubMed.
- C. Liu, H. Huang, L. Ma, X. Fang, C. Wang and Y. Yang, Modulation of beta-amyloid aggregation by graphene quantum dots, R. Soc. Open Sci., 2019, 6(6), 190271 CrossRef CAS PubMed.
- K. Tak, R. Sharma, V. Dave, S. Jain and S. Sharma,
Clitoria ternatea Mediated Synthesis of Graphene Quantum Dots for the Treatment of Alzheimer's Disease, ACS Chem. Neurosci., 2020, 11(22), 3741–3748 CrossRef CAS PubMed.
- G. Perini, V. Palmieri, G. Ciasca, M. De Spirito and M. Papi, Unravelling the Potential of Graphene Quantum Dots in Biomedicine and Neuroscience, Int. J. Mol. Sci., 2020, 21(10), 3712 CrossRef CAS PubMed.
- E. R. Ghareghozloo, M. Mahdavimehr, A. A. Meratan, N. Nikfarjam, A. Ghasemi, B. Katebi and M. Nemat-Gorgani, Role of surface oxygen-containing functional groups of graphene oxide quantum dots on amyloid fibrillation of two model proteins, Plos One, 2020, 15(12), e0244296 CrossRef PubMed.
- H. Huang, P. Li, M. Zhang, Y. Yu, Y. Huang, H. Gu, C. Wang and Y. Yang, Graphene quantum dots for detecting monomeric amyloid peptides, Nanoscale, 2017, 9(16), 5044–5048 RSC.
- M. Yousaf, M. Ahmad, I. A. Bhatti, A. Nasir, M. Hasan, X. Jian, K. Kalantar-Zadeh and N. Mahmood,
In Vivo and In Vitro Monitoring of Amyloid Aggregation via BSA@FGQDs Multimodal Probe, ACS Sens., 2019, 4(1), 200–210 CrossRef PubMed.
- H. Tang, Y. Li, A. Kakinen, N. Andrikopoulos, Y. Sun, E. Kwak, T. P. Davis, F. Ding and P. C. Ke, Graphene quantum dots obstruct the membrane axis of Alzheimer's amyloid beta, Phys. Chem. Chem. Phys., 2021, 24(1), 86–97 RSC.
- C. Lai, S. Lin, X. Huang and Y. Jin, Synthesis and properties of carbon quantum dots and their research progress in cancer treatment, Dyes Pigm., 2021, 196, 109766 CrossRef.
- U. A. Rani, L. Y. Ng, C. Y. Ng and E. Mahmoudi, A review of carbon quantum dots and their applications in wastewater treatment, Adv. Colloid Interface Sci., 2020, 278, 102124 CrossRef PubMed.
- C. Wang, M. Yang, H. Shi, Z. Yao, E. Liu, X. Hu, P. Guo, W. Xue and J. Fan, Carbon quantum dots prepared by pyrolysis: investigation of the luminescence mechanism and application as fluorescent probes, Dyes Pigm., 2022, 204, 110431 CrossRef CAS.
- Z. Zhu, Y. Zhai, Z. Li, P. Zhu, S. Mao, C. Zhu, D. Du, L. A. Belfiore, J. Tang and Y. Lin, Red carbon dots: optical property regulations and applications, Mater. Today, 2019, 30, 52–79 CrossRef CAS.
- A. Voronova, A. Barras, V. Plaisance, V. Pawlowski, R. Boukherroub, A. Abderrahmani and S. Szunerits, Anti-aggregation effect of carbon quantum dots on diabetogenic and beta-cell cytotoxic amylin and beta amyloid heterocomplexes, Nanoscale, 2022, 14(39), 14683–14694 RSC.
- S. Li, L. Wang, C. C. Chusuei, V. M. Suarez, P. L. Blackwelder, M. Micic, J. Orbulescu and R. M. Leblanc, Nontoxic Carbon Dots Potently Inhibit Human
Insulin Fibrillation, Chem. Mater., 2015, 27(5), 1764–1771 CrossRef.
- Q. Q. Yang, J. C. Jin, Z. Q. Xu, J. Q. Zhang, B. B. Wang, F. L. Jiang and Y. Liu, Active site-targeted carbon dots for the inhibition of human insulin fibrillation, J. Mater. Chem. B, 2017, 5(10), 2010–2018 RSC.
- R. Malishev, E. Arad, S. K. Bhunia, S. Shaham-Niv, S. Kolusheva, E. Gazit and R. Jelinek, Chiral modulation of amyloid beta fibrillation and cytotoxicity by enantiomeric carbon dots, Chem. Commun., 2018, 54(56), 7762–7765 RSC.
- K. Koppel, H. Tang, I. Javed, M. Parsa, M. Mortimer, T. P. Davis, S. Lin, A. L. Chaffee, F. Ding and P. C. Ke, Elevated amyloidoses of human IAPP and amyloid beta by lipopolysaccharide and their mitigation by carbon quantum dots, Nanoscale, 2020, 12(23), 12317–12328 RSC.
- X. Zhou, S. Hu, S. Wang, Y. Pang, Y. Lin and M. Li, Large Amino Acid Mimicking Selenium-Doped Carbon Quantum Dots for Multi-Target Therapy of Alzheimer's Disease, Front. Pharmacol., 2021, 12, 778613 CrossRef PubMed.
- F. Li, T. Li, C. Sun, J. Xia, Y. Jiao and H. Xu, Selenium-Doped Carbon Quantum Dots for Free-Radical Scavenging, Angew. Chem., Int. Ed., 2017, 56(33), 9910–9914 CrossRef CAS PubMed.
- E. Damian Guerrero, A. M. Lopez-Velazquez, J. Ahlawat and M. Narayan, Carbon Quantum Dots for Treatment of Amyloid Disorders, ACS Appl. Nano Mater., 2021, 4(3), 2423–2433 CrossRef PubMed.
- H. Li, Y. Zhang, J. Ding, T. Wu, S. Cai, W. Zhang, R. Cai, C. Chen and R. Yang, Synthesis of carbon quantum dots for application of alleviating amyloid-beta mediated neurotoxicity, Colloids Surf., B, 2022, 212, 112373 CrossRef CAS PubMed.
- C. Zhang, X. Wang, J. Du, Z. Gu and Y. Zhao, Reactive Oxygen Species-Regulating Strategies Based on Nanomaterials for Disease Treatment, Adv. Sci., 2021, 8(3), 2002797 CrossRef CAS PubMed.
- S.-S. Wan, J.-Y. Zeng, H. Cheng and X.-Z. Zhang, ROS-induced NO generation for gas therapy and sensitizing photodynamic therapy of tumor, Biomaterials, 2018, 185, 51–62 CrossRef CAS PubMed.
- W. Liu, X. Dong, Y. Liu and Y. Sun, Photoresponsive materials for intensified modulation of Alzheimer's amyloid-beta protein aggregation: a review, Acta Biomater., 2021, 123, 93–109 CrossRef CAS PubMed.
- J. Yu, X. Yong, Z. Tang, B. Yang and S. Lu, Theoretical Understanding of Structure–Property Relationships in Luminescence of Carbon Dots, J. Phys. Chem. Lett., 2021, 12(32), 7671–7687 CrossRef CAS PubMed.
- Y. J. Chung, C. H. Lee, J. Lim, J. Jang, H. Kang and C. B. Park, Photomodulating Carbon Dots for Spatiotemporal Suppression of Alzheimer's beta-Amyloid Aggregation, ACS Nano, 2020, 14(12), 16973–16983 CrossRef CAS PubMed.
- B. I. Lee, Y. J. Chung and C. B. Park, Photosensitizing materials and platforms for light-triggered modulation of Alzheimer's beta-amyloid self-assembly, Biomaterials, 2019, 190–191, 121–132 CrossRef CAS PubMed.
- Y. J. Chung, K. Kim, B. I. Lee and C. B. Park, Carbon Nanodot-Sensitized Modulation of Alzheimer's beta-Amyloid Self-Assembly, Disassembly, and Toxicity, Small, 2017, 13(34), 1700983 CrossRef PubMed.
- Y. J. Chung, B. I. Lee and C. B. Park, Multifunctional carbon dots as a therapeutic nanoagent for modulating Cu(II)-mediated beta-amyloid aggregation, Nanoscale, 2019, 11(13), 6297–6306 RSC.
- W. Gao, W. Wang, X. Dong and Y. Sun, Nitrogen-Doped Carbonized Polymer Dots: A Potent Scavenger and Detector Targeting Alzheimer's beta-Amyloid Plaques, Small, 2020, 16(43), e2002804 CrossRef PubMed.
- M. H. Melchor, F. G. Susana, G. S. Francisco, I. B. Hiram, R. F. Norma, A. L. Jorge, Y. L. Perla and B. I. Gustavo, Fullerenemalonates inhibit amyloid beta aggregation, in vitro and in silico evaluation, RSC Adv., 2018, 8(69), 39667–39677 RSC.
- Z. Markovic and V. Trajkovic, Biomedical potential of the reactive oxygen species generation and quenching by fullerenes (C60), Biomaterials, 2008, 29(26), 3561–3573 CrossRef PubMed.
- Z. Du, N. Gao, X. Wang, J. Ren and X. Qu, Near-Infrared Switchable Fullerene-Based Synergy Therapy for Alzheimer's Disease, Small, 2018, 14, e1801852 CrossRef PubMed.
- A. G. Bobylev, O. A. Kraevaya, L. G. Bobyleva, E. A. Khakina, R. S. Fadeev, A. V. Zhilenkov, D. V. Mishchenko, N. V. Penkov, I. Y. Teplov, E. I. Yakupova, I. M. Vikhlyantsev and P. A. Troshin, Anti-amyloid activities of three different types of water-soluble fullerene derivatives, Colloids Surf., B, 2019, 183, 110426 CrossRef PubMed.
- J. Wang, Y. Fan, Y. Tan, X. Zhao, Y. Zhang, C. Cheng and M. Yang, Porphyrinic Metal-Organic Framework PCN-224 Nanoparticles for Near-Infrared-Induced Attenuation of Aggregation and Neurotoxicity of Alzheimer's Amyloid-beta Peptide, ACS Appl. Mater. Interfaces, 2018, 10(43), 36615–36621 CrossRef PubMed.
- J. Geng, M. Li, J. Ren, E. Wang and X. Qu, Polyoxometalates as inhibitors of the aggregation of amyloid beta peptides associated with Alzheimer's disease, Angew. Chem., Int. Ed., 2011, 50(18), 4184–4188 CrossRef PubMed.
- S. Mourtas, M. Canovi, C. Zona, D. Aurilia, A. Niarakis, B. La Ferla, M. Salmona, F. Nicotra, M. Gobbi and S. G. Antimisiaris, Curcumin-decorated nanoliposomes with very high affinity for amyloid-beta1-42 peptide, Biomaterials, 2011, 32(6), 1635–1645 CrossRef CAS PubMed.
- M. Hulsemann, C. Zafiu, K. Kuhbach, N. Luhmann, Y. Herrmann, L. Peters, C. Linnartz, J. Willbold, K. Kravchenko, A. Kulawik, S. Willbold, O. Bannach and D. Willbold, Biofunctionalized Silica Nanoparticles: Standards in Amyloid-beta Oligomer-Based Diagnosis of Alzheimer's Disease, J. Alzheimer's Dis., 2016, 54(1), 79–88 Search PubMed.
- J. Ji, W. Lou and P. Shen, Modular design in metal–organic frameworks for oxygen evolution reaction, Int. J. Hydrogen Energy, 2022, 47(93), 39443–39469 CrossRef CAS.
- J. Wang, Y. Fan, H.-w. Lee, C. Yi, C. Cheng, X. Zhao and M. Yang, Ultrasmall Metal-Organic Framework Zn-MOF-74 Nanodots: Size-Controlled Synthesis and Application for Highly Selective Colorimetric Sensing of Iron(III) in Aqueous Solution, ACS Appl. Nano Mater., 2018, 1(7), 3747–3753 CrossRef CAS.
- B. Wang, X. L. Lv, D. Feng, L. H. Xie, J. Zhang, M. Li, Y. Xie, J. R. Li and H. C. Zhou, Highly Stable Zr(IV)-Based Metal–Organic Frameworks for the Detection and Removal of Antibiotics and Organic Explosives in Water, J. Am. Chem. Soc., 2016, 138(19), 6204–6216 CrossRef CAS PubMed.
- Y. W. Zhang, Y. Cao, C. J. Mao, D. Jiang and W. Zhu, An Iron(III)-Based Metal–Organic Gel-Catalyzed Dual Electrochemiluminescence System for Cytosensing and In Situ Evaluation of the VEGF165 Subtype, Anal. Chem., 2022, 94(9), 4095–4102 CrossRef CAS.
- J. Liu, Y. Cai, R. Song, S. Ding, Z. Lyu, Y.-C. Chang, H. Tian, X. Zhang, D. Du, W. Zhu, Y. Zhou and Y. Lin, Recent progress on single-atom catalysts for CO2 electroreduction, Mater. Today, 2021, 48, 95–114 CrossRef.
- D. Yu, Y. Guan, F. Bai, Z. Du, N. Gao, J. Ren and X. Qu, Metal–Organic Frameworks Harness Cu Chelating and Photooxidation Against Amyloid beta Aggregation in Vivo, Chemistry, 2019, 25(14), 3489–3495 CrossRef PubMed.
- X. Yan, Y. Pan, L. Ji, J. Gu, Y. Hu, Y. Xia, C. Li, X. Zhou, D. Yang and Y. Yu, Multifunctional Metal–Organic Framework as a Versatile Nanoplatform for Abeta Oligomer Imaging and Chemo-Photothermal Treatment in Living Cells, Anal. Chem., 2021, 93(41), 13823–13834 CrossRef.
- L. Zeng, L. Huang, Z. Wang, J. Wei, K. Huang, W. Lin, C. Duan and G. Han, Self-Assembled Metal–Organic Framework Stabilized Organic Cocrystals for Biological Phototherapy, Angew. Chem., Int. Ed., 2021, 60(44), 23569–23573 CrossRef.
- M. Bazi Alahri, R. Arshadizadeh, M. Raeisi, M. Khatami, M. Sadat Sajadi, W. Kamal Abdelbasset, R. Akhmadeev and S. Iravani, Theranostic applications of metal–organic frameworks (MOFs)-based materials in brain disorders: recent advances and challenges, Inorg. Chem. Commun., 2021, 134, 108997 CrossRef.
- M. R. Horn, A. Singh, S. Alomari, S. Goberna-Ferrón, R. Benages-Vilau, N. Chodankar, N. Motta, K. Ostrikov, J. MacLeod, P. Sonar, P. Gomez-Romero and D. Dubal, Polyoxometalates (POMs): from electroactive clusters to energy materials, Energy Environ. Sci., 2021, 14(4), 1652–1700 RSC.
- M. Ma, N. Gao, Y. Sun, X. Du, J. Ren and X. Qu, Redox-Activated Near-Infrared-Responsive Polyoxometalates Used for Photothermal Treatment of Alzheimer's Disease, Adv. Healthcare Mater., 2018, 7(20), e1800320 CrossRef.
- M. Li, C. Xu, J. Ren, E. Wang and X. Qu, Photodegradation of beta-sheet amyloid fibrils associated with Alzheimer's disease by using polyoxometalates as photocatalysts, Chem. Commun., 2013, 49(97), 11394 RSC.
- M. Li, C. Xu, L. Wu, J. Ren, E. Wang and X. Qu, Self-assembled peptide-polyoxometalate hybrid nanospheres: two in one enhances targeted inhibition of amyloid beta-peptide aggregation associated with Alzheimer's disease, Small, 2013, 9(20), 3455–3461 CrossRef CAS.
- N. Gao, H. Sun, K. Dong, J. Ren, T. Duan, C. Xu and X. Qu, Transition-metal-substituted polyoxometalate derivatives as functional anti-amyloid agents for Alzheimer's disease, Nat. Commun., 2014, 5, 3422 CrossRef PubMed.
- N. Gao, K. Dong, A. Zhao, H. Sun, Y. Wang, J. Ren and X. Qu, Polyoxometalate-based nanozyme: design of a multifunctional enzyme for multi-faceted treatment of Alzheimer's disease, Nano Res., 2016, 9, 1079–1090 CrossRef CAS.
- D. Ni, D. Jiang, H. F. Valdovinos, E. B. Ehlerding, B. Yu, T. E. Barnhart, P. Huang and W. Cai, Bioresponsive Polyoxometalate Cluster for Redox-Activated Photoacoustic Imaging-Guided Photothermal Cancer Therapy, Nano Lett., 2017, 17(5), 3282–3289 CrossRef CAS.
- C. Zhang, W. Bu, D. Ni, C. Zuo, C. Cheng, Q. Li, L. Zhang, Z. Wang and J. Shi, A Polyoxometalate Cluster Paradigm with Self-Adaptive Electronic
Structure for Acidity/Reducibility-Specific Photothermal Conversion, J. Am. Chem. Soc., 2016, 138(26), 8156–8164 CrossRef CAS PubMed.
- H. Sun, N. Gao, J. Ren and X. Qu, Polyoxometalate-based Rewritable Paper, Chem. Mater., 2015, 27(22), 7573–7576 CrossRef CAS.
- N. Gao, Z. Liu, H. Zhang, C. Liu, D. Yu, J. Ren and X. Qu, Site-Directed Chemical Modification of Amyloid by Polyoxometalates for Inhibition of Protein Misfolding and Aggregation, Angew. Chem., Int. Ed., 2022, 61(16), e202115336 Search PubMed.
- L. Sercombe, T. Veerati, F. Moheimani, S. Y. Wu, A. K. Sood and S. Hua, Advances and Challenges of Liposome Assisted Drug Delivery, Front. Pharmacol., 2015, 6, 286 Search PubMed.
- E. Moghimipour and S. Handali, Liposomes as Drug Delivery Systems: Properties and Applications, Res. J. Pharm., Biol. Chem. Sci., 2013, 4(1), 169–185 Search PubMed.
- M. Gobbi, F. Re, M. Canovi, M. Beeg, M. Gregori, S. Sesana, S. Sonnino, D. Brogioli, C. Musicanti, P. Gasco, M. Salmona and M. E. Masserini, Lipid-based nanoparticles with high binding affinity for amyloid-beta1-42 peptide, Biomaterials, 2010, 31(25), 6519–6529 CrossRef.
- M. Taylor, S. Moore, S. Mourtas, A. Niarakis, F. Re, C. Zona, B. La Ferla, F. Nicotra, M. Masserini, S. G. Antimisiaris, M. Gregori and D. Allsop, Effect of curcumin-associated and lipid ligand-functionalized nanoliposomes on aggregation of the Alzheimer's Abeta peptide, Nanomedicine, 2011, 7(5), 541–550 CrossRef PubMed.
- L. Bana, S. Minniti, E. Salvati, S. Sesana, V. Zambelli, A. Cagnotto, A. Orlando, E. Cazzaniga, R. Zwart, W. Scheper, M. Masserini and F. Re, Liposomes bi-functionalized with phosphatidic acid and an ApoE-derived peptide affect Abeta aggregation features and cross the blood–brain barrier: implications for therapy of Alzheimer disease, Nanomedicine, 2014, 10(7), 1583–1590 CrossRef CAS PubMed.
- K. Papadia, E. Markoutsa, S. Mourtas, A. D. Giannou, B. La Ferla, F. Nicotra, M. Salmona, P. Klepetsanis, G. T. Stathopoulos and S. G. Antimisiaris, Multifunctional LUV liposomes decorated for BBB and amyloid targeting. A. In vitro proof-of-concept, Eur. J. Pharm. Sci., 2017, 101, 140–148 CrossRef CAS PubMed.
- K. Papadia, A. D. Giannou, E. Markoutsa, C. Bigot, G. Vanhoute, S. Mourtas, A. Van der Linded, G. T. Stathopoulos and S. G. Antimisiaris, Multifunctional LUV liposomes decorated for BBB and amyloid targeting. B. In vivo brain targeting potential in wild-type and APP/PS1 mice, Eur. J. Pharm. Sci., 2017, 102, 180–187 CrossRef CAS PubMed.
- Y.-C. Kuo, I. Y. Chen and R. Rajesh, Use of functionalized liposomes loaded with antioxidants to permeate the blood–brain barrier and inhibit β-amyloid-induced neurodegeneration in the brain, J. Taiwan Inst. Chem. Eng., 2018, 87, 1–14 CrossRef CAS.
- B. Yang, Y. Chen and J. Shi, Mesoporous silica/organosilica nanoparticles: synthesis, biological effect and biomedical application, Mater. Sci. Eng., 2019, 137, 66–105 CrossRef.
- Y. Huang, P. Li, R. Zhao, L. Zhao, J. Liu, S. Peng, X. Fu, X. Wang, R. Luo, R. Wang and Z. Zhang, Silica nanoparticles: biomedical applications and toxicity, Biomed. Pharmacother., 2022, 151, 113053 CrossRef PubMed.
- Z. Zhang, J. Wang, Y. Song, Z. Wang, M. Dong and L. Liu, Disassembly of Alzheimer's amyloid fibrils by functional upconversion nanoparticles under near-infrared light irradiation, Colloids Surf., B, 2019, 181, 341–348 CrossRef PubMed.
- M. Ma, N. Gao, X. Li, Z. Liu, Z. Pi, X. Du, J. Ren and X. Qu, A Biocompatible Second Near-Infrared Nanozyme for Spatiotemporal and Non-Invasive Attenuation of Amyloid Deposition through Scalp and Skull, ACS Nano, 2020, 14(8), 9894–9903 CrossRef PubMed.
- J. Yang, J. Wang, B. Hou, X. Huang, T. Wang, Y. Bao and H. Hao, Porous hydrogen-bonded organic frameworks (HOFs): From design to potential applications, Chem. Eng. J., 2020, 399, 125873 CrossRef.
- H. Zhang, D. Yu, S. Liu, C. Liu, Z. Liu, J. Ren and X. Qu, NIR-II Hydrogen-Bonded Organic Frameworks (HOFs) Used for Target-Specific Amyloid-beta Photooxygenation in an Alzheimer's Disease Model, Angew. Chem., Int. Ed., 2022, 61(2), e202109068 Search PubMed.
- C. Cabaleiro-Lago, F. Quinlan-Pluck, I. Lynch, K. A. Dawson and S. Linse, Dual effect of amino modified polystyrene nanoparticles on amyloid beta protein fibrillation, ACS Chem. Neurosci., 2010, 1(4), 279–287 CrossRef PubMed.
- S. Kuk, B. I. Lee, J. S. Lee and C. B. Park, Rattle-Structured Upconversion Nanoparticles for Near-IR-Induced Suppression of Alzheimer's beta-Amyloid Aggregation, Small, 2017, 13(11), 1603139 CrossRef.
- C. Yan, C. Wang, X. Shao, Y. Teng, P. Chen, X. Hu, P. Guan and H. Wu, Multifunctional Carbon-Dot-Photosensitizer Nanoassemblies for Inhibiting Amyloid Aggregates, Suppressing Microbial Infection, and Overcoming the Blood-Brain Barrier, ACS Appl. Mater. Interfaces, 2022, 14(42), 47432–47444 CrossRef PubMed.
Footnote |
† Xu Shao and Chaoren Yan contributed equally to this work. |
|
This journal is © The Royal Society of Chemistry 2023 |
Click here to see how this site uses Cookies. View our privacy policy here.