Direct multi-elemental analysis of whole blood samples by LA-ICP-MS employing a cryogenic ablation cell†
Received
12th August 2022
, Accepted 24th October 2022
First published on 26th October 2022
Abstract
A simple and rapid method for the multi-elemental analysis of whole blood samples by CLA-ICP-MS (laser ablation inductively coupled plasma mass spectrometry with a cryogenic ablation cell) is described. The whole blood samples were frozen with a designed Peltier-cooled ablation cell, followed by the direct analysis of multiple elements by LA-ICP-MS without any pretreatment. Comparing the precision of time-resolved signals during laser ablation processes between the room temperature (20 °C) and low temperature (−20 °C), the precision was significantly improved, and the RSD was reduced from 14.2 (Mn)–36.4% (Al) to 1.9 (Zn)–6.3% (Al) with six times parallel determination. To improve the sensitivity of the analytical signals, the smaller inner volume ablation cell was used, and the signal intensity was increased by three times compared with the standard commercial ablation cell. CRM Trace Elements Whole Blood L-3 was used as the matrix-match external standard and Rh as an internal standard for the quantitative calibration of whole blood samples. The limits of detection (LODs) for most elements ranged from 0.17 μg L−1 (Mn) to 0.94 μg L−1 (Cr). The accuracy and precision were validated by three strategies, including a standard addition recovery experiment, determination of reference materials, and comparison of different analytical methods. All results showed there were excellent accuracy and precision in the proposed method. A microwell plate was used for high-throughput analysis. The analytical time of each sample was less than 1 min, and the sample consumption was only about 10 μL. With the advantages of high-precision, high-throughput, and small sample consumption, the proposed method was promising for applications in health screenings and disease risk assessments of large populations through blood analysis.
Introduction
The determination of trace elements in human blood is significant for investigations and the predictions of deficiency diseases.1–4 However, routine acid digestion methods could not fully meet the analytical requirements of trace elements in human blood due to time-consuming and large sample consumption.5,6 Therefore, the development of direct blood analysis methods with low sample consumption and high throughput has become a research hotspot.
LA-ICP-MS (Laser Ablation Inductively Coupled Plasma Mass Spectrometry) is a reliable method for the direct analysis of solid samples, which has the advantages of simple preprocessing, low limit of detection, and fast analysis speed and has been applied in several research fields.7–9 Recently, LA was proposed as an introduction system for samples in solution state.10 Günther11 pioneered a method of direct ablation for liquid samples, and the results showed that this method could directly analyze high salt liquid samples and small volume liquid samples due to its small amount of sample introduction. However, the direct ablation of liquid could cause uncontrollable droplet spatter,12 and thus might lead to an unstable amount of sample introduced into ICP, which reduces the precision of data severely, particularly when the concentrations of elements are relatively low. In addition, the liquid surface needs to be covered with a plastic film before analysis,11,12 which increases the difficulty of operation. LA-ICP-MS combined with dry blood spot (DBS) pretreatment is another strategy for the direct analysis of multi-elements in whole blood samples, which has been applied for the direct analysis of a few elements at low concentrations, such as Pb and Cd, Cu, and Co in DBS samples from children, adults, and certified reference materials.13–16 The DBS technology not only has the advantages of convenient sample collection, transportation, and storage but also could convert liquid blood into a solid state by dropping whole blood samples on filter paper/film and drying, which could meet the analysis requirements of LA-ICP-MS.17 In spite of the remarkable advantages of DBS sampling, the inhomogeneous distribution of elements on filter-paper discs caused by the hematocrit and the contamination caused by a filter paper matrix were the main limitations, which could severely influence the accuracy and precision of measured results.18–21 In summary, the conversion of liquid samples into the solid state while ensuring analytical accuracy and precision was the key to the direct analysis of liquid samples by LA-ICP-MS.
Cryogenic ablation cell is a novel and important device in LA-ICP-MS analysis in recent years, which could improve the analytical precision by changing the physical state of the sample or improving the thermal effect during the process of interaction between the laser and material.22,23 Cryogenic ablation cell has been successfully applied to the analysis of fresh biological soft tissues24–26 and fluid inclusions,27 and proved to be a promising strategy. Theoretically, the cryogenic ablation cell could be used for the direct analysis of liquid samples because it could quickly and effectively convert liquid samples into a solid state, which has proved to be an effective strategy to avoid liquid splashing during the laser ablation process in the analysis of fluid inclusions.27
In this study, we developed a high-precision method for the direct analysis of multi-elements in whole blood samples based on CLA-ICP-MS (laser ablation inductively coupled plasma mass spectrometry with a cryogenic ablation cell). A designed cryogenic ablation cell was applied to improve the analytical precision, and a designed microwell plate was used to load the whole blood samples to improve the analysis throughput. Fifty-seven whole blood samples were analyzed by the proposed method.
Experimental section
Instrument
Experiments were carried out using a Coherent GeoLas 193 nm ArF excimer laser ablation (LA) system (MicroLas Göttingen, Germany) in combination with an Agilent 7700× ICP-MS (Agilent Technologies, Tokyo, Japan). Table 1 details the optimized parameters of LA-ICP-MS. LA-ICP-MS was warmed up for about 30 min before operation and optimization. Helium was used as a carrier gas to transport the ablated aerosol from the ablation cell to the ICP-MS, and argon as the makeup gas for aerosol transport. The optimization was conducted by ablating a glass NIST SRM 610 to obtain a maximum signal intensity for 7Li, 89Y, and 238U while keeping the ThO/Th ratio <0.3% and the U/Th ratio close to 1. 7Li, 25Mg, 27Al, 53Cr, 55Mn, 63Cu, 66Zn, 137Ba, and 208Pb in the whole blood samples were determined based on clinical analysis.
Table 1 The operating conditions of LA-ICP-MS
ICP-MS |
Agilent technology, 7700× |
RF power (W) |
1550 |
Reflected power (W) |
8 |
Sampling depth (mm) |
7.5 |
Plasma gas flow (L min−1) |
15 |
Auxiliary gas flow (L min−1) |
1.0 |
Carrier gas flow (L min−1) |
1.0 |
Mode |
No gas |
Isotopes |
7Li, 25Mg, 27Al, 53Cr, 55Mn, 63Cu, 66Zn, 137Ba, 208Pb |
Laser system |
Coherent lambda physik GmbH, GeoLas HD |
Energy density (J cm−2) |
12 |
Spot diameter (μm) |
160 |
The speed of scanning (μm s−1) |
10 |
Frequency (Hz) |
12 |
Carrier gas (He) (L min−1) |
0.4 |
Ablation mode |
Line scan |
The temperature of cryogenic cell |
−20 °C |
Reagents and samples
A multi-element standard solution of 10 μg mL−1 and rhodium (Rh) standard solution of 1000 μg mL−1 were purchased from the National Center for Analysis and Testing of Steel Materials (Beijing, China). The human blood certified reference materials (CRM) were purchased from Seronorm™ (Trace Elements Whole Blood L-2, LOT 2011934, Trace Elements Whole Blood L-3, LOT 2011935). Superior-grade bovine serum albumin powder was purchased from Bomei Biotechnology Co., Ltd. (Hefei, China). Guaranteed HNO3 was purchased from Alfa Aesar Ltd. (Tianjin, China) and was further distilled twice using a homemade PTFE sub-boiling distillation system in an ultra-clean laboratory. Fifty-seven whole blood samples were taken from the hospital of the China University of Geosciences (Wuhan, China). All blood samples were stored in dark at 4 °C before use. Ultrapure water (18.2 MΩ) was obtained from a Milli-Q water purification system (Millipore, Bedford, MA, USA).
Direct elemental analysis of whole blood samples by CLA-ICP-MS
In this study, the CRM Trace Elements Whole Blood L-3 was used as the external standard, and Rh was selected as the internal standard for quantitative analysis. 0.2 μL Rh standard solution at a concentration of 100 μg mL−1 and 9.8 μL blood sample were injected into the circular holes of a microwell plate with a micro-volume sampler, and then the microwell plate was put into a designed cryogenic ablation cell (Fig. S1†). The microplate has 64 holes with a diameter of 1.25 mm and a depth of 2.5 mm and was cleaned with 2% nitric acid to ensure no residual contamination after use. The whole blood samples were frozen in the cryogenic ablation cell at −20 °C for about 5 min and analyzed by LA-ICP-MS. When the blood samples were analyzed, the laser beam was focused on the surface of solid blood before data acquisition, and the aerosol generated by LA was transported with a carrier gas into the ICP-MS. Each whole blood sample was analyzed 3 times with line scan mode, and each analysis process included 10 s of background signal generated by the carrier gas and 40 s of data acquisition, and a washing time of 10 s was included between successive ablations to minimize or avoid memory effects. The external standard was analyzed at intervals of ten samples to correct the drift of the instrument state.
Results and discussion
Comparison of precision of signal at room temperature and low temperature
To suppress the droplet splashing and improve the analytical precision, the designed cryogenic ablation cell was used in the analysis of whole blood samples. In this experiment, the CRM (Trace Elements Whole Blood L-2) sample was analyzed 6 times with line scan mode at low (−20 °C) and room temperature (20 °C), and the precision of the signal obtained at these two temperatures was compared. Fig. 1(a) shows the RSD of the 6 times parallel determination. At room temperature, the RSD of the signal of elements ranged from 14.2% (Mn) to 36.4% (Al). The results were probably due to the splash and deposition of droplets caused by laser pulses, which meant an unstable quantity of sampling and led to poor reproducibility of measured results. When the sample was ablated at a low temperature, the RSD of elemental signals was less than 6.3%, which indicated that a stable and reliable ablation could be realized during the laser ablation of whole blood samples. Therefore, using the cryogenic ablation cell could provide excellent reproducibility, which could ensure the quality of measured data. Fig. 1(b) presented the time-resolved signals of a blood sample at room temperature mode. There were obvious fluctuations and some spikes in the signal at room temperature, and the RSD of the signal of all elements was more than 18.2%. On the contrary, as shown in Fig. 1(c), the signal in the low temperature mode had ideal stability, and the RSD of elemental signals is less than 8.3%. Presumably, there was a stable and controllable ablation behavior during the analysis of solid blood samples, and the materials could be stably ablated by the laser pulse and introduced to ICP by the carrier gas. With the reduction of temperature of the cryogenic ablation cell, the signal intensity of the elements decreases by about 20.42% (Pb) – 34.18% (Zn), probably because the density of the frozen blood sample increased as the temperature decreased, which meant that relatively less material was ablated out under the same laser conditions. In summary, the cryogenic ablation cell could freeze the whole blood samples effectively, thus improving the precision significantly, which was the basis of guaranteeing data quality.
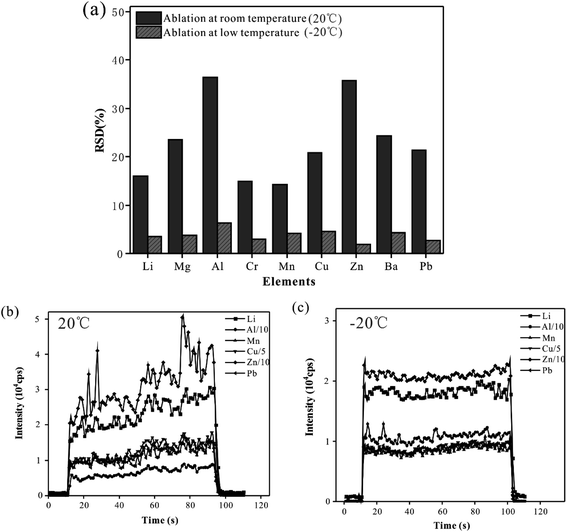 |
| Fig. 1 (a) The precision of six times parallel determination of CRM whole blood at room and low temperature with line scan mode. The time-resolved signals of elements in the analysis of CRM at room temperature (20 °C) (b) and low temperature (−20 °C) (c). | |
Effects of ablation cell temperature
To provide good precision and accuracy for this method, the effect of the cryogenic ablation cell on the stability and sensitivity of elemental signals was investigated. In this experiment, the CRM was analyzed three times with line scan mode at different temperatures (20 °C to −30 °C). As shown in Fig. 2, when the temperature of the cryogenic ablation cell was 20 °C, the RSD of all elements was more than 20%, and when the temperatures of the cryogenic ablation cell ranged from −10 to −30 °C, the RSD of all elements was less than 8%. On the other hand, the signal intensity of elements obtained at −10 to −30 °C decreased by about 20% compared with that at 20 °C. The results showed that low temperatures could effectively improve the analytical precision. In addition, the cryogenic ablation cell could provide an excellent refrigeration performance at different temperatures, which could freeze the blood into solid quickly, thus ensuring the uniform distribution of elements in the frozen blood. Based on these results, a freezing temperature of −20 °C was selected for further experiments.
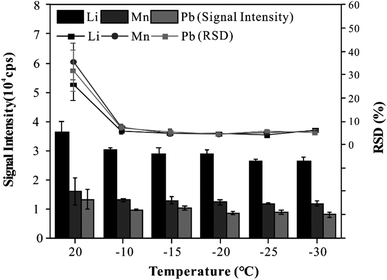 |
| Fig. 2 Effect of cryogenic ablation cell temperature on intensity and precision of signal. | |
Matrix effects
The difference in the matrix between the external standard and the sample would directly affect the accuracy and precision of the method in LA-ICP-MS analysis. To enable the quality of data, the matrix effect was studied. In this experiment, the matrix effects were investigated by analyzing three standard solutions with different matrices (4% BSA solution, water solution, and whole blood CRM L-2). The elemental signals were normalized to that of the whole blood CRM. Fig. 3 shows that there are obvious differences between the whole blood matrix, 4% BSA matrix, and water matrix. The signals of Al, Cr, Zn, and Pb produced by the water sample were more than 20% different from that produced by the CRM whole blood. In addition, there was a difference of more than 20% for signals of Mg, Zn, and Pb between 4% BSA matrix and CRM whole blood. This was probably because the blood matrix was complex and quite different from the water or 4% BSA matrix in physical properties and chemical composition, so the three solutions would have different behavior in laser ablation, aerosol transport, and ionization processes. To enable the accuracy of this method, it was necessary to use the matrix-matching external standard for quantitative calibration of whole blood samples.
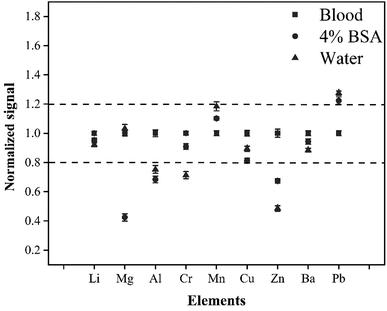 |
| Fig. 3 The signal generated by three standard solutions with different matrices (CRM whole blood, 4% BSA, water). The elemental signals generated by the three solutions were normalized to that of the CRM whole blood sample. | |
Effect of the volume of added internal standard elements on the signal intensity and precision
To correct the difference in the absolute amount of ablating material during the analysis of LA-ICP-MS, it is necessary to add a reliable internal standard element into the whole blood samples.28 Many scholars choose element C as an internal standard in blood analysis by LA-ICP-MS.17,29 However, it is not an ideal choice due to the high content of C in the blood samples. In this experiment, Rh (the concentration of 100 μg mL−1) was selected as the internal standard and was mixed with CRM Trace Elements Whole Blood L-2 in the hole of the microwell plate. Predictably, the volume of the added internal standard solution is an important factor affecting the uniformity of element distribution in frozen blood samples. Therefore, the effects of internal standard addition volume on the precision and signal intensity were investigated. The total volume of liquid added to the hole of the microwell plate was set at 10 μL, the volume of the internal standard solution gradually increased from 0.1 μL to 0.5 μL, and the volume of the blood sample gradually decreased from 9.9 μL to 9.5 μL. All samples were analyzed by CLA-ICP-MS with line scan mode, and elements Li, Mn, Rh, and Pb representing different mass ranges were monitored. As shown in Fig. 4, with the increasing volume of the added internal standard, there was no significant variation in the RSD of the elemental signals, indicating that the blood and the internal standard solution could be mixed uniformly. As can be seen, the signal intensity increased with the increase of the internal standard volume addition. When the volume of the added internal standard was greater than 0.2 μL, the signal intensity of Rh was more than 120
000 cps, and a small RSD (4.62%) could be obtained, which is conducive to accurate quantification. In this experiment, 0.2 μL was selected as the optimal internal standard addition volume.
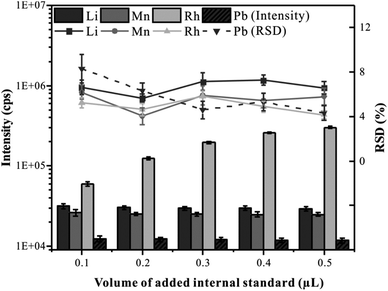 |
| Fig. 4 Effect of volume of the added internal standard on signal intensity and precision. | |
Effect of ablation cell volume
To improve the problem that the elemental signal intensity of whole blood samples decreases at a low temperature, the strategy of reducing the effective volume of the ablation cell was used. Recently, a series of ablation cells for efficient aerosol transport were reported.30,31 The reduction of the volume of the ablation cell is still a simple and effective strategy to enhance the transport efficiency of aerosols. In the experiment, we reduced the inner volume of this circular cryogenic ablation cell to just 12.6 cm3, which is less than one-third of the volume of the circular commercial ablation cell (internal volume of 40 cm3). Glass reference material NIST610 was analyzed using the present cryogenic ablation cell and the circular commercial standard ablation cell respectively with line scan mode, and Li, V, Mn, Cu, Pb, and U were monitored as representative elements. As shown in Fig. 5, a relatively high intensity could be observed with this cryogenic ablation cell, about three times of that with the commercial cell. This result was consistent with the conclusion of a previous study. Bleiner32 believed that aerosol extraction could be regarded as the collision probability of aerosol particles with the air outlet. The small inner volume, small diameter of the inlet nozzle, and large diameter of the outlet nozzle mean high aerosol density, fast flight speed of aerosol, and high collision probability between the outlet nozzle and aerosol particles, which could effectively enhance the signal intensity, thereby improving the accuracy, precision, and limit of detection of the method.
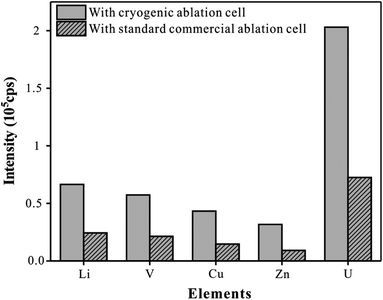 |
| Fig. 5 Comparison of signals obtained from NIST610 analysis using the proposed cryogenic ablation cell and commercial standard ablation cell. The internal volume of the standard commercial ablation cell is about 40 cm3. | |
Analytical performances
Limits of detection
In this experiment, a gas blank was repeatedly measured 11 times, and 3 times the standard deviation of 11 parallel signals of the corresponding analyte was used to calculate LODs according to the study of Longerich et al.33 The limits of detection (LODs) ranged from 0.17 μg L−1 (Mn) to 0.94 μg L−1 (Cr) except for Mg (21.39 μg L−1).
The validation of accuracy and precision
The accuracy and precision of the proposed method were evaluated by three strategies, including a standard addition recovery experiment, determination of reference materials, and comparison of different analytical methods. These results demonstrate that the proposed method has excellent accuracy and precision for most elements.
Standard addition recovery experiment.
A real whole blood sample was added to the standard solution with different concentrations and then injected into the holes of the microwell plate and analyzed directly by CLA-ICP-MS with line scan mode. The CRM whole blood L-3 was used as an external standard. Table 2 shows the analytical results and the recoveries of all elements ranging from 97% (Ba) to 106% (Al).
Table 2 The data for standard addition recovery (N = 3)
Elements |
Concentration in sample/μg L−1 |
Added concentration/μg L−1 |
Found concentration/μg L−1 |
Recovery/% |
μg mL−1.
|
Li |
108.4 |
100 |
209.1 |
100.7 |
Mg |
13.4a |
10a |
23.2a |
98.0 |
Al |
88.7 |
100 |
194.7 |
106.0 |
Cr |
81.2 |
100 |
183.1 |
101.9 |
Mn |
46.2 |
50 |
98.3 |
104.2 |
Cu |
7.4a |
10a |
17.8a |
104.0 |
Zn |
17.1a |
20a |
37.6a |
102.5 |
Ba |
19.5 |
20 |
38.9 |
97.0 |
Pb |
82.7 |
100 |
187.1 |
104.4 |
Determination of reference materials.
The CRM Trace Elements Whole Blood L-3 was used as the matrix-match external standard for quantification of the CRM Trace Elements Whole Blood L-2. The CRM samples were analyzed directly by CLA-ICP-MS with line scan mode. Table 3 shows that the determined values are in good agreement with certified values, and the relative errors for all elements range from 1.29% (Al) to 7.33% (Li).
Table 3 Analytical results for the determination of multiple elements in CRM whole blood L-2 (N = 3)
Elements |
Certified value (μg L−1) |
Found value (μg L−1) |
Relative error (%) |
μg mL−1.
|
Li |
7.5 ± 1.5 |
7.0 ± 0.2 |
7.3 |
Mga |
26.7 ± 5.4 |
25.2 ± 0.7 |
5.6 |
Al |
59 ± 12 |
58.2 ± 1.4 |
1.3 |
Cr |
9.8 ± 2 |
10.3 ± 0.1 |
5.3 |
Mn |
22.5 ± 1.8 |
23.1 ± 1.0 |
2.4 |
Cua |
0.92 ± 0.08 |
0.88 ± 0.03 |
3.8 |
Zna |
6.2 ± 1.3 |
6.3 ± 0.2 |
1.6 |
Ba |
65 |
68.9 ± 2.2 |
6.0 |
Pb |
295 ± 30 |
279.0 ± 7.8 |
5.4 |
Comparision with different analytical methods.
The proposed method and routine SN-ICP-MS were used to determine multi-elements in fifty-seven whole blood samples. The analytical results obtained with the two methods are compared and confirmed to be consistent (Table S1†). For the CLA-ICP-MS analysis, 57 whole blood samples were determined within 1 h, and each sample consumed less than 1 min, which allows high-throughput detection of blood samples, particularly suitable for large-scale population health investigations. There is no complex and time-consuming pretreatment in this process, thus avoiding the risk of sample contamination and improving the analytical efficiency.
Conclusions
A novel high-precision and high-throughput method based on the CLA-ICP-MS system for the direct determination of major and trace elements in whole blood samples was developed. This method used a designed cryogenic ablation cell to avoid the droplet splash during the ablation process by freezing liquid whole blood samples into the solid state, which improved the precision significantly. The use of a microwell plate allowed high throughput analysis of blood samples, which is expected to meet large population screening for diseases. Further, by combining CLA-ICP-MS with the automatic location raster mode of the fs-laser ablation system, high-throughput automatic analysis of whole blood samples could be achieved.
Conflicts of interest
There are no conflicts to declare.
Acknowledgements
This study was financially supported by the National Natural Science Foundation of China (No. 41873072) the National Key R&D Program of China (No. 2021YFC2903003). All the whole blood samples were provided by the hospital of China University of Geosciences (Wuhan, China), and informed consent was obtained from human participants of this study.
References
- S. Meyer, M. Markova, G. Pohl, T. A. Marschall, O. Pivovarova, A. F. H. Pfeiffer and T. Schwerdtle, J. Trace Elem. Med. Biol., 2018, 49, 157–163 CrossRef CAS
.
- A. Stojsavljević, J. Trifković, Z. Rasić-Milutinović, D. Jovanović and D. Manojlović, J. Trace Elem. Med. Biol., 2018, 48, 134–140 CrossRef
.
- J. Trifunovic, D. Jovanovic, J. Mutic, Z. Rasic-Milutinovic and G. Bogdanovic, Exp. Clin. Endocrinol. Diabetes Off. J. Ger. Soc. Endocrinol. Ger. Diabetes Assoc., 2017, 125, 79–85 Search PubMed
.
- N. Laur, R. Kinscherf, K. Pomytkin, L. Kaiser, O. Knes and H.-P. Deigner, PLoS One, 2020, 15, e0233357 CrossRef CAS
.
- A. Bazzi, J. O. Nriagu, M. C. Inhorn and A. M. Linder, J. Environ. Monit., 2005, 7, 1251–1254 RSC
.
- A. Bazzi, J. O. Nriagu and A. M. Linder, J. Environ. Monit., 2008, 10, 1226–1232 RSC
.
- Y. Ke, J. Zhou, X. Yi, Y. Sun, J. Shao, S. You, W. Wang, Y. Tang and C. Tu, J. Anal. At. Spectrom., 2020, 35, 886–895 RSC
.
- J. S. Becker, A. Matusch and B. Wu, Anal. Chim. Acta, 2014, 835, 1–18 CrossRef CAS
.
- S. Durrant and N. Ward, J. Anal. At. Spectrom., 2005, 20, 821–829 RSC
.
- X. Liao, Z. Hu, T. Luo, W. Zhang, Y. Liu, K. Zong, L. Zhou and J. Zhang, J. Anal. At. Spectrom., 2019, 34, 1126–1134 RSC
.
- D. Günther, R. Frischknecht, H.-J. Müschenborn and C. A. Heinrich, Fresenius. J. Anal. Chem., 1997, 359, 390–393 CrossRef
.
- X. Liao, T. Luo, S. Zhang, W. Zhang, K. Zong, Y. Liu and Z. Hu, J. Anal. At. Spectrom., 2020, 35, 1071–1079 RSC
.
- J. V. Cizdziel, Anal. Bioanal. Chem., 2007, 388, 603–611 CrossRef CAS PubMed
.
- H. F. Hsieh, W. S. Chang, Y. K. Hsieh and C. F. Wang, Talanta, 2009, 79, 183–188 CrossRef CAS PubMed
.
- P. Foltynová, V. Kanický and J. Preisler, Anal. Chem., 2012, 84, 2268 CrossRef PubMed
.
- M. Aramendía, L. Rello, S. Bérail, A. Donard, C. Pécheyran and M. Resano, J. Anal. At. Spectrom., 2015, 30, 525 RSC
.
- J. Moreda-Piñeiro, A. M. Cantarero-Roldán, A. Moreda-Piñeiro, J. Á. Cocho and P. Bermejo Barrera, J. Anal. At. Spectrom., 2017, 32, 1500–1507 RSC
.
- S. Velghe, L. Delahaye and C. P. Stove, J. Pharm. Biomed. Anal., 2019, 163, 188–196 CrossRef CAS PubMed
.
- J. D. Freeman, L. M. Rosman, J. D. Ratcliff, P. T. Strickland, D. R. Graham and E. K. Silbergeld, Clin. Chem., 2018, 64, 656–679 CrossRef CAS PubMed
.
- M. Resano, M. A. Belarra, E. García-Ruiz, M. Aramendía and L. Rello, TrAC, Trends Anal. Chem., 2018, 99, 75–87 CrossRef CAS
.
- R. J. Meesters, J. Zhang, N. A. van
Huizen, G. P. Hooff, R. A. Gruters and T. M. Luider, Bioanalysis, 2012, 4, 2027–2035 CrossRef CAS
.
- M. Albrecht, I. T. Derrey, I. Horn, S. Schuth and S. Weyer, J. Anal. At. Spectrom., 2014, 29, 1034–1041 RSC
.
- I. Konz, B. Fernández, M. L. Fernández, R. Pereiro and A. Sanz-Medel, Anal. Chim. Acta, 2014, 809, 88–96 CrossRef CAS PubMed
.
- J. Feldmann, A. Kindness and P. Ek, J. Anal. At. Spectrom., 2002, 17, 813–818 RSC
.
- I. Konz, B. Fernández, M. L. Fernández, R. Pereiro, H. González-Iglesias, M. Coca-Prados and A. Sanz-Medel, Anal. Bioanal. Chem., 2014, 406, 2343–2348 CrossRef CAS PubMed
.
- J. S. Hamilton, E. L. Gorishek, P. M. Mach, D. Sturtevant, M. L. Ladage, N. Suzuki, P. A. Padilla, R. Mittler, K. D. Chapman and G. F. Verbeck, J. Anal. At. Spectrom., 2016, 31, 1030–1033 RSC
.
- W. Jian, M. Albrecht, B. Lehmann, J. Mao, I. Horn, Y. Li, H. Ye, Z. Li, G. Fang and Y. Xue, Geofluids, 2018, 2018, 1–17 CrossRef
.
- Y. Liu, Z. Hu, S. Gao, D. Günther, J. Xu, C. Gao and H. Chen, Chem. Geol., 2008, 257, 34–43 CrossRef CAS
.
- M. P. Chantada-Vázquez, J. Moreda-Piñeiro, A. Cantarero-Roldán, P. Bermejo-Barrera and A. Moreda-Piñeiro, Talanta, 2018, 186, 169–175 CrossRef
.
- H. A. O. Wang, D. Grolimund, C. Giesen, C. N. Borca, J. R. H. Shaw-Stewart, B. Bodenmiller and D. Günther, Anal. Chem., 2013, 85, 10107–10116 CrossRef CAS PubMed
.
- S. J. M. Van Malderen, J. T. van Elteren and F. Vanhaecke, J. Anal. At. Spectrom., 2015, 30, 119–125 RSC
.
- D. Bleiner and A. Bogaerts, Spectrochim. Acta, Part B, 2007, 62, 155–168 CrossRef
.
- H. P. Longerich, S. E. Jackson and D. Gunther, J. Anal. At. Spectrom., 1996, 11, 899–904 RSC
.
|
This journal is © The Royal Society of Chemistry 2023 |
Click here to see how this site uses Cookies. View our privacy policy here.